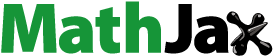
ABSTRACT
In this work, column experiments were applied to investigate the transport of four kinds of microplastics (MPs) under a series of ionic strength (IS) conditions. Under 0.1 mM IS, PMMA MPs showed the highest mobility, as well as the PET MPs showed the lowest mobility. With the IS increased, the transport of all kinds of MPs in porous media was generally reduced to the minimum. The transport reducing efficiency of PMMA MPs and PET MPs was lower than that of the PVC MPs and PP MPs. It was found that both the hydro-chemical conditions and basic properties showed combined effect on MPs transport in porous media. The DLVO results were well used to describe the deposition of MPs onto sand surface and excavate the transport behaviors of MPs. The one-site kinetic deposition model was successfully conducted to fit the observed breakthrough curves. Findings from this study elucidated the key factors controlling the MPs transport in porous media, contributing to the prediction and assessment of the environmental risks of MPs.
1. Introduction
Plastic is a synthetic polymer material with high chemical stability and strong plasticity, which is widely used in construction, packaging, textile, medicine, agricultural production, electronic manufacturing and other industries [Citation1,Citation2]. The increase has resulted in a great number of plastic wastes accumulated in the environment, leading to the pollution and potential risks to the ecosystems [Citation3]. Only a small fraction of about 6–26% of plastic wastes are recycled, lots of the plastic wastes remain in the environment [Citation4]. The particles remaining in the environment can break into microplastic (MPs, <5 mm) [Citation4–6]. MPs can be easily ingested by organisms at different trophic levels and accumulate along the food webs [Citation7,Citation8]. MPs in the human body may result in maternal metabolic disorder, malnutrition, inflammation, and chemical poisoning [Citation9,Citation10]. On the other hand, MPs can be an important carrier for the transport and accumulation of heavy metals and organic pollutants in the environment. Older MPs transported from distant areas can accumulate other pollutants and are therefore at greater risk than local MPs [Citation11]. In addition to the contaminants in external environment, MPs may also release some pollutants, such as phthalic acid esters and polycyclic aromatic hydrocarbons during the transport process [Citation12]. It also has been reported that MPs also can affect the bulk density, water holding capacity, water-stable aggregation permeability, and porosity of soils [Citation8]. Pollution by MPs is an increasingly emerging threat to the terrestrial environment as they have the potential to mobilize into the environment and subsequently lead to serious problems [Citation13–16]. MPs contamination is an issue of high concern and has been listed as the second-most emerging ecological issue after global warming and environmental pollution and the concern has been justified and expressed in data from world environment organizations.
Mulching, irrigation, sewage sludge landfills, application of compost, inundation of wastewater, automobile tire debris, and atmospheric deposition are considered to be major contributors to MPs in the soil environment. The findings show that in Europe and North America, about 700,000 tons of polymers enter the soil each year, exceeding the global burden of polymers in Marine surface waters (93,000–236,000 tons) [Citation17]. In Shanghai (eastern China), MPs were found to be ~ 78.00 particles kg−1 in shallow soil (3 cm) and ~ 62.50 particles kg−1 in deep soil (6 cm) in farmland, and ~ 10.3 particles kg−1 (10 cm) in farmland paddy soils [Citation18]. On the other hand, 600–10,400 particles kg−1 MPs were found in agricultural land in Mellipilla, Chile [Citation19]. Soil migration, landfill leachates, septic effluents, sinkholes, surface runoffs, and anthropogenic activities are the potential ways of MPs entering groundwater [Citation20]. Due to their recalcitrant nature, microplastics can remain in soil and groundwater for a long time. After MPs enters the complex heterogeneous system of soil and groundwater, it may undergo different environmental processes, resulting in various ecological risks. The fate and environmental behavior of MPs in soil and groundwater should be an important issue that requires further research to promote awareness and understanding. It is impossible to assess and predict the supply and environmental behavior of MPs without considering their fate and migration in soil and groundwater. Several valuable laboratory studies, controlled by multiple physicochemical factors, have investigated the transport and fate of MPs in porous media. The results showed that the mobility of MPs was influenced by ionic strength (IS), cation type, dissolved organic matter (DOM) and surfactant [Citation21–30]. The mobility of MPs usually decreases with the increase of IS and the presence of polyvalent cations [Citation31]. Transport of MPs is usually inhibited with a decrease in pH, which can be attributed to deprotonation of functional groups. The hydrophilicity of MPs can be enhanced by deprotonation of functional groups, which may contribute to their migration. However, under certain conditions, such as the presence of polyvalent cations, deprotonation of functional groups may provide more reaction sites for crosslinking between MPs and porous media, thus inhibiting the mobility of MPs.
