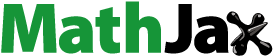
ABSTRACT
Synchronized indoor and outdoor sampling campaigns of PM10 were performed in two research laboratories and a mechanical workshop in Mexico City, during the dry cold season. The goals were to measure indoor and outdoor gravimetric mass and elemental concentrations, recognize the origin of particles, penetration toward the indoor environments, and assess inhalation, ingestion, and dermal absorption risks to human health. Concentrations of 18 elements were measured with X-ray Fluorescence; selected samples were studied with Scanning Electron Microscopy. Mass concentrations were higher outdoors than indoors and elemental concentrations were similar. Enrichment factors identified several geogenic elements. Cluster Analysis recognized common sources of elements and penetration of outdoor particles to indoor environments. Particles emitted indoors could be identified. Exposure to Mn, Fe, Ni, Cu, Zn, and Pb particles produced low health risks; Cr may only cause an ingestion risk. Only outdoor Mn and Ni Hazard Quotients are higher outdoors than indoors.
1. Introduction
Professional tasks carried out in indoor environments may expose workers to different kinds of pollutants, depending on the type of activity. Therefore, many investigations have been carried out about the air quality in classrooms [Citation1,Citation2], dental premises [Citation3], offices [Citation4–7], libraries [Citation8], restaurants [Citation9], and industrial facilities [Citation10], among others. Particles suspended in the atmosphere (particulate matter, or PM) are critical pollutants, in particular, those with an aerodynamic diameter smaller than 10 µm (PM10) or 2.5 µm (PM2.5), because they can penetrate either the upper respiratory tract or the lungs, respectively [Citation11]. Furthermore, these airborne particles may also cause health damage through ingestion and dermal absorption [Citation12]; these processes may be more remarkable for PM10 than for PM2.5.
Numerous studies have demonstrated that the presence of certain elements in PM10 may produce effects on human health. For instance, Negral et al. [Citation13] studied the relationship among meteorological variables, metal concentrations in PM10, and health risks in northern Spain; they found that most elements had an anthropogenic origin and both cancer and non-cancer risks were not too high. Embiale and collaborators have published investigations about concentrations of volatile compounds and trace elements in Ethiopian living rooms [Citation14] and elemental composition of PM10 during traditional Ethiopian food cooking [Citation15]; the associated health risks for lifetime exposure to PM10 and the constituent elements were low, but the risks due to daily exposure to wood-burning stoves emissions were considerable. Seasonal changes in concentrations of 18 elements and cancer risk were analyzed by Koukoulakis et al. [Citation16], in samples collected in an industrialized area near Athens, Greece; it was determined that the majority of the elements observed were produced by the nearby industries and that there was a significant cancer risk by inhalation. The contents of lanthanoids (rare earth elements) and other metals have been determined in the particles collected in an urban area in northern India [Citation17] and Mexico City [Citation18]; both studies showed that the concentrations were below allowed limits, but no risk assessments were given. Roy et al. [Citation19] measured the concentrations of As, Be, Cd, Cr, Cu, Fe, Mn, Ni, and Pb in samples collected in Seoul, Korea, and presented a health risk assessment, which was considered as significant mostly by ingestion, and the authors found that the elements were anthropogenic. Zhang et al. [Citation20] analyzed PM10 and PM2.5 from roadsides in Tianjin, China, with the health risk estimation; the authors determined a high risk for non-cancerous elements via inhalation. In a similar study, Cerón et al. [Citation21] determined the outdoor concentrations of Cu, Co, Zn, Cd, Fe, Mg, and Mn, in PM10 from León, central Mexico; it was found that there is a substantial cancer risk for the inhabitants. Reynoso-Cruces and coworkers [Citation22] measured concentrations of 18 elements in indoor and outdoor environments from households in Mexico City and assessed potential health risks; although the measured concentrations never surpassed the official standards, there is a potential risk for children through Cr ingestion. These works stress the need to measure the elemental concentrations in the airborne particles to assess potential health risks.
There are many published works about indoor air quality in teaching laboratories at universities [Citation23–26]; an example is the paper by Perrino et al. [Citation27], studying the chemical composition of PM10 in several university laboratories at the University of Rome; it was possible to identify external and local emitting sources, and important differences in the concentrations of several chemical species were found between the indoor and outdoor environments. However, fewer studies regarding the presence and composition of PM10 in research laboratories can be found. For instance, Fuehne et al. [Citation28] published extensive air quality reports from Los Alamos National Laboratory, with different conclusions, depending on the analyzed pollutant; Žitnik et al. [Citation29] conducted a study about time-resolved measurements in a chemistry laboratory for nanoparticle materials, and demonstrated that measuring the elemental concentrations with a one-hour resolution permitted to identify the emitting sources, also through multivariate analytical methods; the air quality in a research laboratory (which includes offices, student rooms and a chemical area for experiments) was investigated by Mishra et al. [Citation30], finding that the concentrations of airborne particles were higher indoors than outdoors; Reynoso-Cruces et al. [Citation31] analyzed the elemental composition of PM10 and PM2.5 in a particle accelerator laboratory, incorporating a simple risk assessment; the contributions by the accelerator and different associated devices were determined and cancer risks were not relevant. Other facilities related to research laboratories are specialized mechanical workshops, where many components for those laboratories are built. In this regard, several studies are available, such as the works by Iavicoli et al. [Citation32], where PM in a mechanical engineering factory was analyzed to identify the origin of the observed particles, although no risk assessments were given; Demou et al. [Citation33] studied the inhalation exposure to PM1 in a machine shop, taking the PM2.5 and PM10 values as a reference; Isaxon et al. [Citation34] characterized nanoparticles emitted in three Swedish welding workshops, determining the source of the particles using several analytical methods; and Zhou et al. [Citation35] in a printed circuit board workshop, who measured the concentrations of several metals in PM10 and PM2.5, concluding that only Cr may pose a carcinogenic risk. In many of these works, the need for the characterization of indoor pollutants, especially PM, is emphasized, including risk assessments.
