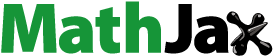
ABSTRACT
Enrofloxacin (ENR) is widely used in aquaculture due to its broad-spectrum activity against a large number of pathogenic bacteria. However, ENR exhibits strong adsorption and a low biodegradation rate in the environment. This study proposes a new method for degrading ENR, which combines dielectric barrier discharge (DBD) with advanced oxidation of peroxymonosulfate (PMS, HSO5−) and the use of nitrilotriacetic acid (NTA) combined with Fe(III) activator to improve reaction efficiency. The effects on the degradation efficiency were experimentally investigated by adjusting the internal process parameters and adding coexisting substances. The ESR and free radical scavenging experiments showed that both hydroxyl and sulfate radicals contributed to the degradation of the pollutant ENR. Based on the identified intermediates, and three possible degradation pathways were suggested. And the intermediates were less harmful. In conclusion, the Fe(III)-NTA/PMS/DBD system may be an efficient, environmentally friendly and new technology with development potential.
1. Introduction
Enrofloxacin (ENR) is a synthetically produced third-generation fluoroquinolone antibiotic. Due to its broad-spectrum activity against a wide range of pathogens, it is extensively used in aquaculture [Citation1]. However, ENR is not completely absorbed by animals, and its residues not only affect the growth of animals and plants but also easily lead to water and soil pollution. Currently, the average concentration of enrofloxacin detected in the main rivers, lakes, seas, and adjacent marine waters within China is 100 ng/L [Citation2]. Enrofloxacin not only can cause liver damage and a decline in immune system function in fish [Citation3], but its prolonged presence in soil and aquatic environments also impacts the structure and function of microbial communities [Citation4]. Moreover, due to their high adsorption, difficult biodegradation, and long half-life period [Citation5], it is challenging to be removed through natural processes. The accumulation of Enrofloxacin in the environment poses a threat to human health. Studies [Citation6] also have indicated that traditional activated sludge methods in sewage treatment plants cannot effectively remove it due to the high antimicrobial activity of antibiotics. Therefore, there is a need to seek an efficient method to remove ENR from water bodies.
Advanced Oxidation Processes (AOPs) are currently effective methods for removing antibiotics, such as Fenton [Citation7], ozone [Citation8], photocatalysis [Citation9], electrochemistry [Citation10], solar light/periodate [Citation11] and persulfate techniques [Citation12], all of which have strong oxidation capabilities [Citation13,Citation14]. Dielectric Barrier Discharge (DBD) is an emerging low-temperature plasma water treatment technology among AOPs. It integrates the advantages of advanced oxidation in treating organic substances. In addition to directly using reactive oxygen species (ROS) produced by the discharge effect, it can also degrade organic pollutants through the combination of chemically active substances, ultraviolet light, thermal effects, and shockwaves [Citation15]. DBD is mainly applied for the removal of organic pollutants [Citation16], heavy metals [Citation17] and inactivation and disinfection of pathogenic microorganisms [Citation18] in wastewater. However, compared to other advanced oxidation technologies, many energy effects produced in this technology, such as ultraviolet radiation and shockwaves, are not fully utilized, leading to low energy efficiency of the plasma [Citation19]. To improve the energy efficiency of this treatment technology and thereby enhance the degradation efficiency of organic pollutants, the combination of DBD with other advanced oxidation technologies has become an inevitable trend. Studies [Citation20,Citation21] have shown that the addition of some solid catalysts can improve the energy utilization of the plasma and the removal efficiency of pollutants, but there are also difficulties in separation in the solution and medium conduction. Persulfate advanced oxidation technology can avoid these problems, and peroxymonosulfate (PMS, HSO5−) can be activated to produce SO4·− with stronger oxidizing properties than ·OH [Citation22] and it can also react with H2O to produce ·OH [Citation23], thereby improving the energy utilization efficiency of the plasma. At the same time, the addition of transition metal Fe(III) will also enhance the ability of PMS to produce active substances, thereby jointly improving the system’s efficiency in removing organic pollutants [Citation24]. However, the addition of Fe(III) has disadvantages, such as the easy production of iron sludge [Citation25] and the extremely slow redox cycle of Fe(III)/Fe(II) [Citation26]. According to a report [Citation27], nitrilotriacetic acid (NTA), a common aminopolycarboxylate metal complexing agent, can solve the aforementioned issues, regulate the generation rate of hydroxyl radicals, and is easily biodegradable. As a result, it is widely used in the water treatment and soil remediation industries [Citation28]. This study constructs a Fe(III)-NTA/PMS/DBD oxidation system, investigates the degradation performance of this system on ENR, and proposes the degradation pathway of ENR through radical quenching tests and intermediate product detection.
2. Materials and methods
2.1. Materials
The analytical grade of NTA, KHSO5, FeCl3, ENR, humic acids(HA),NaHCO₃, NaCl, NaOH, HCl, Na2S2O3, C4H10O, C2H5OH, C6H4O2 were purchased from the China National Pharmaceutical Group Corporation. All solutions were prepared using ultrapure water (resistivity, σ > 18.25 MΩ·cm).
2.2. Experimental methods
was a schematic representation of the experimental apparatus, comprising primarily four components: a pulse power supply (Model CTP-2000K, Nanjing Suman Plasma Technology Co., Ltd., China), a contact voltage regulator (Model TDGC2–1, Zhejiang Chint Electrics Co., Ltd., China), a DBD reactor system (featuring a quartz glass dielectric with an outer diameter of 100 mm, a discharge area diameter of 55 mm, a discharge gap of 8 mm, dielectric layers on the upper and lower sides each with a thickness of 2 mm, an electrode gap of 10 mm, and a working volume of 15 mL), and an electrical detection system. This detection system encompassed an oscilloscope (Model TBS1102C, Tektronix, Inc., China), a current probe (Model TPP0100, Tektronix, Inc., China), and a voltage probe (Model TPP0100, Tektronix, Inc., China).
A Fe(III)-NTA solution was prepared by mixing an aqueous solution of FeCl3 with an NTA solution in a predefined molar ratio. The solution was shielded from light and allowed to stand for at least one hour to facilitate complete complexation. The experimental parameters were set to a peak voltage of 20 kV and a frequency of 50 Hz, with an electrical discharge duration of 40 minutes. Subsequently, a specified concentration of Fe(III)-NTA, PMS, and 50 mg/L ENR solution was introduced into a DBD reactor. Except for investigating the effect of initial pH value, the pH was not adjusted in all other experiments. Samples were collected at predetermined intervals. Upon completion of the reaction, it was quenched by adding 1 mL of 0.5 M Na2S2O3 solution. Finally, the water samples were filtered through a 0.22 μm membrane to analyze the aqueous contaminants and assess the water quality parameters.
