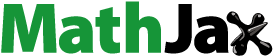
ABSTRACT
This study was conducted to identify the ability of a modern developed amino-acid modified montmorillonite clay (AA-Mont) to adsorb Cu2+, Pb2+ and Ni2+ cations from a multi-aqueous wastewater. This experimental was conducted to identify the potential of clay-based adsorbents to remediate pollutants from the PRA river basin. Experimental wastewater was prepared using a range of the lowest and highest recorded upstream and downstream heavy metal concentrations of u2+(10.84 mg/L-108.48 mg/g), Ni2+(7.99 mg/g–79.92 mg/g), and Pb2+(33.538 mg/L—335.38 mg/L). The variants of the designed clay-based adsorbents were L-Cysteine montmorillonites (Cys-Mont), Glycine montmorillonite (Gly-Mont), and L-lysine Montmorillonites (Lys-Mont) prepared at pH 4, 5, and 6 respectively. The experimental conditions were; Adsorbent dosages range = 25 mg–400 mg, pH = 6.0, Contact time = 24 hrs, Temperature = 25°C and Shaker speed = 180 rmp. After the adsorption experiments, it was identified that the maximum average removal efficiency obtained for Cu2+, Ni2+, and Pb2+ were all attained at adsorbent dosage 400 mg/L and in the order Lys-Mont (99.82%)>Cys-Mont (99.28%)>Gly-Mont (95.8%). Thus, the modified Lys-Mont clay exhibited the highest heavy metal adsorption capacity amongst the above three studied. The highest and lowest adsorbed metal pollutants amounts recorded for Lys-Mont were Cu2+ (86.49 and 8.65) mg/g, Ni2+ (44.51 and 5.99) mg/g, and Pb2+ (267.85 and 26.67) mg/g respectively. The number of metal ions adsorbed per unit mass for each metal cation tested decreased with increasing adsorbent dosage. The general order of selectivity documented for all the AA-Monts in this study was Pb>Cu>Ni which is similar to the levels in the prepared wastewater solution (Pb>Cu>Ni). The findings of this study have shown that heavy metal polluted river basins can remediated given the right conditions of temperature, adsorbent dosage, pH and agitation speed.
Public interest statement
Ghana with its rich natural resources has significantly benefited from the exploitation of gold. By January 2016, gold mining contributed ~5.7% and ~40% to its GDP and gross foreign exchange earnings. Notwithstanding these notable gains, gold mining in Ghana continues to harm the environment, human health, and the long-term sustenance of community livelihoods.
In this research study, heavy metals (Cu2+, Ni2+ & Pb2+) associated with Ghana’s illegal gold mining (Galamsey) were adsorbed from a multi-aqueous wastewater solution using a variety of novel amino-acid modified montmorillonite clay absorbents. The best performing absorbent was Lysine montmorillonite (Lys-Mont) with a removal efficiency of 99.82% at an adsorbent dosage of 400 mg/L, pH (6), Temperature (25°C), contact time (24 hrs) and agitation speed (180rmp). This result indicates the possibility of remediating mining polluted rivers with eco-friendly, green, and inexpensive adsorbents. This is a good step in ensuring sustainable gold mining in developing countries.
1. Introduction
In recent years, the world has witnessed phenomenal growth in industrialization, urbanization and high population growth in most places (M Chen et al., Citation2020). One of the current global environmental challenge is the pollution of the ecosystems by heavy metals (Ali et al., Citation2021). Freshwater which hitherto served as source of raw water for water treatment facilities and also used in various economic activities have all been polluted with heavy metals (Bogardi et al., Citation2020). The pollution of these water bodies are as a result of illegal mining and other anthropogenic activities (Xie et al., Citation2022). The toxicity of heavy metals differs with oxidation and physical states, making it laborious to demarcate them into common toxicity ranks (Edokpayi et al., Citation2017). Several metals and metalloids already act toxically at low concentrations and are considered deadly in high concentrations. Heavy metals are known to migrate in the global ecological cycle wherein soil and water play the main roles, posing a great ecological risk. These heavy metals have varied damaging effects on the health and proper functioning of the body and its organs. They pose threats not only to the human populace but also to other terrestrial, and aquatic organisms and their habitats (Tutic et al., Citation2015). Their harmfulness lies in the substitution of essential metals in enzymes and other vital biomolecules, which leads to inhibiting functions. The effect of the heavy metals on the environment is emphasized by their non-degradability and bioaccumulation. The bio-absorption, bioaccumulation, and bio-magnification of heavy metals along the food chain consequently result in diseases such as organ damage, cancer, nervous system damage, and in some severe cases, loss of life for the human populace (Akpor et al., Citation2014).
The goal of wastewater treatment is to eliminate any current or potential threat to human/animal health and the environment (AJ et al., Citation2015). However, anthropogenic activities like unsustainable artisanal gold mining in developing countries like Ghana causes the contamination of water bodies, destroys aquatic habitats, disrupts the ecosystem and risks both aquatic and human health through the biosorption and bioaccumulation of heavy metals(Taha et al., Citation2016). From the toxicological order of harmfulness using the World Health Organization (WHO) limits for each heavy metal, the most dangerous and hazardous heavy metals in the mining wastewater found in the studies conducted on rivers in Ghana are in the order of Hg > Pb, Cd > Chromium > As> Ni > and Cu (Duncan et al., Citation2018). The depuration of polluted water and soil at present is expensive, inefficient and usually not eco-friendly. There is, therefore, the need to find an eco-friendly, efficient and economical heavy metal wastewater treatment solution. To meet this wastewater treatment requirement, researchers around the world are working to identify more efficient, new and innovative materials for heavy metal scavenging from aqueous solutions. Adsorption techniques have been widely applied to the treatment of industrial wastewater containing dyes, heavy metals, and other inorganic and organic impurities due to their various advantages including, the ease of its technical application, efficiency, cost-effectiveness, nature of its end products and desorption abilities (A. Gupta et al., Citation2021; Rashed, Citation2013). To eliminate or reduce the concentration levels of metals in wastewater, simple, natural, and low-cost adsorbents like clays which are modified with environmentally friendly materials like amino acids to increase and provide selectivity for metal adsorption, can be adopted and applied (Kurniawan et al., Citation2006). Over the last decade, studies have concentrated on identifying the potential of modified natural and ecofriendly materials (such as, boxymethyl cellulose/montmorillonite Nanocomposite (CMC/MMT-NC), Egyptian Na-activated bentonite (Na-AB) and Mesoporous chitin blended MoO3-montmorillonite nanocomposite) to remediate wastewater from organic and inorganic pollutants (Heiba et al., Citation2018; Taha et al., Citation2016). Before the successful application of the clay as an absorbent in the remediation of heavy metals from wastewater, it requires purification and the Sodium activation of a Calcium Montmorillonite (Ca-Mont) clay to the more suitable and efficient form; Sodium Montmorillonite (Na-Mont) if the experimental details require it (C. Chen et al., Citation2015; Gil et al., Citation2021).