Therefore, in the present work, results of elemental analyses of PM10, obtained with X-ray fluorescence (XRF) and Scanning Electron Microscopy (SEM) plus qualitative Electron Probe Microanalysis (EPMA), of samples that were collected in two research laboratories and a machine shop are described, to determine the elemental concentrations, the possible origin of the airborne particles, as well as a risk assessment for elements that may be hazardous to human health. This study is expected to increase the knowledge about the composition of PM10 present in working environments in Mexico, a subject that has not been widely studied.
2. Materials and methods
2.1. Sampling sites and procedures
Two research laboratories and the mechanical workshop at the Instituto de Física, Universidad Nacional Autónoma de México (IFUNAM), were selected as sampling sites; all of them are situated in different buildings. The first site was the Aerosols laboratory (located at the Colisur building), mainly dedicated to analyzing aerosol, soil, and food samples using XRF. The second location was the 5.5 MV Van de Graaff laboratory, where Nuclear Physics experiments and ion beam analytical techniques are carried out. The third site was the mechanical workshop, connected to the electronics and mechatronics laboratory. shows the location of the Instituto de Física, at the main UNAM campus, southwest of the Mexico City Metropolitan Area (MCMA), and that of the studied buildings.
Figure 1. Map of the buildings at the Instituto de Física, where the indoor environments are located. The shaded area represents the urbanized surface in the metropolitan area of Mexico City.

Figures S1, S2, and S3 of the supplementary material display more detailed sketches of the laboratories and the workshop, respectively, with the location of the equipment for the indoor sampling. Each of these spaces is usually ventilated through windows or doors. All the walls are built with bricks or concrete, the floor in the Aerosols laboratory is covered with tiles, and the other two studied areas have concrete floors. The outdoor sampling was carried out on the roof of the Colisur building, 7 m above the ground level, as marked in . The indoor sampler was placed 1.8 m above the floor level at the three sites, to avoid possible physical contact of the personnel with the device.
Regarding the activities in the Aerosols laboratory, two persons operate the equipment from 7:30 to 14:00 local standard time (LST). As for the Van de Graaff laboratory, four to five people work from 7:30 until 19:00 LST. Finally, around 25 people work in the mechanical workshop daily, starting at 7:00 LST and leaving at 18:00 LST. Also, routine cleaning is done at every site around 7:00 LST before the personnel starts operating the equipment.
Vacuum pumps regularly operate inside the laboratories, together with the spectrometers or the accelerator, including the necessary electronic devices. In this case, the Van de Graaff accelerator uses a mixture of N2 and CO2 as dielectric gas instead of SF6 employed in the Instituto de Física Tandetron accelerator, as reported in a previous paper [Citation31]. In contrast, drills, lathes, polishing machines, welding equipment, and cutters are manipulated in the mechanical workshop.
The PM10 samples were collected through portable MiniVol TAS 5.0 air samplers (Airmetrics, U.S.A), operating at an air flux of 5 L min−1, depositing the particles on 47 mm polycarbonate filters, with 0.4 µm pore size (Whatman, Florham Park, NJ, U.S.A). The air flux in the samplers was calibrated with a Dwyer series 475 Mark III digital manometer (Dwyer Instruments, Michigan City, IN, U.S.A) and compared to a fluxmeter [Citation36].
Due to access limitations on weekends, the PM10 samples were collected from Monday to Friday in each place, starting at 10:35 h until 10:30 h of the next day, indoors and outdoors simultaneously. The campaign ran at the Aerosols laboratory from 18 October 2021, until 6 December 2021; at the Van de Graaff laboratory, from 8 February 2022, to 24 March 2022; at the workshop, starting 8 November 2022, and ending 15 December 2022. All these periods correspond to the dry cold season of the MAMC [Citation37]. A total of 50 valid samples were collected at the Aerosols laboratory (25 indoors and 25 outdoors), 47 samples at the Van de Graaff laboratory (23 indoors and 24 outdoors), and 42 filters at the mechanical workshop (21 indoors and 21 outdoors).
2.2. Analyses of samples
As explained in previous works [Citation31,Citation38], the environmental conditions during gravimetric analyses were 35% - 40% RH and a temperature range of 19°C to 22°C. Field blanks and exposed filters were kept in Petri dishes in a desiccator cabinet for 48 h before pre- and post-weighing (three times each) with an Ohaus 200GD electrobalance (0.01 mg resolution). Outdoor mass concentrations were additionally obtained from the Automatic Atmospheric Monitoring Network (RAMA) [Citation39], operated continuously by the local government, with data from the Benito Juárez station (BJU), located 5.5 km north of the sampling site. The measurements of pollutants and meteorological variables from this network are officially taken as a reference [Citation37]. For quality assurance purposes, one field blank filter was exposed for 24 h in each site. The gravimetric mass and elemental concentration analyses were corrected according to the field blanks.
XRF was used for the elemental analysis of the filters, utilizing the spectrometer designed and constructed at the Instituto de Física, Universidad Nacional Autónoma de México, operating at the Aerosols laboratory [Citation40,Citation41]. The device is based on an X-ray tube with Rh anode (Oxford Instruments, Mountain View, CA, U.S.A), functioning at 50 keV and 750 µA, and an Amptek 123-FastSDD spectrometer, with a resolution of 120 eV at 5.9 keV. The filters were irradiated at a high vacuum (10−4 Pa). The irradiation time was 900 s, and the collected spectra were analyzed through the Quantitative X-ray Analysis System (QXAS) [Citation42]. Experimental uncertainties for each elemental concentration (between 6% and 20%) were evaluated according to the procedures explained by Espinosa et al. [Citation43] and Reynoso-Cruces et al. [Citation31].