2.3. Analytical methods
The concentration of ENR was determined using a UV-Vis spectrophotometer (UV-1800, Shanghai Mapada Instruments Co., Ltd., China) at a detection wavelength of 271 nm. After testing, the detection limit of ENR’s UV spectrophotometer was 0.1 mg/L. The zeta potential and particle size were measured using a Zetasizer Ultra (Malvern Panalytical Limited, UK) at pH levels of 2, 4, 6, 8, and 10 to ascertain the presence of electrostatic adsorption in the oxidation system. Total Organic Carbon (TOC) was quantified using a TOC analyzer (multi N/C 2100, Analytik Jena AG, Germany). The electron spin resonance (ESR) spectra were conducted on a ESR spectrometer (Bruker EMX-PLUS, Germany). 5,5-dimethyl-1-pyrroline N-oxide (DMPO) and 2,2,6,6-tetramethyl-4-piperidinol (TEMP) were used as spin trapping agents. The degradation products of ENR were analyzed using High-Performance Liquid Chromatography-Mass Spectrometry (HPLC-MS) coupled with Quadrupole Time-of-Flight Mass Spectrometry (1290uplc-qtof6550, Agilent, U.S.A.). The chromatographic column used was a C18–2.1 × 100 mm *1.7 μm (BEH, Waters, U.S.A.) with a column temperature of 40°C.
In this study, the Toxicity Estimation Software Tool (TEST, Version 5.1.2) was utilized to predict the toxicity of the degradation intermediates of ENR. The potential toxicological risks were assessed using several evaluation metrics, including the Bioconcentration Factor, developmental toxicity, mutagenicity, and the 48hour -Lethal Concentration 50(LC50) for Daphnia magna. These indices were employed to provide a comprehensive prediction of the potential toxicological hazards presented by the ENR degradation products.
3. Results and discussion
3.1. Comparison of oxidation process for ENR degradation
To identify the optimal system for the treatment of ENR, we conducted degradation experiments under various systems to determine the degradation efficiency of ENR. illustrated the relationship between the performance of the treatment system and the plasma discharge time.
Figure 2. Degradation of ENR in different systems. Experimental conditions: [ENR] = 50 mg/L; [PMS] = 0.3 g/L; the molar ratio of Fe(III)-NTA = 1:1;[Fe(III)-NTA] = 0.2 mM; peak voltage = 20 kV.
![Figure 2. Degradation of ENR in different systems. Experimental conditions: [ENR] = 50 mg/L; [PMS] = 0.3 g/L; the molar ratio of Fe(III)-NTA = 1:1;[Fe(III)-NTA] = 0.2 mM; peak voltage = 20 kV.](/cms/asset/b84bc6e7-e31c-4c23-9fd4-98adced4cc89/tcsb_a_2319250_f0002_oc.jpg)
In the process without DBD discharge, persulfates generated sulfate radicals (SO4·-) with high oxidative potential (Equation (1)). However, the degradation efficiency of persulfates alone was relatively low. Typically, transition metal ions such as Fe(III)/Fe(II) were added to disrupt the -O-O- bond in persulfates (Equation (2)) [Citation29,Citation30]. As shown in , the addition of Fe(III) resulted in an approximate 3% increase in the degradation efficiency of ENR within 40 minutes. Nevertheless, this system also faced issues of low degradation rates. The reason was that the regeneration rate of Fe(II) was significantly lower than the oxidation rate of Fe(III), while Fe(II) played a crucial role in the activation process [Citation31]. When the organic chelating agent NTA was added, it participated in the electron transfer oxidation reaction mediated by Fe(III), converting Fe(III) to Fe(II). The reason for this transformation was as follows: The complex formed by the chelator with Fe(III) had a lower redox potential than free Fe(III). A lower redox potential indicates stronger reducing properties. Therefore, the complex formed by the chelator with Fe(III) had a greater reducing capacity than free Fe(III). Thermodynamically, the complex formed by the chelator with Fe(III) was more easily reduced to Fe(II), thereby increasing the concentration of Fe(II) in the reaction and consequently enhancing the degradation of the pollutant ENR. This enhancement generated more free radicals in the system, thereby facilitating the degradation of target pollutants, as detailed in reactions (Equations (3–6)) [Citation32,Citation33]. As indicated in , the Fe(III)-NTA/PMS system increased the degradation rate by 10% within 40 minutes of treatment compared to the single PMS system.
During the single DBD discharge process, an increase in discharge voltage caused a breakdown of the air layer between the high-voltage electrode and the ground electrode. This transformed electrons receiving electrical energy into high-energy electrons. At the gas-liquid interface, O2, N2, and H2O underwent a series of physicochemical reactions with these high-energy electrons. These reactions produced a substantial amount of reactive species, ultraviolet radiation, and localized high temperatures [Citation34,Citation35] Additionally, the discharge process was accompanied by a certain intensity of ultraviolet light and thermal effects produced by the high-voltage discharge (Equations (7–11)). These chemical and physical reactions collectively attacked the organic pollutants in the solution. This led to the oxidative decomposition of the target pollutants, resulting in a 63% degradation rate of ENR within 40 minutes of experimental time [Citation36].
Upon integrating PMS during the plasma discharge process, radicals such as O3 and H2O2, generated by the plasma discharge, enhanced the oxidative capacity of the reaction system. These radicals, possessing higher oxidation-reduction potentials, contributed to this enhancement. For instance, the ·OH and SO4·− generated by PMS, along with the ·OH produced during the plasma discharge process, collaboratively degraded organic pollutants. SO4·− selectively degraded organic pollutants containing electron-donating groups, while ·OH non-selectively degraded those with electron-withdrawing groups. This effectively improved the treatment efficiency of organic pollutants [Citation37]. Concurrently, PMS was also activated by the thermal effects of the DBD. The e− and O2·− generated during the DBD discharge played a significant role in the formation of radicals within the DBD/PMS system [Citation24,Citation37] (Equations (12–18)). As illustrated in , the degradation rate of ENR reached 78% within 40 minutes of experimental time.