In addition, surface functional groups are one of the main basic chemical factors of MPs. The hydrophilicity and hydrophobicity of microplastics are affected by their surface functional groups, which in turn affect their deposition and transport. MPs surface coating materials, such as polyacrylic acid and polystyrene sulfonic acid, are commonly observed to stabilize microplastics and promote their migration in porous media [Citation32]. For example, MPs coated with DOM in porous media usually show higher mobility than those uncoated. On the other hand, physical factors, such as concentration, flow rate, media grain size, moisture content, temperature also may influence the transport of MPs. The retention of microplastics in porous media is inhibited as their concentration increases, thus improving transport. Similar to other micro/nanoparticle particles, the increased flow rate usually facilitates the transport of MPs in porous media due to the clogging effect [Citation33]. Since the kinetic energy of a particle collision is proportional to the square of the particle trajectory velocity, the particle transport volume usually increases with the velocity. The retention rate of microplastics in porous media usually increases with the decrease of particle size, indicating that the removal efficiency of microplastics is higher in fine sand. Compared with saturated porous media, microplastics usually exhibit lower mobility in unsaturated porous media due to the influence of gas–water interface and membrane tension [Citation25]. MPs exposed to the freeze–thaw cycle (−10°C to 10°C) showed significant aggregation and reduced mobility compared to being kept at a constant 10°C [Citation34]. Microorganisms are prevalent in soil and groundwater environments and may affect the retention and transport of microplastics. Microplastics may be captured by biofilms or swallowed by microorganisms during transport, resulting in increased retention and reduced mobility of microplastics in porous media. The surface of the medium is further affected by growth media or microbiome related extracellular polymeric substances, which further affect the retention behavior of microplastics in porous media [Citation35]. On the other hand, certain pollutants, such as heavy metals and organic pollutants, may be adsorbed on MPs through π-π, complexation and electrostatic interactions and co-transported with MPs in soil and groundwater systems [Citation36].
Although the fate and migration of MPs have received increasing attention in recent years, almost all recent research has been limited to the study of normalized polystyrene microspheres. The normalized polystyrene microspheres have uniform shape and single characteristics, and cannot fully represent all MPs in the environment. The physical properties of MPs, such as particle length, morphology, and density, also affect filtration processes and stress conditions, which can affect the transport of MPs in soil and groundwater systems [Citation22]. For example, MPs in particle form exhibit greater fluidity than MPs in fragment, filament, film, or foam form, suggesting that morphology also has a considerable impact on retention and transport of MPs [Citation37]. High-density MPs also exhibit lower mobility in porous media due to high gravity sedimentation [Citation38]. Different MPs have different surface properties, such as functional groups. Surface functional group is one of the main basic chemical factors of MPs. It affects the hydrophilicity of MPs, and then affects its transport. Some hydrophilic functional groups can improve the hydrophilicity of MPs in water environment, thus inhibiting the deposition of MPs on porous media and improving the mobility of MPs in soil and groundwater [Citation39]. For this reason, the studies focused on the transport of diversified MPs in porous media are urgent.
In this study, a series of column experiments were conducted to provide novel insights into understanding the transport behaviors of polypropylene (PP) MPs, polyvinyl chloride (PVC) MPs, polyethylene terephthalate (PET) MPs and polymethyl methacrylate (PMMA) MPs in saturated porous media under various IS conditions. The main objectives of this study are to: (1) understand the interactions between each kind of MPs and porous media under various IS conditions; (2) investigate the mobility of each kind of MPs under various IS conditions; (3) test the numerical models for simulating diversified MPs transport in porous media under various IS conditions.
2. Materials and methods
2.1. The preparation of porous media and MPs suspension
Quartz sand (with the diameter 0.75–0.85 mm) was used to construct the porous media. Clean the sand successively with NaOH solution (0.25 M), diluted nitric acid (1.5 M) and deionized water (DI) to remove dirt. The washed sand is dried at 45°C and stored. Store the sand in an airtight jar under dry conditions at room temperature (25°C). PP MPs, PVC MPs, PET MPs and PMMA MPs purchased from Huachuang plastic materials Co., Ltd (Dongguan, China) were used in this study. The sizes are about 6 μm.
The background solution for each test was prepared with deionized water and NaCl, respectively, to the required gradient concentrations (0.1, 1 and 10 mM). The pH conditions for all tested MPs suspensions were graded to 5.6 with tiny HCl (0.1 M) or NaOH (0.1 M) stock solution. For preparing the MPs suspension, 10 mg each MPs was mixed with 100 mL tested background solution. After stirring, the mixture was then ultrasonicated for 60 min for sufficient dispersion. During the ultrasonic process, the mixture was stirred every 30 minutes. The Zeta potential of all MPs and sand under test IS conditions was determined using Nanotrac Wave II (Microtrac, U.S.A.) according to the procedure of Dong et al. [Citation40].