The detection system efficiency was determined by irradiating a set of thin film standards (MicroMatter Co., Vancouver, Canada), collecting three spectra per standard, for 300 s, under the same conditions as those used for the exposed filters. Furthermore, an accuracy verification was carried out with the analysis of the NIST standard reference material 2783, Air Particulate on Filter Media. The results of this verification, determination of limits of detection, as well as the quality control – quality assurance procedures have been deeply explained elsewhere [Citation38,Citation41,Citation44].
The Stata® 15 software (StataCorp, College Station, TX, U.S.A) was employed to evaluate basic statistics and Analysis of Variance (ANOVA). Cluster Analysis (CA) was also applied to each data set, with the purpose of finding associations among the elements and their possible emitting sources; Stata® 15 was also used for these calculations. Ward’s method of amalgamation and Pearson’s correlation coefficients were used to build the corresponding dendrograms with CA.
Additionally, selected samples were analyzed with SEM to explain several results obtained with XRF. Images were acquired for various areas of the samples, together with a qualitative X-ray analysis with EPMA. These studies were done at the Central Microscopy Laboratory (LCM), at IFUNAM, with a JEOL 7800F scanning electron microscope. Operating voltages ranged between 15 kV and 20 kV. The samples were covered with a thin Au layer to prevent the electrostatic charging of the particles.
2.3. Health risk assessment
As an indicator of the possible health risk produced by elements detected in indoor PM10, the Hazard Quotient (HQ) is computed for men and women. The US EPA [Citation45] gives relevant criteria to explain the evaluation results, asserting that if HQ < 1, there is not a high probability for non-cancerous, and if HQ ≥1, adverse health effects might occur. Moreover, if HQ > 10, there exists a high chronic risk to human health. Thus, the US EPA fixes tolerable risk limits between 1 × 10−4 and 1 × 10−6 [Citation45].
The expressions to determine HQ are [Citation12]:
In these equations, C means the elemental concentration (mg m−3); ED the exposure duration (years); daily doses are represented by Dinh, Ding, and Dder for inhalation, ingestion, and dermal absorption (mg kg−1 d−1), respectively; InhR is the inhalation rate (m3 d−1); EF denotes the exposure frequency (d year−1); BW the body weight (kg); AT the averaging time (years); IngR symbolizes the ingestion rate (mg d−1); AF is the skin adherence factor (mg cm−2 d−1); ABS represents the dermal absorption factor (dimensionless); SA is the skin surface area of an average man or woman (cm2); RfD means the reference dose referred to each intake path (mg kg−1 d−1), employed to approximate the upper limit for determining if the daily exposure has a low probability of inducing adverse health effects.
In this work, InhR takes distinct values for women (11.2 m−3 d−1) and men (15.3 m−3 d−1) [Citation12,Citation31]. The results were extrapolated to one year from the sampling periods, which covered nearly two months. This way, EF = 1 d y−1 and ED = 1 y. Average body weight for women is assumed to be 60 kg and 70 kg for men [Citation45], AT = 365 d, for extrapolating to one year, while IngR = 100 mg d−1; SA is equal to 1694 cm2 for women and 2011 cm2 for men [Citation12], while ABS = 0.01. Finally, RfD takes different values for each element [Citation12,Citation45].
Only a few elements were considered in this work as posing a potential risk to human health (Cr, Mn, Fe, Ni, Cu, Zn, and Pb), mostly due to the possible occurrence of cancer. In this regard, the other elements (Al, Si, P, S, Cl, K, Ca, Ti, and V) are not taken into account for HQ computing, because no reference values exist or are not considered carcinogenic.
2.4. Enrichment factors
As another test of the possible origin of the registered elements, the Enrichment Factor (EFi) can be used, taking Si as a reference element, through the equation [Citation46]:
where Ci,S and CSi,S are the concentrations of element i and Si in a sample, respectively, and Ci,EC and CSi,EC are the concentrations of element i and Si in the average Earth crust [Citation47]. When EFi has a value smaller than 10, a geogenic origin can be expected; otherwise, the element is probably anthropogenic.
3. Results and discussion
3.1. Gravimetric mass concentrations
As mentioned above, 50 valid samples were collected at the Aerosols laboratory (25 indoors and 25 outdoors), 47 samples at the Van de Graaff laboratory (23 indoors and 24 outdoors), and 42 filters at the mechanical workshop (21 indoors and 21 outdoors). Their gravimetric mass and elemental concentrations were measured for all of them.
To check the validity of the gravimetric mass concentrations measured in this work, data obtained from the Benito Juárez station of the RAMA were used as a reference [Citation39]. compares both data sets for the mechanical workshop campaign. The correlation between them is very good (r = 0.911), although the slope is different from unity (0.764 (0.092)), a fact explained by the 5.5 km separation between both sites. Thus, the data presented here can be considered reliable.
Figure 2. PM10 mass concentrations measured in this work as a function of those measured at the Benito Juárez RAMA station.

presents Tukey diagrams for the data from all sites and displays the official Mexican and World Health Organization (WHO) standards (70 µg m−3 and 50 µg m−3 24 h averages, respectively) [Citation48,Citation49]. The WHO standard is applicable to both outdoor and indoor environments [Citation47]. The Mexican standard was never surpassed. This happened only on one occasion with the WHO reference at both laboratories, but only outdoors, and several days during the workshop campaign. Thus, in this regard, it can be said that the studied indoor environments are not hazardous.