Several studies have been conducted to investigate the ability of natural bentonite to adsorb some heavy metals. A study of the Adsorption performance of Cd2+, Cu2+, Ni2+, and Pb2+ onto natural bentonite both in mono- and multi-metal systems was conducted by (Anna et al., Citation2014). In this study, the removal of Cd2+, Cu2+, Ni2+and Pb2+ from simple-and complex-metal ion aqueous solutions using natural Calcium (Ca) bentonite from Milos island in Greece was studied. In this study, two (2) blank experiments were prepared. One without the addition of adsorbent and others without the presence of the metal were all prepared. During the testing of the varying conditions that influence the adsorption capacity of the raw/natural Calcium (Ca) bentonite, one parameter was altered whereas all other parameters were kept constant. The removal efficiency of the clay from synthetic aqueous solutions and real wastewater was investigated under various conditions such as a variable concentration of metal ions, the amount of Calcium bentonite clay (adsorbent dose), pH, and contact time. The time interval used for this study was in the time scope of 20–360 min, the adsorbent amount range was from 1 to 10 g L−1. The initially applied solution pH values were in the interval of 2–6 for Copper (Cu2+) and Lead (Pb2+), and pH of 3–8 for Cadmium (Cd2+) and Nickel (Ni2+). The results from the tests indicate that the adsorption experiments of the metal ions by the adsorbent were highly pH-dependent. Higher pH resulted in higher removal of the metals and vice versa. Increased absorbent dosages increased the removal efficiencies of the metals. (Anirudhan et al., Citation2012) researched the potential use of Amine and carboxylate functionalized bentonite (supplied by Fluka, Switzerland) as adsorbents of heavy metal ions including (Pb2+, Hg2+, and Cu2+) from aqueous solutions. The number of amine groups was 1.25 meq/g in Bent-NH2 and for carboxyl groups in the Bent-COOH 1.19 meq/g. The results from the SEM images showed that the bentonite before sialylation became visible as super-tiny flaky particles whereas the organo-functionalized bentonite particles were finer and composed of clamped thin sheet-like particles. Disparate to the water-loving bentonite particles, which formed large clamps after drying, the functionalized particles were hardly well mixed in water. (Asiabi et al., Citation2017) investigated the use of highly selective and efficient removal of Arsenic (As5+), Chromium (Cr6+), and Selenium (Se6+) oxyanions by anionic clays pillared with zwitterionic glycine. In this study, a new procedure to effectively and easily extract toxic oxyanions (Cr6+, Se6+, and As5+) from aqueous solutions using non-ionic glycine pillared anionic clay (Gly-LDH) was reported. Therefore, to study the effect of non-ionic glycine on the metal uptake capacity, selectivity, and the mechanistic procedure of anionic clays (LDHs), two NiAl LDHs pillared with varying inter-layer anions, including NO3− and glycine, were manufactured. For the chemical analysis of layered double hydroxides (LDHs), the Inductively Coupled Plasma-Atomic Emission Spectroscopy and EDAX analyses were used. The X-ray diffraction, BET specific area, and BJH techniques, as well as Scanning Electron Micrometer and IR spectroscopy, were used to identify the new traits of the new products and certify the pillaging of the glycine into the inter-layer gaps of the NiAl-LDH. The extraction of oxyanions by LDHs was examined using the batch experimental method and the influences of the pH of the solution on the metal uptake of oxyanions were also investigated using a constant contact time and temperature of 3hrs and 25◦C, respectively. The XRD patterns of the NO3-LDH and Gly-LDH showed a high degree of crystallinity and layered structure. The basal spacing of Gly-LDH (8.25 Å) compared with NO3-LDH (2.98 Å), was larger which indicating the successful intercalation of LDH by glycine. It can be said that both NO3-LDH and Gly-LDH presented a plate-like morphology, which is typical for layered double hydroxides of size ~100–400 nm and thickness ~10–50 nm. A decrease in pH caused a decline in the metal uptake ability, which is connected to the partial decomposition of the Gly-LDH. Their results indicate that the metal uptake ability and metal preference indicator of oxyanions occurred via the ion-exchange mechanism in NO3-LDH and were lower than Gly-LDH. Gly-LDH displayed a selectivity order of Se6+ < Cr6+ «< As5+ for the oxyanions.
Limited or no research has been conducted on the ability of 3 different Amino-acids to adsorb heavy metals from any multi-aqueous mining wastewater. The objective of this study is to identify and assess the most suitable novel Amino-acid modified clay adsorbent and its optimum adsorption conditions needed to efficiently extract three of the known heavy metals pollutants (Nickle, Lead, Copper) in the synthesized Pra River basin mining wastewater. The characteristics and adsorption properties of the novel clay based adsorbent were identified and discussed. This research will serve as a guiding document for large scale application for industrial work targeted remediating heavy metals especially from gold mining polluted water resources.
2. 1 Materials and chemicals
Natural Calcium Montmorillonite (Ca-Mont) clay was used in the experiment. The clay was gray in color and rocky in texture and had medium-sized lumps. For the surface functionalization/intercalation into the clay, three different amino acids: Glycine, L-cysteine, and L-Lysine (Aladin, purity >99%) were used. The natural Calcium Montmorillonite was crushed to small lumps by hammering and then grounded, sieved, and dried (at 70°C) to get a fine powder using a 71um sieve. The Bentonite clay is here referred to as montmorillonite in this study because it was purified during the sodium activation process to make it get a purer form of Montmorillonite clay.
Montmorillonite purification and sodium activation
To activate and purify the Montmorillonite Clay with sodium Na+ (referred to as “Soda activation”), 25 g of the raw and natural montmorillonite and 1.5 g of Na2CO3 were weighed and added to 500 ml deionized water. The mixture was stirred at a speed of 3000 rmp for 30 min with a high-speed homogenizer. The suspension was ultra-sonicated with a rod-shaped ultrasonic machine at 80 kHz for 10 min. The suspension is then centrifuged at 10,000 rmp for 10 min, after which the uppermost part is removed and dried as the purified Na-Mont leaving the bottom precipitate of impurities. The collected uppermost montmorillonite sample was dried at 70°C and further ground and sieved with a 71um/200mesh sieve to obtain fine powder for experimental use and labeled as K1.
2.2 Modification of amino -Acid modified montmorillonite (AA-Mont)
Why use amino acids?
Amino acids are vital sources of nitrogen in soils since chemical analysis different soil samples revealed a large number of amino acids and amino sugar residues (Cheshire et al., 2000). The use of amino acids as montmorillonite clay additives is suggested by their binding of heavy metals in soils. However, the pH, contact time, adsorbent dose, temperature, and heavy metal concentration are all factors that can determine the adsorption performance of its modified clays in aqueous solution. Amino acids are intercalated into the interlayer space and bind with the Montmorillonite clay by either creating a strong electrostatic interaction between its NH3+ and negatively charged surface of clay or by the coordination between its carboxylate (COO-) groups and the silicate layer of the clay (Kitadai et al., Citation2009; Zhu et al., Citation2019).
Selection criteria for the amino acids
Three amino acids were selected for this experiment on criteria to enable the comparison of the influence of functional groups of amino acids on adsorption properties of the montmorillonite in a complex metal solution. Also, previous studies conducted by other researchers involving the selected amino acids intercalated on montmorillonite clay minerals have showed improved adsorption in single heavy metal-polluted wastewater, hence providing the required basic relevant knowledge to undertake the adsorption experiment time in a complex aqueous solution (Kitadai et al., Citation2009; Zhu et al., Citation2019).