However, the addition of Fe(III), in the Fe(III)/PMS/DBD system, did not show a significant difference in degradation rate within 40 minutes of experimental time compared to the PMS/DBD system, which aligns with the analysis of the PMS and Fe(III)/PMS systems. In the Fe(III)-NTA/PMS/DBD system, the generated Fe(II)-NTA· not only reacts with PMS and H2O2 to produce ·OH and SO4·-, but also competes with ENR molecules for these radicals, reducing the consumption of ·OH and SO4·- [Citation24] (Equations (2, 18–21)).This promotes the system’s degradation efficiency for the target pollutant ENR, as shown in , where the final degradation rate of ENR reached 92% within 40 minutes.
In summary, without DBD, systems such as Fe(III), Fe(III)-NTA, Fe(III)/PMS, and Fe(III)-NTA/PMS were ineffective in substantially degrading ENR. The most effective among these, Fe(III)-NTA/PMS, achieved only a 29% degradation rate in 40 minutes. However, integrating the DBD system with the PMS oxidation process significantly enhanced ENR’s degradation efficiency. The Fe(III)-NTA/PMS/DBD system achieved a 92% degradation efficiency in 40 minutes. This indicates a synergistic effect between the DBD plasma and PMS in degrading ENR.Additionally, the degradation of ENR through different systems all follow pseudo-first-order kinetics (R2 >0.95), with the reaction rates ranked as follows: Fe(III)-NTA/PMS/DBD (0.06456 min−1) > DBD (0.02821 min−1) > Fe(III)-NTA/PMS (0.00925 min−1). showed the comparison of the degradation efficiency of this experimental system with other advanced oxidation techniques. This indicated that the synergistic system was a promising technology for the degradation of organic pollutants.
Table 1. A summary of plasma water treatment technology and other advanced oxidation technology applications.
HSO5−SO4·-
OH− (1)
Fe (II)HSO5−
SO4·-
OH−
Fe(III) (2)
Fe(III)NTA
Fe(II)
NTA· (3)
NTA·O2
NTA
O2·− (4)
Fe(III)NTA
O2·−
Fe(II)
NTA·
O2 (5)
Fe(II)NTA·
HSO5−
H+
Fe(III)
NTA
SO4·−
H2O (6)
*e−O2
2·O
e− (7)
O2·O
O3 (8)
·OH2O
2·OH (9)
2·OHH2O2 (10)
O3HO2·
HO·+O2
−O2· (11)
HSO5− SO4·−
OH− (12)
HOOSO3−e−
SO42-
HO· (13)
HOOSO3−e−
SO4·−
HO· (14)
-O3SOOSO3−
e−
SO4·−
SO42- (15)
HOOSO3−O2·−
SO42-
HO·−
O2 (16)
HOOSO3−O2·−
SO4·-
HO−
O2 (17)
-O3SOOSO3−
O2·−
SO4·-
SO42-
O2 (18)
Fe(II)NTA·
H2O2
Fe(III)
NTA
·OH
OH− (19)
Fe(II)NTA·
·OH
Fe(III)
NTA
OH− (20)
Fe(II)NTA·
SO4·−
Fe(III)
NTA
SO42- (21)
3.2. Effect of internal factors of the system
3.2.1. Effect of molar ratio of Fe(III)-NTA
showed the impact of the molar ratio of Fe(III)-NTA on the degradation of ENR. When the molar ratio increased from 1:0.5 to 1:1, the degradation rate of ENR rose from 87% to 92%, and the reaction rate increased from 0.05779 min−1 to 0.06456 min−1. However, further increasing the concentration of NTA reduced the efficiency. Specifically, as the Fe(III)-NTA molar ratio rose from 1:1 to 1:1.5 and 1:2, the degradation efficiency of ENR decreased from 92% to 90% and 87%, respectively. The trend’s potential reason is that typically, one NTA molecule binds with one Fe(II) or Fe(III) ion, forming a 1:1 Fe-NTA complex [Citation26]. Studies [Citation45,Citation46] suggest that more NTA could mean more complete Fe(III) complexation, but NTA also competes with target reactants for radicals. With increased NTA concentration, its scavenging effect on radicals becomes more pronounced, affecting the degradation efficiency of ENR.
3.2.2. Effect of Fe(III)-NTA dosages
A degradation experiment was conducted using the Fe(III)-NTA molar ratio of 1:1, which was selected for its optimal degradation efficiency. showed that as the dosage of Fe(III)-NTA increased from 0.02 mM to 0.2 mM, both the degradation efficiency and reaction rate of ENR progressively improved. The degradation rate rose from 86% (0.04221 min−1) to 92% (0.06456 min−1). However, increasing the Fe(III)-NTA dosage to 0.5 mM paradoxically decreased the ENR degradation efficiency to 89%. This decrease could be due to high doses of NTA inducing competitive reactions, excessively consuming active species like ·OH and SO4·− [Citation47]. Therefore, 0.2 mM Fe(III)-NTA was identified as the optimal dosage for this oxidation system.
3.2.3. Effect of PMS dosages
illustrated that the degradation effect of the Fe(III)-NTA/PMS/DBD system on ENR initially increased and then decreased with rising PMS dosage. When the concentration of PMS went up from 0.1 g/L to 0.3 g/L, ENR’s removal rate climbed from 86% to 92%. Yet, a further increase in PMS dosage (from 0.4 g/L to 0.5 g/L) led to no additional improvement in degradation efficiency, with the reaction rate dropping from 0.05706 min−1 to 0.05363 min−1. This trend could be explained by the fact that a moderate PMS concentration boost helped generate more reactive species, thereby aiding in ENR degradation. However, a higher PMS concentration might have turned PMS into a scavenger for SO4·−, reacting with SO4·- and ⋅OH to form SO5·− with a lower oxidation-reduction potential (E0 = 1.1 eV) than SO4·− [Citation48,Citation49] (Equations (22, 23)), thereby reducing the degradation efficiency of the system.
HSO5−SO4·−
SO5·−
SO42-
H+ (22)
HSO5−HO·
SO5·−
OH−
H+ (23)
3.2.4. Effect of initial ENR concentrations
showed how varying initial ENR concentrations affected the degradation efficiency. Higher efficiency was observed at lower ENR concentrations. At an initial concentration of 5 mg/L, the degradation rate reached approximately 91% in 20 minutes. For concentrations of 10 mg/L and 20 mg/L, the rate was about 90% after 30 minutes. At 50 mg/L, efficiency reached 92% by 40 minutes. As the initial ENR concentration rose, the time for degradation extended, and reaction rates dropped. The rates were 0.12789 min−1 for 5 mg/L, 0.09278 min−1 for 10 mg/L, 0.07553 min−1 for 20 mg/L, and 0.06456 min−1 for 50 mg/L. This pattern occurred because the total amount of reactive substances in the system was fixed. With higher initial ENR levels, the limited reactive substances couldn’t degrade all ENR molecules effectively, leading to slower degradation rates at higher ENR concentrations [Citation42].