Figure 3. Tukey diagrams for PM10 gravimetric mass concentrations measured at the three sites, showing the Mexican official standard (dotted line) and the WHO standard (dash-dotted line).

Furthermore, through the application of ANOVA (α = 0.05, p-value <.001) among all the sites, it was possible to establish that the gravimetric mass concentrations are significantly higher outdoors in each case, contrasted to the simultaneous indoor sampling. This means that the indoor sources may not contribute more to the PM10 concentrations than the outdoor sources in case infiltration of the latter particles occurs. It must be noted, too, that the mean mass concentrations grew with time in the outdoor environments, probably because of the slow increase of activities from 2021 to 2022 associated with the pandemic lockdown.
3.2. XRF results
A total of 18 elements (Al, Si, P, S, Cl, K, Ca, Ti, V, Cr, Mn, Fe, Ni, Cu, Zn, Se, Br, and Pb) were detected, although not in all the samples. displays the number of appearances, mean concentrations, and standard deviations for each element and site. After applying ANOVA, it was possible to identify significantly higher concentrations by comparing the indoor and outdoor values at each site (written with bold typeface in ).
Table 1. Basic statistics of measured concentrations (µg m−3) in PM10..
To compare with recent studies of outdoor PM10 at the same site, the paper by Mejía-Ponce et al. (2022), with data from a campaign carried out during the dry-warm season in 2019, reports a Si concentration of 2.12 (0.53) µg m−3, similar to the outdoor concentrations measured in the present work. Regarding S, in 2019, a mean concentration of 1.21 (0.63) µg m−3 was obtained, higher only than the mean concentration determined in 2021 (Aerosol laboratory campaign).
With this information, it is possible to examine which elements may be enriched indoors as compared to outdoors through the average ratio of indoor/outdoor concentrations, as given in . Elements with few simultaneous appearances in both environments were not included (V, Ni, and Se). The ratios of geogenic elements Al, K, Ca, Ti, and Fe, are smaller than unity at the Aerosols and Van de Graaff laboratories, showing that, due to limited ventilation of the spaces, a high number of particles from the exterior did not enter the experimental areas. Also, Zn was less abundant at the Aerosols laboratory, and P at the Van de Graaff. In contrast, Si, and Pb had higher concentrations inside the Aerosols laboratory, suggesting the presence of a local Si source, while Pb is present in several radiation shields. The Cu concentrations were very high at the Van de Graff laboratory, perhaps because of the large number of electric cables in this facility. The workshop is ventilated almost all the time; thus, it is not surprising to see that the concentrations of the soil-derived particles are very similar indoors and outdoors. Moreover, Si and Ca presented higher contents outdoors, in contrast with Cl, Mn, Cu, Zn, and Pb, which were higher indoors. The latter elements might be associated with several materials used at the workshop [Citation32,Citation33]. Finally, it is remarkable that S had almost equal concentrations indoors and outdoors, revealing that the ventilation processes in all the sites allowed the penetration of particles containing this anthropogenic element. The sources of the elements in the outdoor environment at the sampling site have been discussed elsewhere [Citation38].
Figure 4. Ratios of indoor to outdoor elemental concentrations measured at the three sampling sites. The bars represent type a uncertainties.

Additionally, the PM10 gravimetric mass concentrations measured in the present work can be contrasted with the results found in other studies. Here, PM10 had 24 h averages of 26.3 ± 9.2 µg m−3, 29 ± 12 µg m−3, and 40 ± 10 µg m−3, at the Aerosols laboratory, Van de Graaff laboratory, and mechanical workshop, respectively. Chen et al. [Citation23] assessed an 83.6 µg m−3 concentration at a research laboratory in Lao-Pi, Taiwan; Ugranli et al. [Citation24] determined a 63 ± 32 µg m−3 concentration at chemical laboratories in Izmir, Turkey; Mishra et al. [Citation30] found a value of 114 µg m−3 at a chemical laboratory in Narajna, India; Demou et al. [Citation33] measured 160 ± 120 µg m−3 at a machine shop in Zurich, Switzerland; and Zhou et al. [Citation35] obtained a 90.0 µg m−3 value in a printed circuit workshop located in Shanghai, China. As can be seen, the concentrations determined in the Mexico City environments are much lower than those of similar environments in other sites.
shows the mean EF for all the elements with a significant number of appearances. As expected, Al, K, Ca, Ti, Mn, and Fe have EF close to unity, so the geogenic origin is confirmed. The remaining elements are emitted by anthropogenic sources.
3.3. Cluster analysis
A second assessment was conducted to verify the possible origin of the elements and relationships among the indoor and outdoor concentrations by applying Cluster Analysis (CA). The dendrograms obtained with CA are shown in . The Aerosols laboratory dendrogram () presents five groups: the red cluster corresponds mostly to anthropogenic elements found indoors, with a contribution of soil-derived Al and Si; the blue one is associated with indoor geogenic elements; the green group is a mixture of indoor and outdoor anthropogenic elements; the purple cluster includes the highly correlated S indoor and outdoor contributions, plus several outdoor anthropogenic elements; and the outdoor geogenic elements constitute the dark yellow group. This dendrogram shows that, even though there is limited ventilation in the laboratory, almost no penetration of the particles occurs towards the laboratory. A similar behavior is observed for the Van de Graaff laboratory (), where the red and dark yellow clusters comprise geogenic elements, for indoor and outdoor environments, respectively; the blue group includes indoor elements (Si associated with Cu), and both indoor and outdoor S; the green cluster is composed by anthropogenic outdoor elements, indicating there is also a limited Pb penetration; finally, the purple group is constituted by outdoor anthropogenic elements. Again, it can be concluded that the limited ventilation of the laboratory allows only penetration of certain elements. In the workshop dendrogram (), only three clusters could be found. It is noticeable that all the indoor and outdoor geogenic elements (red group) are fully associated, indicating that the indoor particles were transported from the outdoor environment, thanks to the wide ventilation; the blue cluster contains mostly indoor anthropogenic elements; however, it must be seen that indoor metals (Cr, Mn, and Fe) appear in this group, most probably originated by the materials used at the workshop, and, again, the indoor and outdoor S; the green cluster, finally, is a mixture of indoor and outdoor elements, showing also penetration of Cl-rich particles.