For the chosen amino acids, the values of isoelectric points and selected pH for the intercalation experiments are as follows:
pI = 5.97 for Glycine, pH<pl = 5.0, pH> pl = 7.0, pl = 9.74 for Lysine, pH<pl = 6.0, pH>pl = 11.0,
pl = 5.07 for Cysteine, pH<pl = 4.0, pH>pl = 6.0
The preparation of the Amino-acid modified montmorillonite through intercalation is a hydrophilic process and highly water intensive. The interaction of montmorillonite with its water swelling property is propelled by the migration of montmorillonites surface counter-ions to the central interlayer plane (Hensen & Smit, Citation2002). A study conducted to determine the adsorption energies between the interlayer cations and water molecules to that of the montmorillonite layers discovered that the exchangeable interlayer cations had more significant negative energy driving force than the later. Implying that the hydration of the montmorillonite clay can be greatly attributed to the nature its interlayer cations than the layer of the montmorillonite clay itself (Peng et al., Citation2019).
To prepare the Amino acid modified montmorillonite, 5 g of montmorillonite was added to 500 ml of distilled water (ratio 1 g: 100 ml) and amino acid solution at pH Cys (4.0), Gly (5.0), Lys (6.0), which was adjusted with 1 M of HCl or NaOH. The suspensions were stirred at a constant rate of 300 rmp at room temperature of 25°C for 24 hrs using a magnetic stirrer. Thus, the adsorption was allowed to reach this obvious equilibrium, and then the tubes were centrifuged. The solid phase (AA–Bent) was separated by centrifugation at 9000 rpm for 15 min, washed 3 times with distilled/Milli Q (18 MΩ cm−1) water and then the products were dried in an oven at 70°C, ground with a crucible, sieved through a 71um/200 mesh and stored in an air-tight plastic bottle for subsequent studies. The modified montmorillonite was designated the name Cys–Bent, Lys-Bent, and Gly-bent for easy identification.
2.4 Synthesizing the PRA River simulated aqueous complex heavy metal solution
The synthesized mining wastewater solution was prepared using 3 of the heavy metals that were present and were in high concentration when measured in the Pra River Basin. All solutions were prepared with high-purity water (18 MΩ cm−1) obtained from a Milli Q water purification system.
The synthesized mining wastewaters’ constituent was similar to those reported for the Ghana Pra river and was treated with the modified clay at established optimized conditions to assess the effectiveness of montmorillonite clay for the removal of metal species from the synthetic wastewaters.
Preparation of Heavy Metal Stock Solution
To calculate the total concentration of the complex heavy metal solution, the concentration of individual heavy metals was calculated manually its mass (mg), molar mass (g/mol) and then summed up to obtain the total concentration in mmol. The concentration of the complex heavy metal was found to be 4.6879 mmol
A 500 ml complex heavy metal polluted waste water solution of 10times concentration was prepared with the following values in order to obtain the needed concentration.
The complex solution was prepared and stored in a clean 500 ml polyethylene plastic bottle with a cover instead of a glass to prevent any reaction between the container and the heavy metal solution. After carefully weighing the grams needed to prepare a stock solution of 10 times the actual concentration needed into the container, by the use of an electronic scale distilled water was added to it till it reached the 500 ml calibration mark on the container. The solution was mixed for 10 minutes, 25°C and at 1000 rmp by the help of a magnetic stirrer and a magnetic stirring rod.
2.3 Characterization techniques
XRD
The grounded and sieved clay was pressed against the stainless steel sample holder of an X-Ray diffraction (XRD) machine. XRD pattern of the samples was collected through CuKα radiation (λ = 1.5418 Å) by employing an Ultima IV X-ray diffractometer with a diffracted beam monochromator and a rotating sample holder. It operated at an applied voltage of 40 kV and 40-mA current. The patterns were recorded from between 2° and 80° (2θ) at a step size of 0.016° and a counting time was 1 s per 0,02°20. The basal spacing was calculated from the 2θ value using Bragg’s equation, n*λ = 2d sin θ; where n is an integer, λ the wavelength and θ the scattering angle.
FTIR
The infrared spectra from the FTIR of all prepared adsorbent samples (Cys-Mont, Gly-Mont, and Lys-Mont), were collected including that of the raw sample (Ca-Mont). The investigation of FTIR was conducted using a Nicolet 5700 US PE Fourier transform IR spectrophotometer in the wavenumber range of 500–4000 cm−1.
SEM
The Scanning Electron Microscope (SEM) was used to analyze the surface morphologies of the materials. The images were captured at a magnification of 15.00KX, EHT = 5.00 kV, WD = 9.2 mm, Gun vacuum = 2.72e-009 mbar using an Oxford instrument, X-Max, Zeiss Ultra 55, module number 4006–800-720 (Germany).
Adsorption Experiments and Analytical techniques
The American Thermo-fisher ICP-OES (iCAP6500) was the Inductively Coupled Plasma – Optic Emission Spectroscopy employed for the adsorption performance of the synthesized mining wastewater after the adsorption process.
The removal efficiency and the number of cations adsorbed qt (mg /g) by each amino acid intercalated clay was calculated according to Eqns. (1) and (2):
Where,
C0 (mg/ L) = the initial concentration of cations, Ct (mg/L) = the cation concentration at time t (min), m (g) = the mass of adsorbent, V (L) = the volume of adsorbate solution and Qt (mg/g) = the adsorbed amount at time t (min).
Adsorption experiments
All experiments were performed under aerobic conditions and with chemicals of analytical purity. The adsorption capacity of Pb2+, Cu2+ and Ni2+ on AA-bent was investigated using the batch technique. However, to ensure maximum metal removal, a contact time (24 hrs), pH (6) and shaker speed (180 rpm) was applied in all experiments conducted. The heavy metal solution was prepared by pipetting (1 ml, 2 ml, 4 ml, 6 ml, 8 ml, and 10 ml) into plastic ethylene bottle using an electronic pipette. The solution was then diluted with distilled water from Milli Q to the 100 ml mark (to the required concentration) and thoroughly mixed by a magnetic stirrer. The pH is then adjusted using NaOH. Afterwards, the adsorbent dosage ranges of (0.025 g, 0.05 g, 0.1 g, 0.2 g, 0.4 g) of each AA-Mont clay (Lys-Mont, Cys-Mont and Gly-Mont) for each batch was carefully weighed and added to the plastic bottles with lids. The magnetic stirrer is replaced in bottles, covered and put in a hot water bath at speed 15 rmp and at temperature 25°C for 24 hrs.The solid phase was separated by centrifugation at 4000 rpm for 20 min. The precipitate and the aliquot was filtered through a 0.45 mm syringe filter, then labeled and stored plastic ethylene bottles with lids for further analysis. The advancement of the metal uptake was analyzed by determining the concentration of metal cation in the aliquot by ICP-OES whilst the precipitate was dried in an oven at 70°C. All the experiments were replicated and average values used for the result analysis.
3. Results and discussion
3.1 Characterization results
The X-ray powder diffraction pattern of the raw and unpurified Montmorillonite clay is illustrated in Figure . It shows Montmorillonite clay mineral as well as other impurities such as quartz and calcite among other impurities. The d001 spacing was found to be ~15.04 A°, suggesting that the raw is most likely Ca-Montmorillonite. The chemical components of the raw and purified Na2CO3 activated Montmorillonite was measured using X-Ray fluorescence (XRF) and the Fourier Transform Infrared Spectroscopy (FTIR).