2.2. Column experiments
An acrylic column with an inner diameter of 2.5 cm and a height of 16.7 cm was used in this study. Two layers of 0.1 mm diameter stainless steel film are arranged at the entrance and exit of the tower to disperse the flow. The peristaltic pump (BT100-3J, Longer Pump, China) is connected to the column inlet to control the flow rate (1 mL min-1). The cleaned quartz sand is then wet packed in the column. The fully filled column was cleaned with 10 well pore volumes (pv) of deionized water and 3 well volume (pv) of background solution to remove contaminants and achieve water chemical equilibrium, then pulsed with 2 pv MPs suspension. Therefore, the 3pv background solution was reintroduced into the column for thorough rinsing. The fraction collector (DBS160, Shanghai Huxi Analysis Instrument Factory Co., Ltd., China) was used to continuously collect the effluents from the outlet of the flow cell. The samples were collected at a 4-minute interval and the concentrations of all samples were determined using an UV-visible spectrophotometer (D8, FILA Instrument Co., China) at 970 nm. The software for conducting model is hydrus 1D. All experimental conditions are summarized in .
Table 1. Summary of experimental conditions and model parameters for all experiments.
2.3. DLVO theory
The Derjaguin-Landau-Verwey-Overbeek (DLVO) usually used to describe the interactions between each kind of MPs and porous media, which can be modeled as the integration of the Lifshitz-van der Waals (LW) attraction and the electrical double layer (EDL) repulsion between two particles. The Lifshitz-van der Waals attraction per unit area (ΦLW), the electrostatic double-layer repulsion per unit area (ΦEDL) and the total interaction energy per unit area (ΦTOT) can be calculated as follows [Citation41,Citation42]:
where A132 represents the Hamaker constant for substances ‘l’ (MPs) and ‘2’ (sand surface) in presence of medium ‘3’ (solution), which can be determined from the Hamaker constant of each material, r is the hydrodynamic radius of MPs, λ is the characteristic wavelength, h is the separation distance between the MPs, ε0 is the dielectric permittivity of vacuum, εr is the dielectric constant of water, κ is the Debye length, K is the Boltzmann constant, T is the absolute temperature, n is the valence of electrolyte, m is the electron charge [Citation41,Citation42].
All interaction energies are normalized by KT (product of the Boltzmann constant K and absolute temperature T) [Citation25].
2.4. Transport model
A numerical model considering one or second order kinetic deposition site was conducted for fitting the transport breakthrough curves (BTCs) of each kind of MPs in porous media under various IS conditions [Citation25]:
where θ is porosity, C is MPs concentration (M L−3), t is time (T), ρ is sand bulk density (M L−3), S is MPs deposition concentration, D is dispersion coefficient (L2 T−1), l is travel distance (L), v is Darcy velocity (L T−1), k is MPs deposition coefficient (T−1), Smax is the maximum MPs deposition concentration.
3. Results and discussion
3.1. Transport of PMMA MPs in porous media under various is conditions
The observation and simulation results of BTCs migration of PMMA MPs in porous media under different IS conditions () are shown in . The BTCs of PMMA MPs under 0.1 and 1 mM IS was slight before 1 PV, then quick increased to reach plateaus, and quick returned to baseline after flushing with background solution (). The BTCs for PMMA MPs under 10 mM IS showed salient features of ‘blocking’ effects during the transport process, declaring the typical time-varying migration process [Citation38]. also shows that the BTCs PMMA MPs significantly became lower with the increasing IS conditions, indicating the transport was decreased. Mass balance calculations demonstrated that the total mass recovery of PMMA MPs was dropped from 83.3% to 29.0% with the IS increased from 0.1 to 10 mM (). Due to containing carboxy groups, the PMMA MPs showed the high mobility in porous media, which was consistent with previous studies [Citation22,Citation43].
Figure 1. The BTCs of PMMA MPs transport in porous media under various is conditions (a) and the DLVO profiles between PMMA MPs and sand surface under various is conditions (b).

With the IS increased from 0.1 to 10 mM, zeta potentials of PMMA MPs increased from −37.5 to −32.2 mV (), as well as the zeta potentials of sand increased from −40.9 to −32.1 mV. The increasing IS may compress the electrical double layer and enhance the zeta potentials [Citation34]. The electrostatic repulsion between PMMA MPs and sand surface can be inhibited with the increasing zeta potentials, which reduced the deposition of PMMA MPs and improve their transport. Consistently, the hydrodynamic diameter of PMMA MPs has also increased from 2.83um to 3.90um and IS from 0.1 mM to 10 mM, which may result in enhanced retention of PMMA MPs and reduced transport ().