Figure 6. Dendrograms obtained for the indoor and outdoor elemental concentrations measured at the (a) Aerosols and (b)Van de Graaff laboratories, and (c) the mechanical workshop.

The behavior of S in the three sites is remarkable. Despite the limited ventilation at the two laboratories, it can be seen that S particles have essentially the same outdoor origin. demonstrates this effect, showing the high correlation between this element’s indoor and outdoor concentrations. It must be noted, too, that Si only correlates significantly with Ca indoors at the Van de Graaff laboratory and the workshop, probably because of the building materials (concrete).
Figure 7. Sulfur concentrations measured indoors as a function of the sulfur concentrations measured outdoors. The numbers between parentheses represent the uncertainty of the fitted parameters.

The detection of all the reported elements agrees well with the finding of other authors, like Ugranli et al. [Citation24] and Hamdan et al. [Citation50] for chemical laboratories or Iavicoli et al. [Citation32] at a mechanical engineering factory. In the sites studied in this work, penetration of the particles to the indoor environment is very important, in contrast to what was reported by Reynoso-Cruces et al. [Citation31] for a particle accelerator laboratory, which is a closed environment, and no relevant infiltration could be noted. Moreover, Zhou et al. [Citation35] distinguished many elements also present in the environments studied in the present work, like Cr, Fe, Ni, Cu, and Zn, in a printed circuit board workshop.
3.4. SEM results
The studies with SEM and EPMA support the identification of the origin of the particles, exemplified by . presents the image and X-ray spectrum of a geogenic particle (an approximate diameter of 10 µm) collected at the Aerosols laboratory, showing the presence mainly of Si, with traces of Mg and Al, including contributions of C and O, probably from the substrate and the oxides in the particle. This fact coincides with the observation that Si does not appear in the same cluster of other soil-derived elements (K, Ca, Ti, Fe). contains the image and EPMA spectrum of a 2.2 µm particle, collected outdoors from the same laboratory, displaying geogenic elements plus S and Zn, in full agreement with the fourth cluster in . Regarding indoor particles collected at the mechanical workshop, shows an almost spherical particle, with a 3 µm diameter, composed mainly of Fe; undoubtedly, the origin of the particle is a result of welding of pieces containing Fe inside the workshop. Iavicoli et al. [Citation32] also observed regularly shaped particles, created by welding processes.
Figure 8. SEM images of a particle collected at (a) the aerosols laboratory, indoors, with the corresponding X-ray spectra obtained with EPMA; this particle has a geogenic origin. (b) particle collected at the aerosols laboratory, outdoors; the particle is assumed to have a geogenic origin with S and Zn contributions. (c) particle collected at the mechanical workshop, indoors; a welding process produced the particle. (d) particle collected at the mechanical workshop, indoors; it appears to be a conglomerate of smaller components with S attachments. (e) particle collected outdoors at the Van de Graaff laboratory; the elements correspond to a geogenic particle.

Moreover, exhibits an irregular conglomerate of smaller particles (approximately 7 µm × 5 µm), constituted mostly by C, O, S, Fe, and Pb. Considering that S has been infiltrated from outdoors, it is feasible that smaller airborne particles were attached to other fragments produced in the workshop. Finally, a particle captured outdoors during the Van de Graaff campaign is composed of the typical elements of geogenic origin (O, Na, Mg, Al, Si, K, Ca, Ti, and Fe) is shown in ; its shape is completely irregular, with a length of about 6 µm. Again, the agreement with the dendrogram presented in is excellent.
In summary, it is found that a very important fraction of particles penetrates from the outdoor atmosphere toward the indoor environment, with contributions either from building materials or activities identified indoors. There is full agreement between XRF and SEM + EPMA results and cluster analysis.
3.5. Health risk assessment
As mentioned above, the risk assessment was carried out for only seven elements: Cr, Mn, Fe, Ni, Cu, Zn, and Pb, because the remaining elements are not considered carcinogenic, or there are no reference values. The parameters specific to each element, required for calculating the HQ values, were taken from the IRIS database [Citation45] and other works [Citation12,Citation51], because IRIS does not include RfD for elements like Pb. Several parameters necessary for the HQ evaluation are given in Table S1.
The computed HQ values are shown in for the Aerosols, Van de Graaf, and workshop sites. It can be seen that, despite exposure to all these elements, most of them have no apparent health risk. Nevertheless, there is a possible risk due to Cr ingestion, because the HQ values are larger than 1. The sources of this element may be different on each site. According to the CA results, Cr is associated with geogenic elements, but at the workshop, it may be produced by either welding or machining activities.
Table 2. Risk assessment (hazard quotients) results for inhalation, ingestion, and dermal absorption of PM10 at the aerosols laboratory.
Table 3. Risk assessment (hazard quotients) results for inhalation, ingestion, and dermal absorption of PM10 at the Van de Graaff laboratory.