The elemental analyses of the raw montmorillonite sample as shown in Table show that the clay obtained was a Calcium Montmorillonite with as low as 0.13% for Na2O. It also revealed traces of other heavy metal impurities, such as Fe2O3, TiO2, and ZnO, in the clay structure.
Table 1. XRF results for the raw Montmorillonite sample
The X-ray diffraction patterns of the original raw Ca-Mont and purified Na-Mont activated samples are illustrated in (Figure ) above. The Na-activated sample has less quartz as compared to the Ca-Mont. According to (Viseras et al., Citation2007), the quartz content should not exceed 2% due to adequate evidence of its carcinogenicity in laboratory animals and to some extent in humans. The Na-montmorillonite obtained by soda activation at 6 g Na2CO3/100 g bentonite has a lower inter-planar spacing of ~12.8 A° than the original raw Ca-montmorillonite which had a d001 spacing of ~15.04 A°. This resulted in Na-Mont having a smaller angle which caused a peak shift of the montmorillonite. This peak shift suggests the conversion of the raw clay Ca2+ to Na+ montmorillonite type of clay as the Na2CO3 activation caused more to be occupied with Sodium cations that were originally found in the interlayer space of the structure of the montmorillonite clay mineral. It was also noticed in the Xrd pattern that aside from the d001 peak shift, all the other peaks remained relatively at the same points signifying that the basic skeletal structure of the raw Calcium Montmorillonite remained unchanged and that the Sodium activation procedure used on the raw montmorillonite clay had still retained a majority of the nature of the raw Calcium Montmorillonite and its desired characteristics.
The elemental analyses conducted with the help of an XRF test of the raw and activated samples are shown in (Table ). A slight decrease of SiO2 and Al2O3 after Na2CO3 activation was observed, which confirmed the removal of some amount of quartz impurity and the proportional increase of the clay mineral content per the results. Also, the Na2O content increased significantly as compared to the increase observed in CaO. The calcium content of this test reflects both the interlayer cation and the calcite content of the samples. Calcite was present in most montmorillonite clays and as shown earlier by the XRD was present in this experimental clay and this could be the cause of the increase of Ca cations identified after soda activation, however, the Sodium content reflects only the interlayer Na+ cations. This can be associated with the conversion of Calcium Montmorillonite (Ca-Mont) to Sodium Montmorillonite (Na-Mont) clay. Research studies by (Ballah et al., Citation2016) have established a link between the type of exchangeable cations found in montmorillonite clay minerals and how it influences its roughness in the order Li+ < Na+ < K+ < Ca2+. Contact angle values is mainly controlled by the hydration energies of the respective exchangeable cation found in the clay interlayer. Clays with large particle sizes have large contact angles and vice-versa. Both surface roughness of films and contact angles of clay minerals relates to its particles size. It was expected that since the raw Calcium montmorillonite was soda activated will have a less rough surface than the Sodium montmorillonite. It was expected that since the dominant exchangeable cation in this activated montmorillonite was Na+ hence the swelling properties this modified clay improved as compared to the raw Ca2+ clay when mixed with water during this experiment study. Swelling montmorillonites (Na Bentonites) as compared to non-swelling ones (Ca Bentonites) exhibit a greater degree of dispersion, plasticity, and rheological properties due to its larger contact angle.
Table 2. XRF results of both Raw and Na-Activated and purified clay
In contrast to the increase seen in Na cations, all the other contents decreased slightly with some Cl− and Y2O3 being absent from the Soda activated sample as seen in the results from the XRF analysis. SrO and Rb2O remained unaffected and very little amounts of ZrO2 were still present in the purified sample and which also marginally increased. Finally, the percentages of Fe2O3, TiO2, K20, P2O5, SO3, and BaO because of the strong bonds to hold them to the clay structure experience only a slight decrement in the activated sample. These changes that occurred in the Soda activated sample in comparison to the raw Ca-Mont clay mineral figure indicate an increase in chemical purity and whiteness of the purified clay as the procedure chosen also helped to remove impurities present in the clay.
The X-ray diffractometry patterns, as shown (Figure ), reveal that the layers of the Na-montmorillonite were propped open upon swelling causing increases in the d001 basal distances after the amino acid modification, suggesting that the modifiers were pillared into the interlayer of the Na-Mont and expanded the interlayer space. As was expected, the activation process was mainly driven by electrostatic interaction between the negatively charged montmorillonite (within the interlayer and/or at the edge) and the positively charged (cationic) state of the 3 Amino acids (Kitadai et al., Citation2009). This shows that the intercalation was successful with each protonated amino acid at all the different pHs used, even after removing the solvent and washing off excess amino acid from the clay’s outer surface. For all the modified amino acid samples tested, a shift of the basal peak to smaller θ values was noticed. This indicates an increase of the interlayer spacing in the structure of the modified clay minerals, which can only be further explained as providing confirming that, the intercalation of amino acid molecules caused the expansion of clay structure. The d001 of all the selected Amino-acids increased from the Na-Mont (1.28) nm to Cys-Mont (1.56) nm, Gly-Mont (1.56) nm, and Lys-Mont (1.44) nm after modification. The values obtained were similar to those reported by (El Adraa et al., Citation2017; Zhu et al., Citation2019) and are slightly higher with an insignificant marginal difference. Comparing the XRD patterns of AA-Monts with the Na-Mont, it can be observed that only d001 surface diffraction peaks of the AA-Monts shift to a smaller angle, and all other peak positions remained unchanged. This indicates that the modification process did not significantly alter the lamellar skeleton structure of the high adsorption property of Na-Mont clay mineral but maintained it. This report is consistent with the results published by (Zhu et al., Citation2019), but higher than the results reported by (Kollár et al., Citation2003) where the Glycine-Montmorillonite and higher for Lysine-Montmorillonite prepared at pH 4.0 had d001 values of 1.32 nm and 1.36 nm respectively. It was however noticed that the peak shift for Cysteine and Glycine were equal although prepared at different pHs of 4.0 and 6.0 respectively. The interlayer spacing for particular adsorbents was determined using the Jade 6 mineral software and can be calculated from the Bragg law equation as described before using obtained θ values. The results of calculated interlayer spacing along with values of Montmorillonite clay structure expansion are presented in (Figure ). For the differences in expansion values between different types of amino acids modified clay, it was expected that the expansion values would be associated with the size of the molecule. Surprisingly, Glycine, as the smallest volume and basic molecule among amino acids selected and used in the research has one carboxyl group (COOH) and one amine group (NH2) in its structure. Glycine had an expansion value the same as cysteine which has an additional Sulfhydryl (SH) group and was greater than Lysine which also has another side chain amine (NH2) group aside from its basic alpha amine group. It can be concluded that steric effects are not responsible for the rate of expansion as seen in the XRD results of the theta angles.