The effect of IS on the transport of PMMA MPs in porous media can also be explained by DLVO calculations (). The DLVO curve between PMMA MPs and sand body shows that when IS increases from 0.1 mM NaCl to 10 mM NaCl, the energy barrier decreases from 3.71 × 103 kT to 2.29 × 103 kT. At the same time, with the increase of IS to 10 mM, the secondary minimum of DLVO profile is gradually produced to the minimum of −5.20 kT. The secondary minimum under high IS condition may cause the tentative deposition of PMMA MPs onto sand surface in the long run [Citation44]. These results indicate that PMMA MPs are more favorable to retained in the porous media at high IS conditions, inhibiting the transport.
The model simulation in good agreement with the experimental BTCs of PMMA MPs under all IS conditions, and the coefficient of determination R2 is greater than 0.98 (). The transport process of PMMA MPs at 0.1 mM and 1 mM IS (columns 1 and 2) was simulated using the unit point kinetic expression of the first-order dynamical site, as BTCs showed a slightly mature state. Correspondingly, as the BTC of PMMA MPs under 10 mM IS showed typical blocking status, second-order kinetic site was invoked in the model for simulating PMMA MPs transport at 10 mM IS. The best-fit values of k for PMMA MPs transport ranged from 0.0064 to 0.0547 min−1 with the IS increased from 0.1 mM to 10 mM, as well as the fitted Smax was 0.626 mg g−1 for 10 mM IS (). The model results were consistent with the experimental results that the mobility of PMMA MPs decreased with the increasing IS conditions.
3.2. Transport of PVC MPs in porous media under various is conditions
The BTCs for PVC MPs showed symmetric appearance with the plateaus under 0.1 mM IS, however, remarkably changed to ‘blocking’ appearance under 1 mM IS, and approached to zero under 10 mM IS (). Mass balance calculations demonstrated that the total mass recovery of PVC MPs was dropped from 78.2% to 0.4% with the IS increased from 0.1 to 10 mM (), also indicating the reduced mobility with the increasing IS. Due to the strong polarity, PVC MPs also showed high mobility under 0.1 mM NaCl [Citation45]. However, compared to PMMA MPs, the reducing of PVC MPs mobility was more sensitive to the decreasing of NaCl concentrations. This result may be because of the presence of NaCl. Presumably, chlorine on PVC MPs may be more attractive to the sand surface under high Cl− concentrations, which may hinder transport.
Figure 2. The BTCs of PVC MPs transport in porous media under various is conditions (a) and the DLVO profiles between PVC MPs and sand surface under various is conditions (b).

With the IS increased from 0.1 to 10 mM, zeta potentials of PVC MPs increased from −40.3 to −36.1 mV (), as well as the hydrodynamic diameters of PVC MPs also increased from 2.92 to 3.82 um with the IS increased from 0.1 to 10 mM (). The decreased zeta potentials and increased hydrodynamic diameters may improve the retention and reduce the mobility of PVC MPs in the porous media, which was consistent to the transport tendency of PVC MPs. The energy barriers of the DLVO profiles between PVC MPs and sand also were decreased from 4.07 × 103 to 2.43 × 103 kT with the IS increased from 0.1 to 10 mM NaCl. The secondary minimum was −6.31 kT under 10 mM IS, implying the deposition in the long run ().
The fitted R2 of the BTCs of PVC MPs under the test IS conditions was also higher than 0.98 (). Under the condition of 0.1 mM IS, the first-order dynamic point IS used for MPs migration model of PVC, and under the condition of 1 mM IS, the second-order dynamic point is used for MPs migration model of PVC. The best-fit values of k for PVC MPs transport ranged from 0.0078 to 0.0920 min−1 with the increasing IS, which also showed the increased retention and decreased transport of PVC MPs. The fitted Smax was 0.396 mg g−1 for 1 mM IS ().
3.3. Transport of PP MPs in porous media under various is conditions
Same as the BTCs for PVC MPs (), the BTCs for PP MPs also showed symmetric appearance under 0.1 mM IS, the ‘blocking’ appearance under 1 mM IS, and almost disappeared under 10 mM IS (). Compared to PMMA MPs and PVC MPs, the PP MPs showed lower mobility under 0.1 mM IS condition, which may be due to their low polarity. With the IS increased from 0.1 to 10 mM, the transport of PP MPs also reduced with the total mass recovery dropped from 68.4% to 0.5% (). The isometric amplitude of mass recovery of PP MPs was smaller than the PVC MPs, but larger than the PMMA MPs. On the other hand, PP MPs would be aged and generate the hydroxyl functional groups, which may be benefit to the transport of PMMA MPs [Citation46].