Table 4. Risk assessment (hazard quotients) results for inhalation, ingestion, and dermal absorption of PM10 at the mechanical workshop.
displays a comparison of HQ computed for the three indoor environments and the average outdoor concentrations. There, it is possible to note that the HQ values by inhalation, ingestion, and dermal absorption are similar for all elements, except Mn and Cr by ingestion and dermal absorption, which are significantly higher in the Van de Graaff laboratory and the workshop. This is explained by the higher concentrations in those environments, especially the workshop, where frequently materials containing Cr are used. Nevertheless, it must be recalled that the time spent by the occupants of the studied working sites in outdoor environments is less than that spent indoors; thus, the health risk caused by outdoor particles should be lower than the values quoted here.
Figure 9. Hazard quotients (HQ) of Fe, Ni, Cu, Zn, Mn, Cr, and Pb, due to (a) inhalation, (b) ingestion, and (c) dermal absorption, due to exposure to PM10 outdoors (average concentrations), and the three indoor environments studied in the present work.

Additionally, contains the CR results for Cr, Ni, and Pb, the only elements with recommended coefficients for its evaluation. In this regard, none of these elements risk human health in the studied environments.
Table 5. Cancer risk coefficients for selected elements.
Considering similar indoor locations, the results are comparable to those given by Zhou et al. [Citation35] in the printed circuit board workshop, where only Cr may represent a risk to human health. Similarly, Reynoso-Cruces et al. [Citation22] did not find any significant risk due to PM10 or PM2.5 exposure in a particle accelerator laboratory.
Finally, it is necessary to stress the limitations of the present study. The sampling periods were rather short, so it was necessary to extrapolate the risk assessments to a full year. The time resolution during sample collection does not allow for identifying periods of different activities carried out in each site during the day or night. The data refer only to elemental concentrations and does not include other chemical species (such as volatile organic compounds or ionic species), that may be more harmful to human health. Future studies should have a wider spectrum of complementary chemical analyses.
4. Conclusions
The present paper is a continuation of previous studies, aimed at measuring the elemental concentration of PM10 in scientific research environments in the MAMC, including risk assessments due to exposure to these particles, through inhalation, ingestion, or dermal absorption.
In all the cases, the gravimetric mass concentrations were higher outdoors than indoors, although the elemental concentrations were generally very similar. The evaluation of enrichment factors allowed to distinguish the elements with a geogenic origin that were present in the particles, and to recognize their indoor and outdoor differences. The significantly high correlation between indoor and outdoor concentrations for certain elements, found through Cluster Analysis, demonstrated the occurrence of an important penetration of the particles toward the indoor environments due to ventilation. It was mainly observed for S and soil-derived elements. Including SEM plus EPMA analyses was very useful in understanding the results obtained with XRF.
The PM10 concentrations were lower than those measured in other sites around the world. Also, the possible health risks were similar to results from other studies.
Finally, no significant risk to human health due to exposure to the elements present in PM10 was detected, except for Cr via ingestion of the airborne particles.
Authors’ contributions
Salvador Reynoso-Cruces participated in the design of the campaigns, collected and analyzed samples, read and corrected the manuscript; Javier Miranda-Martín-del-Campo designed the study, participated in the design of the campaigns, analyzed the data, and wrote the first version of the manuscript; Juan Carlos Pineda-Santamaría collected and analyzed samples, read and corrected the manuscript. All authors approved the final manuscript.
Supplemental Material
Download Zip (617.4 KB)Acknowledgments
This work was supported in part by Dirección General de Asuntos del Personal Académico, Universidad Nacional Autónoma de México, under grant IN-101021. SRC recognizes the support of CONACyT through a scholarship. The authors acknowledge D.L. Serrano for assistance during sampling campaigns, R. Hernández and C.R. Magaña’s technical support for SEM and EPMA analyses, and J.E. Pérez’s support for sampling at the mechanical workshop.
Disclosure statement
No potential conflict of interest was reported by the author(s).
Data availability statement
The datasets used and/or analyzed during the current study are available from the corresponding author upon reasonable request.
Supplementary material
Supplemental data for this article can be accessed online at https://doi.org/10.1080/26395940.2023.2232108
Additional information
Funding
References
- Szoboszlai Z, Furu E, Angyal A, et al. Investigation of indoor aerosols collected at various educational institutions in Debrecen, Hungary. X-Ray Spectrom. 2011;40(3):176–341. doi: 10.1002/xrs.1323
- Elbayoumi M, Ramli NA, Yusof NFFM, et al. Spatial and seasonal variation of particulate matter (PM10 and PM2.5) in middle eastern classrooms. Atmospher Environ. 2013;80:389–397. doi: 10.1016/j.atmosenv.2013.07.067
- Sotiriou M, Ferguson SF, Davey M, et al. Measurement of particle concentrations in a dental office. Environ Monit Assess. 2018;137(1–3):351–361. doi: 10.1007/s10661-007-9770-7
- Seppänen O, Fisk WJ, Lei QH. Ventilation and performance in office work. Indoor Air. 2005;16(1):28–36. doi: 10.1111/j.1600-0668.2005.00394.x
- Vilcekova S, Estokova A, Burdova EK, et al. Investigation of particulate matter concentration in offices. Fresen Environ Bulletin. 2017;26:1225–1233.