BET Surface Area
Generally, ion exchange is a dominant way for organic cations to intercalate into the interlayer of Na-Mont. When the inserting amount is less than the CEC of Na-Mont, the process includes the diffusing out of counter ions and the diffusing in of organic cations. The faster the interlayer cation diffused, the easier the organic cation intercalated. An increase in the interlayer spacing (d001) and BET surface areas of the ion-exchanged materials can verify if ion exchange was the main event for the intercalation process for this particular experimental study (Kollár et al., Citation2003). For most parts of the results displayed above in (Table ) above, there was an increase in the interlayer spacing and the Bet surface areas and hence we can conclude that ion exchange was the main event for the amino acid intercalation process. An increase in the surface area leads to an increase in the collision frequency and this determines how fast the reaction takes place and vice-versa. The increase in the d001 spacing values for both the Ca Mont and the Na Mont was also seen for the BET specific surface area values in both the Sodium activated montmorillonite (Na Mont) than its raw counterpart (Ca Mont) with values (77.40) and (66.00) respectively. The surface area of the Ca-Mont, Na-Mont, Cys-Mont, Gly-Mont, and Lys-Mont was determined by the BET N2 adsorption method. The values of the specific surface area of the table above unexpectedly did not reflect after the sequence of the expansion values as identified in (Table ) above for the Amino acid modified montmorillonite clays. Cys-Mont prepared at a pH value of 4.0 had a higher specific surface area value of (91.58) m2/g as compared to its counterpart Cys-Mont prepared at pH of 6.0 (41.29) m2/g. Although both pHs experienced the same peak shift and expansion value, they had a significant difference in their specific surface area values. However, the trend of Gly-Mont prepared at pH of 7.0 and 5.0 was different as the values reported for their specific surface area had a small margin of difference, (86.66 m2/g) and (88.31 m2/g) for both respectively. For the case of Lysine Montmorillonite (Lys-Mont) prepared at a pH of 6.0 and 11.0, a similar trend of Gly-Mont was evident, however instead of a marginal difference, a distinct difference was identified between a pH of 6.0 (51.61 m2/g) and pH of 11.0 (42.25 m2/g). This result is inconsistent with the values for the BET specific surface area reported by (Kollár et al., Citation2003). In their experimental study, the BET surface area value was lower for Glycine-Montmorillonite (70.4 m2 /g) and higher for Lysine-Montmorillonite although both were prepared at pH 4.0 (74.5 m2/g). However, the decrease in surface area after intercalating the lysine is suspected to have originated from the aggregation of the particles which caused the blockage of the mesopores of the adsorbed Lysine cations as similarly c reported by (Anirudhan et al., Citation2012) and (Yildiz et al., Citation2003). Although no further tests were conducted to determine the exact site at which the lysine cations were adsorbed/intercalated on the clay surface, it is however suspected that the lysine cations were adsorbed on the edges or surface of the montmorillonite clay rather than its interlayer space.
Table 3. Characterization data of both the raw, sodium activated and the amino-acid intercalated clay materials at different pH
Based on the evidence of the increments seen in the d001 spacing for most of the AA-Monts clay samples at pH (i.e. Cysteine, Glycine, and Lysine, there is proof occupied the inter-lamellar spaces of the Sodium (Na) Montmorillonite clay as shown (Figure ) and causing a pillaring effect and hence there was no leaching of the amino acids during the clay intercalation process. In the case of the adsorbed lysine on the montmorillonite, it is suspected to be dominantly present in its cationic state at an applied pH (pH = 6.0). This also indicates that the adsorption of the montmorillonite was mainly driven by electrostatic interaction between the negatively charged montmorillonite surfaces and positively charged cationic lysine. It is also likely that lysine interacts with the montmorillonite surface through the protonated side-chain amino group and has a preferred vertical orientation, with the side-chain amino group pointing toward the surface as reported by (Kitadai et al., Citation2009).
SEM Morphology of raw and Na2CO3-Activated Montmorillonite Clay
The Figures above shows the SEM images of Na-Mont and Ca-Mont. It was observed that both Na-Mont and Ca-Mont displayed lamellar structures at 2um. The pronounced difference noted between their surface morphology was that, there was more evidence of aggregation and less porosity in Ca-Mont while that of the purified Na-Mont was looked more porous and less aggregated. Na-Mont also had larger particle sizes and uneven particle size distribution when compared to all the AA-Mont clay sample SEM images under study (Gly-Mont, Lys-Mont, and Cys-Mont).
It was observed (in Figure above) that after the pillaring/intercalation of the Amino-acids into the interlayer of the Montmorillonite clay. The Cys-Mont and Gly-Mont displayed lamellar surface morphology. The particles of both modified clay surface were more dispersed after modification. The particle size was loose and uniform, and many small pore channels were present which were produced most likely from the intercalation process. The particles of the Lys-Mont surface were more aggregated after modification as compared to the other clay samples captured. The particle size was irregular and with large pore channel instead of the many small channels displayed by Cys-Mont and Gly-Mont clays. This is consistent with the results from the BET surface area value for Lys-Mont which indicated a reduction in the surface area of Na-Mont clay after it was intercalated with the Lysine cations.
FTIR
The FTIR analysis was conducted to further verify if the amino acid retention in the structure of the montmorillonite clay material is the result of intercalation. Fourier transforms infrared (FTIR) spectra of Ca-Mont and AA-Monts (i.e. Cys-Mont, Gly-Mont, and Lys-Mont) were detected in the range of 500 cm−1- 4000 cm−1of wavenumbers. As shown in Figure , the FTIR of Ca-Mont and AA-Monts were very similar, and there were less obvious significant changes between them, which indicates that the basic skeleton structure of modified and unmodified montmorillonite had not changed. The peak corresponding to the OH stretching of the structural hydroxyl groups at 3626 cm−1 was attributed to Ca-Mont. In Figure , the peak near 3433 cm−1 was the O-H stretching vibration of water and H-O-H deformation occurs at peak 1642 cm−1 was also present in the FTIR spectrum of Ca-Mont. The peak around 918 cm−1 represents the Al-OH-Al bending. The sharp absorption peaks observed at 1024 cm−1,470 cm−1 and 523 cm−1 belonged to the typical Si-O stretching vibration, Si-O-Si bending, and Al-O-Si deformation respectively of the Ca-Mont. This was similar to the results published by (Alemdar et al., Citation2015). The FT-IR spectra of the intercalated substances including Gly-Mont and Cys-Mont are displayed in Figure . Generally, all the spectra were dominated by the bands of the host material, but in each case, the presence of the amino acid is also seen partially or more vividly. In the spectra of the intercalated substances, certain features of the amino acids can be noticed since these features were not reflected in the spectrum of the host material. Since spectra used for comparison are not those of the protonated amino acids, but those of the amino acids, shifts in the position of certain bands as to be expected were observed across all the spectra for the intercalated clay substances. Nevertheless, a closer inspection of the 1730–650 cm−1 of the intercalated materials and its comparison with the same wavenumber interval of the amino acids and that of the host material reveal that guest ions are in the montmorillonite or on its outer surface. The protonated amino acid ions should be large among the layers since, after the intercalation process, the montmorillonite clay material was intensively washed, thus, allowing all excess guest ions of the amino acid not bounded on the surface to be removed. However, chemisorption of the protonated amino acids on the outer surface of montmorillonite cannot be ruled out completely since no test was conducted to ascertain the certainty of the excess guest ion removal efficiency. In a detailed observational and comparison study, it is seen that the FTIR spectra of Lys-Mont (Figure ) show an absorption peak around 1501 cm−1occurring because of the shear vibration of the—CH2—group and subsequent adsorption peak near 1343 cm−1 and 1416 cm−1 been the C-N stretching vibration and the peak of was COO—symmetric vibration respectively. The characteristic absorption peak of the modifier Lysine salt appeared, indicating that the modifier had successfully entered the interlayer of Na-Mont, confirming the earlier stated results of the XRD characterization. In a comparison of the raw Ca-Mont with the Gly-Mont and Cys-Mont spectrum, no significant differences were observed except for a series of visible peaks in Ca-Mont in the range 650–900 cm−1which was less evident in them. The impact of montmorillonite in the spectrum is very strong and predominant. The amino acid retained in the interlayer space of the clay seems much diluted, hence the difficulty in identifying any visible peaks to represent it. A close inspection of the 1500–1100 cm–1 and 700–1000 cm–1 of Lys-Mont in comparison with the same wavenumber interval of the host Raw/Ca-Mont though slight suggests that the guest Amino-acid species was adsorbed by the clay sample.