3.3. Effect of initial pH value
After pH testing, the pH of the initial solution was 4.53. The initial low pH observed in the system can be attributed to the inherently acidic nature of the experimental reagents utilized in the system. showed that the degradation efficiency of ENR gradually decreased with increasing pH. This decline was due to the higher binding affinity of OH− to Fe than Fe to the chelating agent at higher pH levels, leading Fe(III)/Fe(II) to form hydroxides more readily [Citation50]. This transition reduced the concentration of Fe(III)/Fe(II) available for activating PMS, decreasing free radical concentration and thus lowering ENR degradation efficiency. Additionally, studies [Citation51] indicated that under alkaline conditions, SO4·− in the oxidation system was more likely to react with OH− and H2O (Equations (24, 25)), producing ·OH, which then became the dominant radical in the system. The generated ·OH (with an oxidation-reduction potential of E0 = 2.5–3.1 eV) had a lower potential than SO4·− (E0 = 2.8 eV) [Citation37]. Compared to ·OH, SO4·− was more selective and effective in electron transfer with unsaturated bonds in organic compounds and had a longer lifespan, potentially enhancing degradation efficiency. Moreover, DBD generated more reactive oxygen species (ROS) under acidic conditions, activating PMS to produce SO4·− [Citation52]. Thus, acidic conditions were more conducive to ENR removal.
Figure 4. (a)Effect of initial pH on ENR degradation, (b) Zeta potential of Fe(III)-NTA(III). Experimental conditions: [ENR] = 50 mg/L; the molar ratio of Fe(III)-NTA = 1:1; [Fe(iii)-NTA] = 0.2 mM; peak voltage = 20 kV.
![Figure 4. (a)Effect of initial pH on ENR degradation, (b) Zeta potential of Fe(III)-NTA(III). Experimental conditions: [ENR] = 50 mg/L; the molar ratio of Fe(III)-NTA = 1:1; [Fe(iii)-NTA] = 0.2 mM; peak voltage = 20 kV.](/cms/asset/d5efb771-c8f7-4dcc-a5cb-501b40412b15/tcsb_a_2319250_f0004_oc.jpg)
SO4·−OH−
SO42-
·OH (24)
SO4·−H2O
HSO4−
·OH (25)
The Zeta potential of the Fe(III)-NTA solution at different pH values was measured (), determining the point of zero charge (pHzpc) for the activator to be 3.31 (pHzpc = 3.31). When the pH was less than pHzpc, the activator carried a positive charge, making it more likely to adsorb anions from the solution. In contrast, at a pH greater than pHzpc, the activator had a negative charge, leading to electrostatic repulsion with anions. This resulted in varying pollutant degradation rates within the system under different pH conditions [Citation53]. Regarding PMS, it predominantly existed as HSO5− below a pH of 9.3 and as SO52- above it, showing its anionic nature over a wide pH range. For ENR, it was cationic when the pH was below its pKa1 (3.85) and anionic above its pKa2 (9.86) [Citation53]. Therefore, at an initial experimental pH of 3.5, with pHzpc (3.31) < pH < pKa (3.85) [Citation53], ENR was positively charged, Fe(III)-NTA negatively charged, and PMS existed as an anion. This facilitated the adsorption of ENR molecules onto the activator’s surface and PMS, thereby accelerating ENR’s degradation.
3.4. Effects of coexisting substances in surface water on ENR degradation
In practical applications, the composition of surface water bodies is complex, with co-existing substances like natural HA and inorganic anions impacting the stability of oxidants and altering the degradation products of target pollutants. This affected the generation of reactive oxygen species during oxidation, thereby influencing the degradation efficiency of organic pollutants [Citation54]. Consequently, this study investigated the impact of co-existing substances on the degradation of ENR by introducing varying concentrations of HA, Cl−, and CO32- into the oxidation system.
When 1 mg/L of HA was added, as depicted in , the degradation rate of ENR decreased from 92% to 89% within 40 minutes. Increasing HA concentrations to 5 mg/L, 10 mg/L, and 20 mg/L led to further declines in ENR degradation to 87%, 85%, and 82%, respectively. This indicated that the presence of HA inhibited the removal of ENR. HA, a polyelectrolyte macromolecule with functional groups like phenolic, carboxylic, hydroxyl, and carbonyl, tended to react with reactive species such as ·OH and SO4·-, acting as a radical scavenger and reducing degradation efficiency. Moreover, HA could affect the generation of ·OH from hydrogen peroxide, further impacting the system’s efficiency [Citation55,Citation56].
Figure 5. Effects of coexisting substance concentrations and free radical quenchers in water on ENR degradation. (a) HA concentration, (b) Cl− concentration, (c) CO32- concentration. Experimental conditions: [ENR] = 50 mg/L; [PMS] = 0.3 g/L; the molar ratio of Fe(III)-NTA = 1:1; [Fe(iii)-NTA] = 0.2 mM; peak voltage = 20 kV.
![Figure 5. Effects of coexisting substance concentrations and free radical quenchers in water on ENR degradation. (a) HA concentration, (b) Cl− concentration, (c) CO32- concentration. Experimental conditions: [ENR] = 50 mg/L; [PMS] = 0.3 g/L; the molar ratio of Fe(III)-NTA = 1:1; [Fe(iii)-NTA] = 0.2 mM; peak voltage = 20 kV.](/cms/asset/5cb66c18-0b45-474f-ab39-966bceb3ea4d/tcsb_a_2319250_f0005_oc.jpg)
showed that adding Cl− significantly inhibited ENR degradation, with higher Cl− concentrations worsening this effect. At 100 mM Cl−, ENR degradation efficiency fell to 56%. Cl−reacted with radicals like ·OH and SO4·- in the system, forming radicals such as Cl·、Cl2·−、ClOH·−、HClO and ClO3− with lower oxidative capacities than ·OH and SO4·- This was because of their lower oxidation-reduction potentials E0(Cl2·-/Cl·) = 2.41 V, E0(Cl2/Cl−) = 1.36 V,E0(HOCl/Cl−) = 1.48 V compared to ·OH and SO4·-(E0 = 2.5 V–3.1 V) [Citation28,Citation57–59]. As a result, ENR degradation efficiency decreased with increasing Cl− concentration.