Figure 3. The BTCs of PP MPs transport in porous media under various is conditions (a) and the DLVO profiles between PP MPs and sand surface under various is conditions (b).

Corresponded to the reducing mobility, the zeta potentials of PP MPs increased from −32.8 to −28.4 mV with the IS increased from 0.1 to 10 mM (), as well as the hydrodynamic diameters increased from 2.74 to 3.96 um (). The energy barriers of the DLVO profiles between PP MPs and sand were decreased from 3.12 × 103 to 1.84 × 103 kT with the IS increased from 0.1 to 10 mM NaCl. The secondary minimum was −7.10 kT under 10 mM IS ().
The fitted R2 of the BTCs of PP MPs under the test IS conditions was higher than 0.98 (), indicating the well matching. The first-order kinetic site was used to fit the BTCs of PP MPs under 0.1 mM IS conditions and the second-order kinetic site was applied to simulate the BTCs of PP MPs at 1 mM IS. The best-fit values of k for PVC MPs transport ranged from 0.0124 to 0.0432 min−1 with the increasing IS, corresponding to the experimental results. The fitted Smax was 0.3650 mg g−1 for 1 mM IS ().
3.4. Transport of PET MPs in porous media under various is conditions
All the BTCs for PET MPs showed symmetric appearance under the test IS conditions (), no ‘blocking’ appearance exhibited, which was coincident with the previous studies [Citation31]. Mass balance calculations demonstrated that the total mass recovery of PET MPs was dropped from 42.1% to 13.3% with the IS increased from 0.1 to 10 mM (). The PP MPs showed lowest mobility under 0.1 mM IS among four kinds of MPs. However, the transport reduced efficiency of PP MPs with the increasing IS was also relatively low, implying the resistance to IS changing. The high density of PET MPs (about 1.4 g cm−3) may increase the gravitational sedimentation of PET MPs onto sand surface [Citation47], which may contribute to the retention and inhibit the transport of PET MPs [Citation48].
Figure 4. The BTCs of PET MPs transport in porous media under various is conditions (a) and the DLVO profiles between PET MPs and sand surface under various is conditions (b).

Density has been recognized as a remarkable factor on colloid/nanoparticle deposition under both favorable and unfavorable chemical conditions [Citation38,Citation49].
With the IS increased from 0.1 to 10 mM, zeta potentials of PET MPs increased from −35.7 to −30.9 mV (), and the hydrodynamic diameters increased from 2.98 to 5.44 um with the IS increased from 0.1 to 10 mM (). The tendency of zeta potentials and hydrodynamic diameters demonstrated the decreasing transport of MPs with increasing IS, which was consistent with the DLVO results. The energy barriers of the DLVO profiles between PVC MPs and sand were decreased from 3.61 × 103 to 2.42 × 103 kT with the IS increased from 0.1 to 10 mM NaCl. The secondary minimum was −14.6 kT under 10 mM IS ().
The transport model also well conducted to simulate the transport of ET MPs under the test IS conditions with the R2 higher than 0.95 (). The first-order kinetic site was used to fit all the BTCs of PET MPs. Corresponded to the transport tendency, the best-fit values of k for PVC MPs transport ranged from 0.0295 to 0.0681 min−1 with the IS increased from 0.1 to 10 mM (). The relatively higher k under 0.1 mM IS also implied the lower mobility of PET MPs.
Several valuable laboratory studies, controlled by multiple physicochemical factors, have investigated the transport and fate of MPs in porous media. The results showed that the mobility of MPs was influenced by ionic strength (IS), cation type, dissolved organic matter (DOM) and surfactant [Citation21–30]. On the other hand, physical factors, such as concentration, flow rate, media grain size, moisture content, temperature also may influence the transport of MPs. The retention of microplastics in porous media is inhibited as their concentration increases, thus improving transport. Similar to other micro/nanoparticle particles, the increased flow rate usually facilitates the transport of MPs in porous media due to the clogging effect [Citation33]. According to the experimental results, PMMA MPs are more favorable to retained in the porous media at high IS conditions, inhibiting the transport. Chlorine on PVC MPs may be more attractive to the sand surface under high Cl− concentrations, which may hinder transport. The isometric amplitude of mass recovery of PP MPs was smaller than the PVC MPs, but larger than the PMMA MPs. On the other hand, PP MPs would be aged and generate the hydroxyl functional groups, which may be benefit to the transport of PMMA MPs [Citation46]. However, the transport reduced efficiency of PP MPs with the increasing IS was also relatively low, implying the resistance to IS changing. The high density of PET MPs (about 1.4 g cm−3) may increase the gravitational sedimentation of PET MPs onto sand surface [Citation47], which may contribute to the retention and inhibit the transport of PET MPs [Citation48]. Density has been recognized as a remarkable factor on colloid/nanoparticle deposition under both favorable and unfavorable chemical conditions.