- Ruan T, Rim D. Indoor air pollution in office buildings in mega-cities: effects of filtration efficiency and outdoor air ventilation rates. Sustainable Cities Soc. 2019;49:101–109. doi: 10.1016/j.scs.2019.101609
- Mundackal A, Ngole-Jeme VM. Evaluation of indoor and outdoor air quality in university academic buildings and associated health risk. Int J Environ Health Res. 2020;32(5):1076–1094. doi: 10.1080/09603123.2020.1828304
- Zhao X, Lin L, Zhang Y. Contamination and human health risks of metals in indoor dust from university libraries: a case study from Qingdao, China. Human Ecol Risk Assess. 2019;27(1):152–161. doi: 10.1080/10807039.2019.1697851
- Taner S, Pekey B, Pekey H. Fine particulate matter in the indoor air of barbeque restaurants: elemental compositions, sources and health risks. Sci Total Environ. 2013;454:79–87. doi: 10.1016/j.scitotenv.2013.03.018
- Kuo SC, Hsieh LY, Tsai CH, et al. Characterization of PM2.5 fugitive metal in the workplaces and the surrounding environment of a secondary aluminum smelter. Atmos Environ. 2007;41(32):6884–6900. doi: 10.1016/j.atmosenv.2007.04.038
- Madureira J, Slezakova K, Silva AI, et al. Assessment of indoor air exposure at residential homes: inhalation dose and lung deposition of PM10, PM2.5 and ultrafine particles among newborn children and their mothers. Sci Total Environ. 2020;717:137293. doi: 10.1016/j.scitotenv.2020.137293
- Embiale A, Chandravanshi BS, Zewge F, et al. Health risk assessment of trace elements through exposure of particulate matter-10 during the cooking of Ethiopian traditional dish sauces. Environ Toxicol Chem. 2020;102(1–4):151–169. doi: 10.1080/02772248.2020.1770257
- Negral L, Suárez-Peña B, Zapico E, et al. Anthropogenic and meteorological influences on PM10 metal/semi-metal concentrations: implications for human health. Chemosph. 2020;243:125347. doi: 10.1016/j.chemosphere.2019.125347
- Embiale A, Chandravanshi BS, Zewge F, et al. Health risk assessment of total volatile organic compounds, particulate matters and trace elements in PM10 in typical living rooms in Addis Ababa, Ethiopia. Int J Environ Anal Chem. 2022;102(18):6583–6601. doi: 10.1080/03067319.2020.1814266
- Embiale A, Chandravanshi BS, Zewge F, et al. Investigation into trace elements in PM10 from the baking of injera using clean, improved and traditional stoves: emission and health risk assessment. Aerosol Sci Engg. 2019;3(4):150–163. doi: 10.1007/s41810-019-00049-y
- Koukoulakis KG, Chrysohou E, Kanellopoulos PG, et al. Trace elements bound to airborne PM10 in a heavily industrialized site nearby Athens: seasonal patterns, emission sources, health implications. Atmos Pollut Res. 2019;10(4):1347–1356. doi: 10.1016/j.apr.2019.03.007
- Rabha S, Subramanyam KSV, Sawant SS, et al. Rare-earth elements and heavy metals in atmospheric particulate matter in an urban area. ACS Earth Space Chem. 2022;6(7):1725–1732. doi: 10.1021/acsearthspacechem.2c00009
- Morton-Bermea O, Garza-Galindo R, Hernández-Álvarez E, et al. Recognition of the importance of geogenic sources in the content of metals in PM2.5 collected in the Mexico City metropolitan area. Environ Monit Assess. 2018;190(2):83. doi: 10.1007/s10661-017-6443-z
- Roy D, Seo YC, Kim S, et al. Human health risks assessment for airborne PM10-bound metals in Seoul, Korea. Environ Sci Pollut Res. 2019;26(23):24247–24261. doi: 10.1007/s11356-019-05213-y
- Zhang J, Wu L, Fang X, et al. Elemental composition and health risk assessment of PM10 and PM2.5 in the roadside microenvironment in Tianjin, China. Aerosol Air Qual Res. 2018;18(7):1817–1827. doi: 10.4209/aaqr.2017.10.0383
- Cerón Bretón JG, Cerón Bretón RM, Espinosa Guzmán AA, et al. Trace metal content and health risk assessment of PM10 in an urban environment of León, Mexico. Atmosph. 2019;10(10):573. doi: 10.3390/atmos10100573
- Reynoso-Cruces S, Miranda-Martín-Del-Campo J, Pineda-Santamaría JC. A study of elemental composition and risk assessment due to exposure to indoor PM10 in two residences in Mexico City. Atmosph. 2023;14(4):734. doi: 10.3390/atmos14040734
- Chen SJ, Lin TC, Tsai JH, et al. Characteristics of indoor aerosols in college laboratories. Aerosol Air Qual Res. 2013;13(2):649–661. doi: 10.4209/aaqr.2012.07.0176
- Ugranli T, Toprak M, Gursoy G, et al. Indoor environmental quality in chemistry and chemical engineering laboratories at Izmir institute of technology. Atmos Pollut Res. 2015;6(1):147–153. doi: 10.5094/APR.2015.017
- Ugranli T, Güngörmüş E, Sofuoğlu A, et al. Indoor air quality in chemical laboratories. Comprehens Analy Chem. 2016;73:859–878. doi: 10.1016/bs.coac.2016.04.007
- Sulaiman FR, Suratmin MA. Composition of metal in indoor dust from university laboratories. Malaysian J Med Health Sci. 2020;16(SUPP11):28–32.