The addition of CO32- notably reduced the degradation efficiency of ENR; efficiency dropped from 92% to 52% as CO32- concentration increased from 0 mM to 100 mM. This occurred because CO32- in the system reacted with radicals like OH and SO4·− to form CO3·− (E0 = 1.78 V) and HCO3·, which had significantly lower oxidation-reduction potentials than ·OH and SO4·−. CO3·− and HCO3· [Citation59,Citation60], being electrophilic radicals with higher selectivity towards organics, led to a reduced oxidative capacity and diminished degradation efficiency of ENR.
3.5. Role of active species and mineralization removal
The radicals produced by this system in the degradation process and the degree of contribution of each radical were determined by adding different concentrations of ESR spectra and free radical scavenger analysis. In the ), DMPO-·O2− produced a distinct 1:1:1:1 characteristic peak, and a distinct 1:1:1 characteristic peak was observed for TEMP-1O2. A 1:2:2:1 signaling characteristic peak was observed for DMPO-·OH and a 1:2:2:1 signaling characteristic peak was observed for DMPO-SO4·− of six characteristic peaks. To investigate the contribution of radicals to ENR degradation, tert-butanol (TBA, ·OH radical scavengers, 10 mM), ethanol (EtOH, ·OH and SO4·− radical scavengers, 10 mM), benzoquinone (BQ, ·O2− radical scavengers, 10 mM) were added to quench radicals during the reaction. As shown in , the addition of tert-butanol, ethanol, and benzoquinone reduced ENR removal efficiency to 45%, 19%, and 63%, respectively. Previously, it was indicated that ·OH, SO4·−, and O2− all participated in ENR degradation, with the extent of inhibition from scavengers in descending order: EtOH>TBA>BQ, suggesting the contribution order to ENR removal was ·OH radical > SO4·− radical > ·O2− radical.
Figure 6. (a, b) ESR spectra showing DMPO and TEMO adducts formed with SO4·−, ·OH, ·O2− and1O2, (c) the effect of free radical scavengers on ENR degradation, (d) removal rate of ENR and TOC. Experimental conditions: [ENR] = 50 mg/L; [PMS] = 0.3 g/L; the molar ratio of Fe(III)-NTA = 1:1; [Fe(iii)-NTA] = 0.2 mM; peak voltage = 20 kV.
![Figure 6. (a, b) ESR spectra showing DMPO and TEMO adducts formed with SO4·−, ·OH, ·O2− and1O2, (c) the effect of free radical scavengers on ENR degradation, (d) removal rate of ENR and TOC. Experimental conditions: [ENR] = 50 mg/L; [PMS] = 0.3 g/L; the molar ratio of Fe(III)-NTA = 1:1; [Fe(iii)-NTA] = 0.2 mM; peak voltage = 20 kV.](/cms/asset/73bd8138-23bd-40e5-ba49-b60ca162945d/tcsb_a_2319250_f0006_oc.jpg)
Furthermore, by monitoring TOC changes during ENR degradation (), it was observed that after 40 minutes of reaction, the removal rate of ENR reached 91%, but the TOC removal rate was only 49%. It was indicated that while ENR was removed, mineralization was relatively low, suggesting incomplete degradation by this oxidation system. Therefore, it was essential to analyze the main degradation products of ENR.
3.6. Proposed pathways for ENR degradation
HPLC-MS was employed to analyze the intermediate products formed during the experimental process. The principal intermediates are presented in . Based on these findings, three possible pathways were proposed, as illustrated in .According to the studies [Citation61,Citation62], Pathway 1 involved ENR undergoing defluorination to form P1 (C19H23N3O3, m/z = 340), which then opened the benzene ring and undergoes hydroxylation to yield P2 (C20H27N3O5, m/z = 390). Further oxidation led to side-chain cleavage, destroying the ENR structure and forming P3 (C6H14N2, m/z = 115). Due to the use of FeCl3 as an activator and the potential participation of free Cl− in water, Pathways 2 and 3 were proposed. In Pathway 2, under radical influence, the piperazine group opened, leading to C-N bond cleavage and forming intermediate A (C20H24FN3O4), which was not detected in this experiment but was reported in other studies [Citation63]. This intermediate could potentially have been formed under UV light produced by the plasma used. Further radical-induced C-F and C-C cleavage and oxidation formed P4 (C19H21N3O5, m/z = 369) or opened the piperazine ring in A, substituting Cl for F to yield P5 (C19H20ClN3O5, m/z = 405). Pathway 3, as reported in the paper [Citation64], indicates that during the degradation of ENR using UV/Fe(II)/H2O2, ciprofloxacin (Intermediate B, C17H18FN3O3) is formed through OH substitution and subsequent decarboxylation. However, it was not detected in this experiment, likely due to its low concentration. Subsequently, Cl replaces F and -OH in ciprofloxacin, resulting in the formation of P6 (C16H17Cl2N3O, m/z = 316). Additionally, the C-N bond between the piperazine group and the aromatic ring breaks, leading to the formation of P7 (C13H10FNO3, m/z = 248). Although Intermediate C, formed by Cl substitution on the carboxyl group of P7 [Citation65], was not detected in this study, it has been reported in similar degradation processes of fluoroquinolone antibiotics like fleroxacin under Cl and ClO2 conditions [Citation65]. Finally, under oxidative conditions, Intermediate C (C12H9ClFNO) undergoes cyclopropane ring cleavage and defluorination, resulting in the formation of P8 (C9H6ClNO, m/z = 180). Under the influence of radicals, these products further mineralize into substances such as H2O and CO2.
Table 2. M/Z, chemical mechanism of degradation products produced in the reaction process.
3.7. Toxicity of transformation products
The T.E.S.T. software was used to calculate and predict the biotoxicity of ENR and its potential intermediates, employing bioaccumulation factors, developmental toxicity, mutagenicity induction, and Daphnia magna 48 h-LC50 as assessment indicators. As shown in revealed that most intermediates exhibited weaker bioaccumulation than ENR, except for P6 and P7, which had higher bioaccumulation potential, particularly P6 with stronger bioaccumulation. However, the overall intermediates showed reduced bioaccumulation capacity. According to , ENR was classified as ‘toxic’ in terms of developmental toxicity, whereas most degradation intermediates, except for P1, P4, P5, and P7, exhibited lower toxicity compared to ENR. Most intermediates were positively mutagenic (), albeit less toxic than ENR, indicating even low-accumulating products like P1, P4, and P5 posed environmental risks post-treatment. The 48 h-LC50 for Daphnia magna indicated acute toxicity, and as shown in , only P6 exhibited higher acute toxicity than ENR. Overall, most degradation intermediates were less toxic than ENR, but the bioaccumulation and acute toxicity of P6 were higher and warranted attention.