4. Conclusion
Given MPs have been widely detected in soil and groundwater environment wordwide, understanding the transport of diversified MPs in porous media is important. In this study, mobility of four kinds of typical MPs under different IS conditions was investigated via column experiments, DLVO theory and transport model. The results indicated that various kinds of MPs showed similar transport tendency with the IS conditions changed, but various mobility under the same IS conditions. Commonly, the transport of all kinds of MPs in porous media was reduced with the increasing IS. However, with the improvement of IS conditions, the transport reducing efficiency of PMMA MPs and PET MPs was relatively lower than the PVC MPs and PP MPs, especially the PVC MPs. Presumably, the PVC MPs may be more attractive to the sand surface with the presence of Cl−, resulting in the sensibility of transport with the changed Cl− concentrations. Under low IS conditions (i.e. 0.1 mM IS), PET MPs showed lowest mobility due to their high density, indicating the effect of density in MPs transport in porous media under unfavorable deposition conditions. In addition, polarity also may influence the transport of MPs in porous media under similar IS conditions. In conclusion, hydrochemical conditions and basic properties will completely impact the transport behaviors of MPs in porous media. DLVO theory performed well to predict the interactions between all kinds of MPs and porous media, which also proved the experimental results. The transport model well fitted all the BTCs of MPs, implying the feasibility of traditional models in simulating diversified MPs transport. Given that soil and groundwater face significant MPs contamination, the findings of this study contribute to understanding the fate and transport of MPs in soil and groundwater, which is critical for assessing their environmental distribution and potential risks of these emerging pollutants. The results of this study have important implications for evaluating the distribution and risk of MPs in groundwater systems and soils.
Acknowledgments
This work was supported by the State Environmental Protection Key Laboratory of Soil Environmental Management and Pollution Control (SEMPC2023007), the Special Fund of State Environmental Protection Engineering Center for Urban Soil Contamination Control and Remediation (USCR-202205), and the National Natural Science Foundation of China (42277001).
Disclosure statement
No potential conflict of interest was reported by the author(s).
Additional information
Funding
References
- Henderson L, Green C. Making sense of microplastics? Public understandings of plastic pollution. Mar Pollut Bull. 2020;152:110908. doi: 10.1016/J.MARPOLBUL.2020.110908
- Tirkey A, Upadhyay LSB. Microplastics: an overview on separation, identification and characterization of microplastics. Mar Pollut Bull. 2021;170:112604. doi: 10.1016/j.marpolbul.2021.112604
- Zhang Y, Kang S, Allen S, et al. Atmospheric microplastics: a review on current status and perspectives. Earth-Sci Rev. 2020;203:103118. doi: 10.1016/j.earscirev.2020.103118
- Erostate M, Huneau F, Garel E, et al. Groundwater dependent ecosystems in coastal mediterranean regions: characterization, challenges and management for their protection. Water Res. 2020;172:115461. doi: 10.1016/j.watres.2019.115461
- Siebert S, Henrich V, Frenken K, et al. Update of the digital global map of irrigation areas to version 5. Rheinische Friedrich-Wilhelms-Universituy, Bonn, Germany and Food and Agriculture Organization of the United Nations, Rome, Italy. 2013.