3.2. Removal efficiency and effect of adsorbent dosage
The effect of the adsorbent dosage was studied on the different Montmorillonites (Cys-Mont, Gly-Mont, and Lys-Mont) amounts ranging from 25 mg to 400 mg and the results are shown in (Figure ). As it was found across all the Amino Acid Modified Montmorillonites, upon increasing the adsorbent dosage, the adsorbed heavy metal ions (i.e. their removal efficiencies) increased. The increment in the removal efficiencies for all the Heavy metals testes in this study (Cu2+, Ni2+, and Pb2+) was steady in the case of Cysteine Montmorillonite (Cys-Mont) and Glycine Montmorillonite (Gly-Mont), whereas it increased rather steep for Lysine montmorillonite (Lys-Mont). This could be attributed to the fact that an increase in the clay concentration increases the surface area of the adsorbent, which in turn increases the number of binding sites for the same liquid volume and thus the total amount of metal ions removed increases (Sen & Gomez, Citation2011). The maximum average removal efficiency for Cu2+, Ni2+, and Pb2+ was attained at a Montmorillonite concentration of 400 mg L−1 for all cases, Lysine Montmorillonite (Lys-Mont) with the highest value, followed by Cysteine Montmorillonite (Cys-Mont) with an average percentage removal efficiency of 99.82% and 99.28% respectively. This results compares similarly to other absorbents like modified chitosan (CS-ES) which had an adsorption efficiency of about 99.07% for Au3+ in an aqueous solution(Zhu et al., Citation2019) and a biomaterial activated Sawdust-Chitosan nanocomposite beads (SDNCB) powder with its highest removal efficiency for Cu2+(92.54%) and Ni2+(88.24%) at pH of 10 (Kayalvizhi et al., 2022). The lowest average percentage removal efficiency for Glycine-Montmorillonite (Gly- Mont) achieved was 95.8% and at an adsorbent dosage of 400-mg L−1. The highest adsorbed amounts for Cu2+, Ni2+ and Pb2+ were (108.4 mg/g), (77.48 mg/g) and (335.0 mg/g) by the Lys-Mont at 400-mg adsorbent dosage. The least average percentage removal efficiency was at an adsorbent dosage of 25 mg with values for Lys-Mont, Cys-Mont, and Gly-Mont being 89.71, 90.56, and 91.62 mg/g respectively. However, the dosage of 400 mg L−1 is not considered the optimum dosage since none of the Amino Acid modified montmorillonites (AA-Monts) used in this experiment recorded an approximately 100% metal removal efficiency. It is however, estimated that the optimum dosage will lie between 400 and 450 mg based on these obtained results and further adsorption experiments would need to be conducted to determine whether any further addition of the Amino Acid Modified Montmorillonite would not cause a significant change in adsorption removal efficiencies. This study’s result is also consistent with that reported by other publications including (Anna et al., Citation2014).
Figure 9. Total Heavy Metal removal efficiency for the Amino Acid modified Montmorillonites (AA-Monts) at adsorbent dosage (25 mg—400 mg), pH(6), Shaker speed (180 rmp) and temp. (25°C).

Furthermore, as is observed in (figures. ) above, the number of metal ions adsorbed per unit mass for each metal cation tested decreased gradually with the increase of adsorbent content in the range (20 mg—400 mg) despite the type of Amino acid montmorillonite used for the adsorption experiments. Whether it was Cysteine Montmorillonite (Cys-Mont), Glycine Montmorillonite (Gly-Mont), or Lysine Montmorillonite (Lys-Mont) that was used for the modification, the trend was almost the same throughout the adsorption studies. The highest and the least values for Cys-Mont were Cu2+ (86.51 and 8.25) mg/g, Ni2+ (46.25 and 6.04) mg/g, and Pb2+ (267.38 and 26.51) mg/g. For Gly-Mont the highest and lowest values recorded were Cu2+ (84.24 and 7.49) mg/g, Ni2+ (49.96 and 6.25) mg/g, and Pb2+ (267.41 and 26.70) mg/g. Finally, for Lys-Mont the highest and lowest recorded values were Cu2+ (86.49 and 8.65) mg/g, Ni2+ (44.51 and 5.99) mg/g, and Pb2+ (267.85 and 26.67) mg/g. The differences between the adsorbed amounts (Qt) of the metal cations were rather small. This may also indicate that the amino acids studied in this research work could form complexes with a high affinity for the tested cations. This is consistent with results from other studies like (Anna et al., Citation2014) and (Liu et al., Citation2020) who reported that excessive dosage of adsorbents hindered mixing efficiency and interfered with the mass-transfer process. At high mineral concentrations, the available metal concentration is insufficient to cover completely the exchangeable sites on the adsorbent, usually resulting in low metal adsorption capacity (Yang et al., Citation2010). Besides, higher clay dosages increase the probability of collision between the particles and therefore may create particle aggregation, decreasing the total surface area and an increase in diffusional path length, both of which contribute to the decrease in the sorption capacity of metal ions on the Amino acid-modified montmorillonites (AA-Monts; Carnoyale & Cuadra, Citation2004). This same behavior has been reported for other adsorbent materials on metal ion adsorption by (Mishra & Patel, Citation2009; Sen Gupta & Bhattacharyya, Citation2012; Yong & Ye, Citation2012) and (Veli & Alyüz, Citation2007). In the above publications, the absorption mechanism involved in the absorption process with the amino-acid modified clay was complex and was composed of Ion exchange and complexation reactions between the heavy metal cations and the amino acids.
Figure 10. Adsorption of Cu2+, Ni2+, and Pb2+ by Cys-Mont at adsorbent dose range (20 mg-400 mg) pH(6), Shaker speed (180 rmp) and temp. (25°C).

Figure 11. Adsorption of Cu2+, Ni2+, and Pb2+ by Gly-Mont at adsorbent dose range (20 mg-400 mg) pH(6), Shaker speed (180 rmp) and temp. (25°C).

Figure 12. Adsorption of Cu2+, Ni2+, and Pb2+ by Lys-Mont at adsorbent dose range (20 mg-400 mg) pH(6), Shaker speed (180 rmp) and temp. (25°C).