4. Conclusions
The Fe(III)-NTA/PMS/DBD oxidation system constructed in this study can effectively and stably remove ENR, and this study can draw the following conclusions:
Compared to other systems, the Fe(III)-NTA/PMS/DBD system increased the degradation rate of ENR to 92%. The optimal conditions obtained in the experiments were: Fe(III)-NTA molar ratio of 1:1, Fe(III)-NTA concentration of 0.2 mM, PMS concentration of 0.3 g/L, PMS concentration of 50 mg/L, and initial solution pH of 3.5.
Coexisting substances in surface water, such as HA, Cl−, and CO32-, all had inhibitory effects on the degradation of ENR, with CO32- having the most significant inhibitory effect.
Free radicals ·OH, SO4·−, and ·O2− all participated in the degradation of ENR, with their contribution rates being in the order of OH > SO4·−>·O2−.
HPLC-MS identified the products formed during the degradation of ENR, indicating three potential degradation pathways: ① Defluorination; ② Pyrazole ring opening; ③ Hydroxyl substitution. The toxicity analysis shows that, the degradation products are difficult to be bio-accumulate and have lower developmental toxicity, mutagenicity, and exhibit weaker acute toxicity.
Disclosure statement
No potential conflict of interest was reported by the author(s).
References
- Shao B, Don H, Feng L, et al. Influence of sulfite/Fe(VI) molar ratio on the active oxidants generation in Fe(VI)/sulfite process. J Hazard Mater. 2020;384:121303. doi: 10.1016/j.jhazmat.2019.121303
- Orimolade BO, Oladipo AO, Idris AO, et al. Advancements in electrochemical technologies for the removal of fluoroquinolone antibiotics in wastewater: a review. Sci Total Environ. 2023;881:163522. doi: 10.1016/j.scitotenv.2023.163522
- Rico A, Satapornvanit K, Haque MM, et al. Use of chemicals and biological products in Asian aquaculture and their potential environmental risks: a critical review. Rev Aquacult. 2012;4(2):75–166. doi: 10.1111/j.1753-5131.2012.01062.x
- Thiele-Bruhn S, Beck IC. Effects of sulfonamide and tetracycline antibiotics on soil microbial activity and microbial biomass. Chemosphere. 2005;59(4):457–465. doi: 10.1016/j.chemosphere.2005.01.023
- Appelbaum PC, Hunter PA. The fluoroquinolone antibacterials: past, present and future perspectives. Int J Antimicrob Agents. 2000;16(1):5–15. doi: 10.1016/S0924-8579(00)00192-8
- Reemtsma T, Weiss S, Mueller J, et al. Polar pollutants entry into the water cycle by municipal wastewater: a European perspective. Environ Sci Technol. 2006;40(17):5451–5458. doi: 10.1021/es060908a
- Shah NS, Rizwan AD, Khan JA, et al. Toxicities, kinetics and degradation pathways investigation of ciprofloxacin degradation using iron-mediated H2O2 based advanced oxidation processes. Process Saf Environ Prot. 2018;117:473–482. doi: 10.1016/j.psep.2018.05.020
- Wang J, Liu H, Ma D, et al. Degradation of organic pollutants by ultraviolet/ozone in high salinity condition: non-radical pathway dominated by singlet oxygen. Chemosphere. 2021;268:128796. doi: 10.1016/j.chemosphere.2020.128796
- Topkaya E, Konyar M, Yatmaz HC, et al. Pure ZnO and composite ZnO/TiO2 catalyst plates: a comparative study for the degradation of azo dye, pesticide and antibiotic in aqueous solutions. J Colloid Interface Sci. 2014;430:6–11. doi: 10.1016/j.jcis.2014.05.022
- Dai J, Feng H, Shi K, et al. Electrochemical degradation of antibiotic enoxacin using a novel PbO2 electrode with a graphene nanoplatelets inter-layer: characteristics, efficiency and mechanism. Chemosphere. 2022;307:135833. doi: 10.1016/j.chemosphere.2022.135833
- Huang Y, Xie Q, Wang H, et al. Degradation of trimethoprim in the simulated solar light/periodate system: process and mechanism analysis. Water Proc Eng. 2024;57:104726. doi: 10.1016/j.jwpe.2023.104726
- Furman OS, Teel AL, Watts RJ. Mechanism of base activation of persulfate. Environ Sci Technol. 2010;44(16):6423–6428. doi: 10.1021/es1013714
- Wang H, Mustafa M, Yu G, et al. Oxidation of emerging biocides and antibiotics in wastewater by ozonation and the electro-peroxone process. Chemosphere. 2019;235:575–585. doi: 10.1016/j.chemosphere.2019.06.205
- Malato S, Fernández-Ibáñez P, Maldonado MI, et al. Decontamination and disinfection of water by solar photocatalysis: recent overview and trends. Catal Today. 2009;147(1):1–59. doi: 10.1016/j.cattod.2009.06.018
- Guo H, Jiang N, Wang H, et al. Enhanced catalytic performance of graphene-TiO2 nanocomposites for synergetic degradation of fluoroquinolone antibiotic in pulsed discharge plasma system. Appl Catal B-Environ. 2019;248:552–566. doi: 10.1016/j.apcatb.2019.01.052
- Dong B, Li Z, Wang P, et al. Dielectric barrier discharge plasma-coupled rare-earth modified Er3±BiOI catalytic materials for degradation of organic pollutant benzohydroxamic acid in mineral beneficiation waster: performance, degradation pathway, and its mechanism. Water Proc Eng. 2023;56:104393. doi: 10.1016/j.jwpe.2023.104393
- Cao Z, Zhao L, Sun Y, et al. Simultaneous removal of enrofloxacin and Cr(VI) using uniaxial wet wall dielectric barrier discharge plasma. J Clean Prod. 2022;379:134800. doi: 10.1016/j.jclepro.2022.134800
- Wu Y, Cheng J-H, Keener KM, et al. Inhibitory effects of dielectric barrier discharge cold plasma on pathogenic enzymes and anthracnose for mango postharvest preservation. Postharvest Biol Technol. 2023;196:112181. doi: 10.1016/j.postharvbio.2022.112181