- Zhang M, Liu L, Xu D, et al. Small-sized microplastics (< 500 μm) in roadside soils of Beijing, China: accumulation, stability, and human exposure risk. Environ Pollut. 2022;304:119121. doi: 10.1016/j.envpol.2022.119121
- Li R, Zhang S, Zhang L, et al. Field study of the microplastic pollution in sea snails (ellobium chinense) from mangrove forest and their relationships with microplastics in water/sediment located on the north of Beibu Gulf. Environ Pollut. 2020;263:114368. doi: 10.1016/j.envpol.2020.114368
- Zhou Y, Yang Y, Liu G, et al. Adsorption mechanism of cadmium on microplastics and their desorption behavior in sediment and gut environments: the roles of water pH, lead ions, natural organic matter and phenanthrene. Water Res. 2020;184:116209. doi: 10.1016/j.watres.2020.116209
- Jin Y, Lu L, Tu W, et al. Impacts of polystyrene microplastic on the gut barrier, microbiota and metabolism of mice. Sci Total Environ. 2019;649:308317. doi: 10.1016/j.scitotenv.2018.08.353
- Duan Z, Zhao S, Zhao L, et al. Microplastics in Yellow River Delta wetland: occurrence, characteristics, human influences, and marker. Environ Pollut. 2020;258:113232. doi: https://doi.org/10.1016/j.envpol.2019.113232
- Gorman D, Moreira FT, Turra A, et al. Organic contamination of beached plastic pellets in the South Atlantic: risk assessments can benefit by considering spatial gradients. Chemosphere. 2019;223:608–528. doi: 10.1016/j.chemosphere.2019.02.094
- Ye X, Wang P, Wu Y, et al. Microplastic acts as a vector for contaminants: the release behavior of dibutyl phthalate from polyvinyl chloride pipe fragments in water phase. Environ Sci Pollut Res. 2020;27(33):4208242091. doi: 10.1007/s11356-020-10136-0
- Cao Y, Lin H, Zhang K, et al. Microplastics: a major source of phthalate esters in aquatic environments. J Hazard Mater. 2022;432:128731. doi: 10.1016/j.jhazmat.2022.128731
- Deng H, Zhang Y, Li D, et al. Mangrove degradation retarded microplastics weathering and affected metabolic activities of microplastics-associated microbes. J Hazard Mater. 2023;445:130535. doi: 10.1016/j.jhazmat.2022.130535
- Kolandhasamy P, Su L, Li J, et al. Adherence of microplastics to soft tissue of mussels: a novel way to uptake microplastics beyond ingestion. Scie Total Environ. 2018;610–611:635–640. doi: 10.1016/j.scitotenv.2017.08.053
- Tang S, Lin L, Wang X, et al. Pb(ii) uptake onto nylon microplastics: interaction mechanism and adsorption performance. J Hazard Mater. 2020;386:121960. doi: 10.1016/j.jhazmat.2019.121960
- Samandra S, Johnston JM, Jaeger JE, et al. Microplastic contamination of an unconfined groundwater aquifer in Victoria, Australia. Scie Total Environ. 2022;802:149727. doi: 10.1016/j.scitotenv.2021.149727
- Lv W, Zhou W, Lu S, et al. Microplastic pollution in rice-fish co-culture system: a report of three farmland stations in Shanghai, China. Sci Total Environ. 2019;652:12091218. doi: 10.1016/j.scitotenv.2018.10.321
- Corradini F, Meza P, Eguiluz R, et al. Evidence of microplastic accumulation in agricultural soils from sewage sludge disposal. Sci Total Environ. 2019;671:411420. doi: 10.1016/j.scitotenv.2019.03.368
- Selvam S. Hazardous microplastic characteristics and its role as a vector of heavy metal in groundwater and surface water of coastal south India. J Hazard Mater. 2020;402:123786. doi: 10.1016/j.jhazmat.2020.123786
- Dong S, Xia J, Sheng L, et al. Transport characteristics of fragmental polyethylene glycol terephthalate (PET) microplastics in porous media under various chemical conditions. Chemosphere. 2021b;276:130214. doi: 10.1016/j.chemosphere.2021.130214
- Jiang Y, Yin X, Xi X, et al. Effect of surfactants on the transport of polyethylene and polypropylene microplastics in porous media. Water Res. 2021;196:117016. doi: 10.1016/j.watres.2021.117016
- Li J, Guo K, Cao Y, et al. Enhance in mobility of oxytetracycline in a sandy loamy soil caused by the presence of microplastics. Environ Pollut. 2021a;269:116151. doi: 10.1016/j.envpol.2020.116151
- Tong M, He L, Rong H, et al. Transport behaviors of plastic particles in saturated quartz sand without and with biochar/Fe3O4-biochar amendment. Water Res. 2020;169:115284. doi: 10.1016/j.watres.2019.115284
- Dong S, Zhou M, Su X, et al. Transport and retention patterns of fragmental microplastics in saturated and unsaturated porous media: a real-time pore-scale visualization. Water Res. 2022;214:118195. doi: 10.1016/j.watres.2022.118195
- He L, Rong H, Li M, et al. Bacteria have different effects on the transport behaviors of positively and negatively charged microplastics in porous media. J Hazard Mater. 2021;415:125550. doi: 10.1016/j.jhazmat.2021.125550
- Amila A, Fujio K, Yoshikazu M, et al. Rapid sampling of suspended and floating microplastics in challenging riverine and coastal Water environments in Japan. Water. 2020;12(7):1903.
- Andreas NA, Nicholas D, Vasileios AT. Water quality focusing on the hellenic world: from ancient to modern times and the future. Water. 2022;14(12):1887.
- Kellie B, Banu O. Microplastics and nanoplastics in the freshwater and terrestrial environment: a review. Water. 2020;12(9):2633.