- Perrino C, Pelliccioni A, Tofful L, et al. Indoor PM10 in university classrooms: chemical composition and source behaviour. Atmos Environ. 2022;287:119260. doi: 10.1016/j.atmosenv.2022.119260
- Fuehne D, Gallagher P, Hjeresen D, et al. Environmental surveillance at Los Alamos during 2008 (no. LA-14407-ENV). Los Alamos National Lab; 2009. doi: 10.2172/1186657
- Žitnik M, Kastelic A, Rupnik Z, et al. Time-resolved measurements of aerosol elemental concentrations in indoor working environments. Atmos Environ. 2010;44:4954–4963. doi: 10.1016/j.atmosenv.2010.08.017
- Mishra AK, Mishra P, Gulia S, et al. Assessment of indoor fine and ultra-fine particulate matter in a research laboratory. In: Sharma A, Goyal R Mittal R, editors. Indoor environmental quality. Lecture notes in civil engineering. Vol. 60. Singapore: Springer Singapore; 2020. p. 19–26. doi: 10.1007/978-981-15-1334-3_3
- Reynoso-Cruces S, Hernández-López AE, Miranda J, et al. Elemental characterization and risk assessment of indoor aerosols in an electrostatic particle accelerator laboratory. Environ Pollut Bioavailabil. 2021;33:334–346. doi: 10.1080/26395940.2021.1988869
- Iavicoli I, Leso V, Fontana L, et al. Characterization of inhalable, thoracic, and respirable fractions and ultrafine particle exposure during grinding, brazing, and welding activities in a mechanical engineering factory. J Occup Environ Med. 2013;55(4):430–445. doi: 10.1097/JOM.0b013e31827cbabe
- Demou E, Mutamba G, Wyss F, et al. Exposure to PM1 in a machine shop. Indoor Built Environ. 2009;18(6):514–523. doi: 10.1177/1420326X09347244
- Isaxon C, Pagels J, Gudmundsson A, et al. Characteristics of welding fume aerosol investigated in three Swedish workshops. J Phys Conf Ser. 2009;151:012059. doi: 10.1088/1742-6596/151/1/012059
- Zhou P, Guo J, Zhou X, et al. PM2.5, PM10 and health risk assessment of heavy metals in a typical printed circuit boards manufacturing workshop. J Environ Sci. 2014;26(10):2018–2026. doi: 10.1016/j.jes.2014.08.003
- Reynoso-Cruces S, Hernández AE, Miranda J, et al. Calibration of MiniVol TA 5.0 atmospheric aerosol samplers. Mexico City(in Spanish): Instituto de Física, UNAM, internal report; 2023.
- Retama A, Ramos-Cerón M, Rivera-Hernández O, et al. Aerosol optical properties and brown carbon in Mexico City. Environ Sci: Atmos. 2022;2(3):315–334. doi: 10.1039/d2ea00006g
- Mejía-Ponce LV, Hernández-López AE, Miranda-Martín-Del-Campo J, et al. Elemental analysis of PM10 in southwest Mexico City and source apportionment using positive matrix factorization. J Atmos Chem. 2022;79(3):167–198. doi: 10.1007/s10874-022-09435-2
- SEDEMA. [Data bases] Red Automática de Monitoreo Atmosférico (RAMA). Secretaría de Medio Ambiente, Mexico City. 2023 [cited 2023 Jan 15]. Available from: http://www.aire.cdmx.gob.mx/default.php?opc=%27aKBh%27
- Espinosa AA, Reyes-Herrera J, Miranda J, et al. Development of an X-ray fluorescence spectrometer for environmental science applications. Instrument Sci Technol. 2012;40(6):603–617. doi: 10.1080/10739149.2012.693560
- Mejía-Ponce LV, Hernández-López AE, Reynoso-Cruces S, et al. Improvements to the X-ray spectrometer at the aerosol laboratory, instituto de física, UNAM. J Nuclear Phys. 2018;6(1):57–60. doi: 10.15415/jnp.2018.61009
- XRF Group. Quantitative X-ray analysis system, computer manual series no.21. Vienna: Austria; 2007. p. 138.
- Espinosa AA, Miranda J, Pineda JC. Uncertainty evaluation in correlated quantities: application to elemental analysis of atmospheric aerosols. Rev Mexicana de Física. 2010;56:134–140.
- Hernández-López AE, Miranda J, Mugica V, et al. A study of PM2.5 elemental composition in southwest Mexico City and development of receptor models with positive matrix factorization. RICA. 2021;37:67–88. doi: 10.20937/RICA.54066
- EPA. Exposure factors handbook: 2011 edition. In: Environmental protection agency. Washington, DC, Springfield, VA: National Center for Environmental Assessment; 2011 [cited November 1, 2020], from National Technical Information Service and online at. http://www.epa.gov/ncea/efh
- Paredes-Gutiérrez R, López-Suárez A, Miranda J, et al. Comparative study of elemental contents in atmospheric aerosols from three sites in Mexico City using PIXE. Rev internacional de contaminación ambiental. 1997;13:81–85.
- Demayo A. Composition of average earth crust. In: Weast RC, editor. Handbook of chemistry and physics. 65th ed. Boca Raton: CRC Press; 1984. p F–146.
- SSA. Mexican official standard NOM-025-SSA1-2021, environmental health. Permissible limits for PM10 and PM2.5 suspended particles concentrations in air and evaluation criteria. Mexico City: Secretaría de Salud. Diario Oficial de la Federación. 2023 [cited 2023 Feb 22].
- World Health Organization. Occupational and Environmental Health Team. WHO Air quality guidelines for particulate matter, ozone, nitrogen dioxide and sulfur dioxide: global update 2005: summary of risk assessment. Geneva: World Health Organization; 2006 [cited 2023 Feb 10]. Available from: https://apps.who.int/iris/handle/10665/69477
- Hamdan NM, Alawadhi H, Jisrawi N. Elemental and chemical analysis of PM10 and PM2.5 indoor and outdoor pollutants in the UAE. Int J Environ Sci Develop. 2015;6(8):566–570. doi: 10.7763/IJESD.2015.V6.658
- Cheng X, Huang Y, Zhang SP, et al. Characteristics, sources, and health risk assessment of trace elements in PM10 at an urban site in Chengdu, Southwest China. Aerosol Air Qual Res. 2018;18(2):357–370. doi: 10.4209/aaqr.2017.03.0112