Effect of Initial Heavy Metal Cation Concentration
The trends of the adsorption results for all heavy metals tested at different initial concentrations of Cu2+ (10.848–108.48) mg/L, Ni2+ (7.99–79.92) mg/L, and Pb2+ (33.538–335.38) mg/L are shown in (figures. ). The amount of heavy metal adsorption by the Amino acid-modified Montmorillonites (AA-Monts) increased rapidly with an increase in the initial concentration of the synthesized complex metal solution. The heavy metal removal efficiency of 25 mg of AA-Monts at the initial concentration of Cu2+ (10.848) mg/L, Ni2+ (7.992) mg/L, and Pb2+ (33.538) mg/L was lower, and then increased steadily when the initial concentration increased and moved through until its highest and final concentration was to 108.48 mg/L for Copper (Cu2+), 79.92 mg/L for nickel (Ni2+) and 335.38 mg/L Lead (Pb2+). This same trend was observed for the Amino Acid Modified Montmorillonites (Cys-Mont, Gly-Mont, and Lys-Mont,) tested This process was due to the adsorbents in the solution contain a fixed number of active adsorption sites and the active adsorption sites were initially in excess at the lower concentrations of the solution. But with the continual increase in the initial concentration of heavy metals in the complex metal solution, the adsorption amount increased rapidly and hence more metal cations were taken out of the solution until all limited active adsorption sites were depleted. The adsorption mechanism seems to involve ion exchange and perhaps complexation reactions (Liu et al., Citation2020).
Figure 13. Adsorption capacity (Qt) with increasing initial concentration for the Amino acid-modified Montmorillonite Cys-Mont at adsorbent dose range (25 mg), pH (6), Shaker speed (180 rmp) and temp. (25°C).

3.3. Selectivity
Generally, complex heavy metal solutions exhibit three possible types of behavior: synergism, antagonism, and non-interaction and for these experimental conditions used, the competition was likely to occur on all of the different adsorption sites (Tovar-Gómez et al., Citation2015). Due to the limited scope of this study, the competitive effects occurring on the strong cation exchange sites could not be identified. However, it is expected that further future research in this study will likely reveal the competition that existed for this adsorption experiment. Notwithstanding, across the modified montmorillonite clays studied (Cys-Mont, Gly-Mont, and Lys-Mont), the general selectivity order of the adsorption was Pb2+ > Cu2+ > Ni2+ in most of the case studies despite the adsorbent dosage or initial concentration applied, except for a few outliers. (Anna et al., Citation2014) reported that a reverse of our obtained selectivity their raw unmodified montmorillonite in the order Cu2+ > Ni2+ > Pb2+ > Cd2+ in both single and multi-component systems. This indicated that their adsorption was not affected by competition and that the adsorption mechanism was likely the Langmuir adsorption isotherm. However, (Faghihian & Nejati-Yazdinejad, Citation2009) reported that a study of modified Cysteine bentonite had a selectivity order of Cd2+ > Pb2+ unlike the above and the isotherm was also the Langmuir adsorption isotherm. A similar result of the adsorption isotherm was identified for Lysine-modified montmorillonite used in removing Lead (Pb) cations by (Zhu et al., Citation2019). Additionally, the order of selectivity for cysteine montmorillonite was found in the order Cu2+ > Zn2+ > Pb2+ > Hg2+ > Ni2+ > Co2+ by (El Adraa et al., Citation2017) in an aqueous single component system. Although it was expected that the study’s metal selectivity would follow a similar pattern in published literature, nonetheless the sequence did not mimic the same result pattern. Ni2+ had the least selectivity as expected, but Pb2+ was highly selected over Cu2+. It is argued that the initial heavy metal concentrations have greatly influenced the heavy metal selectivity sequence, but additional laboratory experiments are needed to make this factual.
Conclusion
An abundant, eco-friendly, and natural substance (Mont-clay) was purified and intercalated with amino acids (Glycine, Cysteine, and Lysine) for the removal of Cu2+, Ni2+, and Pb2+ ions from a synthesized complex metal solution under varying conditions of adsorbent dose and metal concentration. The characterization tests (FT-IR spectroscopy, X-ray diffractometry, SEM, and BET surface area) were conducted. Results indicated that the protonated forms Cysteine, Glycine, and Lysine had successfully intercalated into the montmorillonite host. The Amino acid montmorillonite clays: Cys- Mont, Gly-Mont, and Lys-Mont were used to conduct adsorption studies in a multi-aqueous solution simulated to mimic the concentrations of heavy metals identified in the polluted Ghanaian Pra River basin. Their performances were evaluated using their absorbing capacity and removal. Based on the obtained results, the highest average metal percentage removal efficiency at adsorbent dose (400 mg) was Lys-Mont showed of (99.8%)>Cysteine (99.28%)> Glycine (95.8%). Also, the adsorption capacity of the AA-Mont clays decreased with increasing adsorbent dosage for all 3 adsorbents under study. The highest amount adsorbed by Lys-Mont for Cu2+, Ni2+and Pb2+ was 108.4 mg/g, 77.48 mg/g and 335.0mg/g, respectively. The general selectivity order of the adsorption was Pb2+>Cu2+>Ni2+ for all AA-Mont clay adsorbents under study. Lysine Montmorillonite (Lys-Mont) was identified to be the most efficient modified clay mineral essential for heavy metal adsorption in this experimental study with a removal efficiency of 99.82% and total adsorption capacity of 398.85 mg/g and compares to other well performing adsorbents in literature.
The study in summary demonstrates that Amino acid modified montmorillonites have the capacity to remediate heavy metal polluted waters like the Pra river basin and further studies into this subject is likely to provide a green, eco-friendly and cheap material that can be locally manufactured for large scale adsorption processes in developing countries.
Limitations of the Study
This study used a contact reaction time of 24 hours (assumed reaction time to reach equilibrium). Therefore, the major limitation of the study was that no isotherm or adsorption kinetics was studied. When the Pseudo first or second order studies was also not discussed. However, further studies will be conducted in future research to address these limitations
Availability of data and materials
The dataset generated and/or analyzed during the current study is available from the corresponding author on reasonable request.
Author contributions
Christiana Boahen: Conceptualized the research topic, and developed the objectives and methodology of the research.
Samuel Wiafe: He carried out both the Laboratory and Field Investigation as well as the Data Curation of the research work
Fredrick Owusu: He carried out Laboratory work and assisted in the writing of the manuscript.
Liang Bian: He participated in the Writing of the manuscript (Original Draft Preparation) as well as reviewed and edited the manuscript.
Acknowledgements
The authors are grateful to God for the opportunity given them to materialize their study and to the South West University of Science and Technology – China for the opportunity to conduct all experiments in their laboratory. Also, we appreciate all team members and individuals (especially Professor Liang Bian) who in one way or the other contributed greatly to the success of the study. Thank you and my God bless you all.
Disclosure statement
No potential conflict of interest was reported by the author(s).