- Liu F, Nadia BN, Kai B, et al. Microplastics removal from treated wastewater by a biofilter. Water[j]. 2020;12(4):1085. doi: 10.3390/w12041085
- Dong S, Cai W, Xia J, et al. Aggregation kinetics of fragmental PET nanoplastics in aqueous environment: complex roles of electrolytes, pH and humic acid. Environ Pollut. 2021;268:115828. doi: 10.1016/j.envpol.2020.115828
- Hierrezuelo J, Sadeghpour A, Szilagyi I, et al. Electrostatic stabilization of charged colloidal particles with adsorbed polyelectrolytes of opposite charge. Langmuir. 2010;26(19):1510915111. doi: 10.1021/la102912u
- Bradford SA, Kim HN, Haznedaroglu BZ, et al. Coupled factors influencing concentration-dependent colloid transport and retention in saturated porous media. Environ Sci Technol. 2009;43(18):69967002. doi: 10.1021/es900840d
- Alimi OS, Budarz JF, Hernandez LM, et al. Microplastics and nanoplastics in aquatic environments: aggregation, deposition, and enhanced contaminant transport. Environ Sci Technol. 2018;52(4):1704–1724. doi: 10.1021/acs.est.7b05559
- Mitzel MR, Sand S, Whalen JK, et al. Hydrophobicity of biofilm coatings influences the transport dynamics of polystyrene nanoparticles in biofilm-coated sand. Water Res. 2016;92:113120. doi: 10.1016/j.watres.2016.01.026
- Zhao P, Cui L, Zhao W, et al. Cotransport and deposition of colloidal polystyrene microplastic particles and tetracycline in porous media: the impact of ionic strength and cationic types. Sci Total Environ. 2020;753(142064):142064. doi: 10.1016/j.scitotenv.2020.142064
- Xiang Q, Zhu D, Chen QL, et al. Adsorbed sulfamethoxazole exacerbates the effects of polystyrene (∼2 μm) on gut microbiota and the antibiotic resistome of a soil Collembolan. Environ Sci Technol. 2019;53(21):12823–12834. doi: 10.1021/acs.est.9b04795
- Cai L, Zhu J, Hou Y, et al. Influence of gravity on transport and retention of representative engineered nanoparticles in quartz sand. J Contam Hydrol. 2015;181:153–160. doi: 10.1016/j.jconhyd.2015.02.005
- Wan J, Wilson JL. Colloid transport in unsaturated porous media. Water Resour Res. 1994;30(4):857864. doi: 10.1029/93WR03017
- Dong S, Gao B, Sun Y, et al. Visualization of graphene oxide transport in two-dimensional homogeneous and heterogeneous porous media. J Hazard Mater. 2019a;369:334e341. doi: 10.1016/j.jhazmat.2019.02.042
- Fritz G, Schädler V, Willenbacher N, et al. Electrosteric stabilization of colloidal dispersions. Langmuir[j]. 2002;18(16):6381–6390. doi: 10.1021/la015734j
- Petosa AR, Jaisi DP, Quevedo IR, et al. Aggregation and deposition of engineered nanomaterials in aquatic environments: role of physicochemical interactions. Environ Sci Technol. 2010;44(17):6532–6549. doi: 10.1021/es100598h
- Shaniv D, Dror I, Berkowitz B. Effects of particle size and surface chemistry on plastic nanoparticle transport in saturated natural porous media. Chemosphere. 2021;262:127854. doi: 10.1016/j.chemosphere.2020.127854
- Sun Y, Gao B, Bradford SA, et al. Transport, retention, and size perturbation of graphene oxide in saturated porous media: effects of input concentration and grain size. Water Res. 2015;68:24–33. doi: 10.1016/J.WATRES.2014.09.025
- Bourland LG. Multiphase PVC/styrenic copolymer alloys: internally reinforced by partial miscibility. J Vinyl Addit Technol. 1988;10(4):191–199. doi: 10.1002/vnl.730100407
- Li JF, Yang R, Yu J, et al. Natural photo-aging degradation of polypropylene nanocomposites. Polymer Degradation And Stability. 2008;93(1):84–89. doi: 10.1016/j.polymdegradstab.2007.10.022
- Horton AA, Dixon SJ. Microplastics: an introduction to environmental transport processes. Wiley Interdiscip Rev: Water. 2018;5(2):e1268. doi: 10.1002/wat2.1268
- Hedayati M, Sharma P, Katyal D, et al. Transport and retention of carbon-based engineered and natural nanoparticles through saturated porous media. J Nanopart Res. 2016;18(3):57. doi: 10.1007/s11051-016-3365-6
- Ma H, Pazmino EF, Johnson WP. Gravitational settling effects on unit cell predictions of colloidal retention in porous media in the absence of energy barriers. Environ Sci Technol. 2011;45(19):8306–8312. doi: 10.1021/es200696x