- Crema APS, Borges LDP, Micke GA, et al. Degradation of indigo carmine in water induced by non-thermal plasma, ozone and hydrogen peroxide: a comparative study and by-product identification. Chemosphere. 2020;244:125502. doi: 10.1016/j.chemosphere.2019.125502
- Xin L, Sun Y, Feng J. Ag3PO4/TiO2-assisted degradation of malachite green in aqueous solution treated by dielectric barrier discharge plasma. J Chem Technol Biot. 2016;91(7):2131–2142. doi: 10.1002/jctb.4840
- Cao Y, Qu G, Li T, et al. Review on reactive species in water treatment using electrical discharge plasma: formation, measurement, mechanisms and mass transfer. Plasma Sci Technol. 2018;20(10):103001. doi: 10.1088/2058-6272/aacff4
- Li X, Jie B, Lin H, et al. Application of sulfate radicals-based advanced oxidation technology in degradation of trace organic contaminants (TrOcs): recent advances and prospects. J Environ Manage. 2022;308:114664. doi: 10.1016/j.jenvman.2022.114664
- Yaoyao J, Xiaoning W, Sheng-Peng S, et al. Hydroxyl and sulfate radicals formation in UVA/FeIII-NTA/S2O82- system: mechanism and effectiveness in carbamazepine degradation at initial neutral pH. Chem Eng J. 2019;368:541–552. doi: 10.1016/j.cej.2019.02.182
- Shang K, Li W, Wang X, et al. Degradation of p-nitrophenol by DBD plasma/Fe2+/persulfate oxidation process. Sep Purif Technol. 2019;218:106–112. doi: 10.1016/j.seppur.2019.02.046
- Dao YH, De Laat J. Hydroxyl radical involvement in the decomposition of hydrogen peroxide by ferrous and ferric-nitrilotriacetate complexes at neutral pH. Water Res. 2011;45(11):3309–3317. doi: 10.1016/j.watres.2011.03.043
- Jin Y, Sun S-P, Yang X, et al. Degradation of ibuprofen in water by FeII-NTA complex-activated persulfate with hydroxylamine at neutral pH. Chem Eng J. 2018;337:152–160. doi: 10.1016/j.cej.2017.12.094
- Abida O, Mailhot G, Litter M, et al. Impact of iron-complex (Fe(iii)–NTA) on photoinduced degradation of 4-chlorophenol in aqueous solution. Photochem Photobiol Sci. 2006;5(4):395–402. doi: 10.1039/b518211e
- Yang Y, Pignatello JJ, Ma J, et al. Comparison of halide impacts on the efficiency of contaminant degradation by sulfate and hydroxyl radical-based advanced oxidation processes (AOPs). Environ Sci Technol. 2014;48(4):2344–2351. doi: 10.1021/es404118q
- George C, El Rassy H, Chovelon JM. Reactivity of selected volatile organic compounds (VOCs) toward the sulfate radical (SO4−). Int J Chem Kinet. 2001;33(9):539–547. doi: 10.1002/kin.1049
- Scire S, Minico S, Crisafulli C, et al. Catalytic combustion of volatile organic compounds on gold/cerium oxide catalysts. Appl Catal B-Environ. 2003;40(1):43–49. doi: 10.1016/S0926-3373(02)00127-3
- Yuan D, Zhang C, Tang S, et al. Enhancing CaO2 fenton-like process by Fe(II)-oxalic acid complexation for organic wastewater treatment. Water Res. 2019;163:114861. doi: 10.1016/j.watres.2019.114861
- Liang C, Liang C-P, Chen C-C. PH dependence of persulfate activation by EDTA/Fe(III) for degradation of trichloroethylene. J Contam Hydrol. 2009;106(3–4):173–182. doi: 10.1016/j.jconhyd.2009.02.008
- De Luca A, Dantas RF, Esplugas S. Assessment of iron chelates efficiency for photo-Fenton at neutral pH. Water Res. 2014;61:232–242. doi: 10.1016/j.watres.2014.05.033
- Kanazawa S, Kawano H, Watanabe S, et al. Observation of OH radicals produced by pulsed discharges on the surface of a liquid. Plasma Sources Sci Technol. 2011;20(3):034010. doi: 10.1088/0963-0252/20/3/034010
- Locke BR, Sato M, Sunka P, et al. Electrohydraulic discharge and nonthermal plasma for water treatment. Industrial Engineering Chemistry Research. 2006;45(3):882–905. doi: 10.1021/ie050981u
- Antoniou MG, de la Cruz AA, Dionysiou DD. Degradation of microcystin-LR using sulfate radicals generated through photolysis, thermolysis and e− transfer mechanisms. Applied Catalysis B: Environmental. 2010;96(3–4):290–298. doi: 10.1016/j.apcatb.2010.02.013
- Wu J, Xiong Q, Liang J, et al. Degradation of benzotriazole by DBD plasma and peroxymonosulfate: mechanism, degradation pathway and potential toxicity. Chem Eng J. 2020;384:123300. doi: 10.1016/j.cej.2019.123300
- Mao S, Zhao P, Wu Y, et al. Promoting charge migration of Co(OH)2/g-C3N4 by hydroxylation for improved PMS activation: catalyst design, DFT calculation and mechanism analysis. Chem Eng J. 2023;451:138503. doi: 10.1016/j.cej.2022.138503
- Yi C, Yang L, Yi R, et al. Degradation of the nonylphenol aqueous solution by strong ionization discharge: evaluation of degradation mechanism and the water toxicity of zebrafish. Water Sci Technol. 2022;86(2):227–243. doi: 10.2166/wst.2022.175
- Zhao H, Yi C, Yi R, et al. Research on the degradation mechanism of dimethyl phthalate in drinking water by strong ionization discharge. Plasma Sci Technol. 2018;20:035503. doi: 10.1088/2058-6272/aa97d1
- Nawaz MI, Yi C, Zafar AM, et al. Efficient degradation and mineralization of aniline in aqueous solution by new dielectric barrier discharge non-thermal plasma. Environ Res. 2023;237:117015. doi: 10.1016/j.envres.2023.117015
- Nawaz MI, Yi C, Zhao H, et al. Experimental study of nitrobenzene degradation in water by strong ionization dielectric barrier discharge. Environ Technol. 2021;42(5):789–800. doi: 10.1080/09593330.2019.1645740
- Nawaz MI, Yi C, Asilevi PJ, et al. A Study of the performance of dielectric barrier discharge under different conditions for nitrobenzene degradation. Water. 2019;11(4):842. doi: 10.3390/w11040842
- Wang J, Sun Y, Feng J, et al. Degradation of triclocarban in water by dielectric barrier discharge plasma combined with TiO2/activated carbon fibers: effect of operating parameters and byproducts identification. Chem Eng J. 2016;300:36–46. doi: 10.1016/j.cej.2016.04.041
- Rastogi A, Ai-Abed SR, Dionysiou DD. Sulfate radical-based ferrous–peroxymonosulfate oxidative system for PCBs degradation in aqueous and sediment systems. Appl Catal B-Environ. 2009;85(3–4):171–179. doi: 10.1016/j.apcatb.2008.07.010
- Huang W, Brigante M, Wu F, et al. Effect of ethylenediamine-N,N′-disuccinic acid on Fenton and photo-Fenton processes using goethite as an iron source: optimization of parameters for bisphenol a degradation. Environ Sci Pollut Res. 2013;20(1):39–50. doi: 10.1007/s11356-012-1042-6
- Xiong L, Ren W, Lin H, et al. Efficient removal of bisphenol a with activation of peroxydisulfate via electrochemically assisted Fe(III)-nitrilotriacetic acid system under neutral condition. J Hazard Mater. 2021;403:123874. doi: 10.1016/j.jhazmat.2020.123874
- Buxton GV, Greenstock CL, Helman WP, et al. Critical review of rate constants for reactions of hydrated electrons, hydrogen atoms and hydroxyl radicals (⋅OH/⋅O) in aqueous solution. J Phys Chem Ref Data. 1988;17(2):513–886. doi: 10.1063/1.555805
- Shang K, Wang X, Li J, et al. Synergetic degradation of acid orange 7 (AO7) dye by DBD plasma and persulfate. Chem Eng J. 2017;311:378–384. doi: 10.1016/j.cej.2016.11.103
- Maezono T, Tokumura M, Sekine M, et al. Hydroxyl radical concentration profile in photo-Fenton oxidation process: generation and consumption of hydroxyl radicals during the discoloration of azo-dye orange II. Chemosphere. 2011;82(10):1422–1430. doi: 10.1016/j.chemosphere.2010.11.052
- Lou J, Lu G, Wei Y, et al. Enhanced degradation of residual potassium ethyl xanthate in mineral separation wastewater by dielectric barrier discharge plasma and peroxymonosulfate. Separation and Purification Technology. 2022;282:119955. doi: 10.1016/j.seppur.2021.119955
- Jiang B, Zheng J, Liu Q, et al. Degradation of azo dye using non-thermal plasma advanced oxidation process in a circulatory airtight reactor system. Chem Eng J. 2012;204:32–39. doi: 10.1016/j.cej.2012.07.088
- Li J, Cheng X, Zhang H, et al. Insights into performance and mechanism of ZnO/CuCo2O4 composite as heterogeneous photoactivator of peroxymonosulfate for enrofloxacin degradation. J Hazard Mater. 2023;448:130946. doi: 10.1016/j.jhazmat.2023.130946
- Wang JL, Wang SZ. Effect of inorganic anions on the performance of advanced oxidation processes for degradation of organic contaminants. Chem Eng J. 2021;411:128392. doi: 10.1016/j.cej.2020.128392
- Jiang C, Ji Y, Shi Y, et al. Sulfate radical-based oxidation of fluoroquinolone antibiotics: kinetics, mechanisms and effects of natural water matrices. Water Res. 2016;106:507–517. doi: 10.1016/j.watres.2016.10.025
- Pan X, Yan L, Qu R, et al. Degradation of the UV-filter benzophenone-3 in aqueous solution using persulfate activated by heat, metal ions and light. Chemosphere. 2018;196:95–104. doi: 10.1016/j.chemosphere.2017.12.152
- Yuan R, Ramjaun SN, Wang Z, et al. Effects of chloride ion on degradation of acid orange 7 by sulfate radical-based advanced oxidation process: implications for formation of chlorinated aromatic compounds. J Hazard Mater. 2011;196:173–179. doi: 10.1016/j.jhazmat.2011.09.007
- Wang Z, Yuan R, Guo Y, et al. Effects of chloride ions on bleaching of azo dyes by Co2+/oxone regent: kinetic analysis. J Hazard Mater. 2011;190(1–3):1083–1087. doi: 10.1016/j.jhazmat.2011.04.016
- Zhang RC, Sun PZ, Boyer TH, et al. Degradation of pharmaceuticals and metabolite in synthetic human urine by UV, UV/H2O2, and UV/PDS. Environ Sci Technol. 2015;49(5):3056–3066. doi: 10.1021/es504799n
- Zhang G, He X, Nadagouda MN, et al. The effect of basic pH and carbonate ion on the mechanism of photocatalytic destruction of cylindrospermopsin. Water Res. 2015;73:353–361. doi: 10.1016/j.watres.2015.01.011
- He C, Xia W, Zhou C, et al. Rational design to manganese and oxygen co-doped polymeric carbon nitride for efficient nonradical activation of peroxymonosulfate and the mechanism insight. Chem Eng J. 2022;430:132751. doi: 10.1016/j.cej.2021.132751
- Yang B, Kookana RS, Williams M, et al. Oxidation of ciprofloxacin and enrofloxacin by ferrate(VI): products identification, and toxicity evaluation. J Hazard Mater. 2016;320:296–303. doi: 10.1016/j.jhazmat.2016.08.040
- Li Y, Niu J, Wang W. Photolysis of enrofloxacin in aqueous systems under simulated sunlight irradiation: kinetics, mechanism and toxicity of photolysis products. Chemosphere. 2011;85(5):892–897. doi: 10.1016/j.chemosphere.2011.07.008
- Qiu W, Zheng M, Sun J, et al. Photolysis of enrofloxacin, pefloxacin and sulfaquinoxaline in aqueous solution by UV/H2O2, UV/Fe(II), and UV/H2O2/Fe(II) and the toxicity of the final reaction solutions on zebrafish embryos. Sci Total Environ. 2019;651:1457–1468. doi: 10.1016/j.scitotenv.2018.09.315
- He G, Zhang T, Zheng F, et al. Reaction of fleroxacin with chlorine and chlorine dioxide in drinking water distribution systems: kinetics, transformation mechanisms and toxicity evaluations. Chem Eng J. 2019;374:1191–1203. doi: 10.1016/j.cej.2019.06.022