Additional information
Funding
References
- AJ, E., Jr, Krenkel, P., & Shamas, J. (2015). Wastewater treatment &water reclamation. Earth Systems and Environmental Sciences, PMCID: PMC7158167, B978-0-12-409548-9.09508–17. https://doi.org/10.1016/B978-0-12-409548-9.09508-7
- Akpor, O. B., Ohiobor, G. O., & Olaolu, T. D. (2014). Heavy metal pollutants in wastewater effluents: Sources, effects and remediation 2. Sources of Heavy Metals in, 2(4), 37–43. https://doi.org/10.11648/j.abb.20140204.11
- Alemdar, A., Güngör, N., Ece, O. I., & Atici, O. (2015). The rheological properties and characterization of bentonite dispersions in the presence of non-ionic polymer PEG. Journal of Materials Science, 40(1), 171–177. https://doi.org/10.1007/s10853-005-5703-4
- Ali, M. M., Hossain, D., Al-Imran, A., Khan, M. S., Begum, M., & Osman, M. H. (2021). Environmental pollution with heavy metals: A public health concern. In M. K. Nazal & H. Zhao (Eds.), Heavy Metals - Their Environmental Impacts and Mitigation (pp. 771–783). IntechOpen. https://doi.org/10.5772/intechopen.96805
- Anirudhan, T. S., Jalajamony, S., & Sreekumari, S. S. (2012). Adsorption of heavy metal ions from aqueous solutions by amine and carboxylate functionalized bentonite. Applied Clay Science, 65-66(65), 67–71. https://doi.org/10.1016/J.CLAY.2012.06.005
- Anna, B., Kleopas, M., Constantine, S., Anestis, F., & Maria, B. (2014). The adsorption of Cd(II), Cu(II), Ni(II) and Pb(II) onto natural bentonite: A study in mono- and multi-metal systems. Environmental Earth Sciences, 73(9), 5435–5444. https://doi.org/10.1007/S12665-014-3798-0
- Asiabi, H., Yamini, Y., & Shamsayei, M. (2017). highly selective and efficient removal of arsenic (V), chromium (VI) and selenium (VI) oxyanions by layered double hydroxide intercalated with zwitterionic glycine. Journal of Hazardous Materials, 339, 239–247. https://doi.org/10.1016/j.jhazmat.2017.06.042
- Ballah, J., Chamerois, M., Durand-Vidal, S., Malikova, N., Levitz, P., & Michot, L. J. (2016). Effect of chemical and geometrical parameters influencing the wettability of smectite clay films. Colloids and Surfaces A: Physicochemical and Engineering Aspects, 511, 255–263. https://doi.org/10.1016/j.colsurfa.2016.10.002
- Bogardi, J. J., Leentvaar, J., & Sebesvári, Z. B. F. (2020). Integrating freshwater ecosystem health in water resources management. BIOLOGIA FUTURA, 71(4), 337–358. https://doi.org/10.1007/s42977-020-00031-7
- Carnoyale, G., & Cuadra, J. (2004). The Brauer group of the dihedral group. Glasgow Mathematical Journal, 46(2), 239–257.
- Chen, C., Liu, H., Chen, T., Chen, D., & Frost, R. L. (2015). Insights into the removal of Pb(II. Cu(II), Co(II), Cd(II), Zn(II), Ag(I), Hg(I), Cr(VI) by Na(I)-montmorillonite and Ca(II)-montmorillonite. Applied Clay Science, 118, 239–247. https://doi.org/10.1016/J.CLAY.2015.09.004
- Chen, M., Zhang, H., Liu, W., Zhang, W., & Montoya, A. R. H. (2020). The global pattern of urbanization and economic growth: evidence from the last three decades. PLoS ONE, 9(8), e103799. https://doi.org/10.1371/journal.pone.0103799
- Duncan, A. E., de Varies, N., & Nyarko, K. B. (2018). Assessment of heavy metal Pollution in the main Pra River and its tributaries in the Pra Basin of Ghana. Environmental Nanotechnolgy, Monitoring & Management, 10, 264–271.
- Edokpayi, J. N., Odiyo, J. O., & Durowoju, O. S. (2017) Impact of wastewater on surface water quality in developing countries: a case study of South Africa Ed. Water Quality (pp. 431–443). IntechOpen .https://doi.org/10.5772/66561
- El Adraa, K., Georgelin, T., Lambert, J. F., Jaber, F., Tielens, F., & Jaber, M. (2017). Cysteine-montmorillonite composites for heavy metal cation complexation: A combined experimental and theoretical study. Chemical Engineering Journal, 314, 406–417. https://doi.org/10.1016/j.cej.2016.11.160
- Faghihian, H., & Nejati-Yazdinejad, M. (2009). The equilibrium study of the adsorption of L-cysteine by natural bentonite. Clay Minerals, 44(1), 125–133. https://doi.org/10.1180/claymin.2009.044.1.125
- Gil, A., Santamaría, L., Korili, S. A., Vicente, M. A., Barbosa, L. V., de Souza, S. D., Marçal, L., de Faria, E. H., & Ciuffi, K. J. (2021). A review of organic-inorganic hybrid clay-based adsorbents for contaminant removal: Synthesis, perspective, and applications. Journal of Environmental Chemical Engineering, 9(5), 105808. https://doi.org/10.1016/J.JECE.2021.105808
- Gupta, A., Sharma, V., Sharma, K., Kumar, V, Choudhary, S., Mankotia, P., Kumar, B., Mishra, H., Moulick, A., Ekielski, A., & Mishra, P. K. (2021). materials A review of adsorbents for heavy metal decontamination: growing approach to wastewater treatment. https://doi.org/10.3390/ma14164702
- Heiba, H. F., Taha, A. A., Mostafa, A. R., Mohamed, L. A., & Fahmy, M. A. (2018). Synthesis and characterization of CMC/MMT nanocomposite for Cu2+ sequestration in wastewater treatment. Korean Journal of Chemical Engineering, 35(9), 1844–1853. https://doi.org/10.1007/s11814-018-0096-7
- Hensen, E. J. M., & Smit, B. (2002). Why clays swell. Journal of Physical Chemistry B, 106(49), 12664–12667. https://doi.org/10.1021/jp0264883
- Kitadai, N., Yokoyama, T., & Nakashima, S. (2009). Journal of colloid and interface science in situ ATR-IR investigation of L -lysine adsorption on montmorillonite. Journal of Colloid and Interface Science, 338(2), 395–401. https://doi.org/10.1016/j.jcis.2009.06.061
- Kollár, T., Pálinkó, I., Kónya, Z., & Kiricsi, I. (2003). Intercalating amino acid guests into the montmorillonite host. Journal of Molecular Structure, 651-653(651), 335–340. https://doi.org/10.1016/S0022-2860(03)00109–1
- Kurniawan, T. A., Chan, G. Y. S., Lo, W. H., & Babel, S. (2006). Comparison of low-cost adsorbents for treating wastewater laden with heavy metals. Science of the Total Environment, 366(2–3), 409–426.
- Liu, Z., Sun, Y., Xu, X., Qu, J., & Qu, B. (2020). The adsorption of Hg(II) in an Aqueous Solution by Activated Carbon Prepared from Rice Husk Using KOH Activation. ACS Omega, 5(45), 29231–29242. https://doi.org/10.1021/acsomega.0c03992
- Mishra, P. C., & Patel, R. K. (2009). The removal of lead and zinc ions from water by low-cost adsorbents. Journal of Hazardous Materials, 168(1), 319–325.
- Peng, J., Yi, H., Song, S., Zhan, W., & Zhao, Y. (2019, November). Driving force for the swelling of montmorillonite as affected by surface charge and exchangeable cations: A molecular dynamic study. Results in Physics, 12, 113–117. https://doi.org/10.1016/j.rinp.2018.11.011
- Rashed, M. N. (2013). Adsorption technique for the removal of organic pollutants from water and wastewater. Organic Pollutants - Monitoring, Risk and Treatment. https://doi.org/10.5772/54048
- Sen, T. K., & Gomez, D. (2011). Adsorption of zinc (Zn2+) from aqueous solution on natural bentonite. Desalination, 267(2–3), 286–294. https://doi.org/10.1016/j.desal.2010.09.041
- Sen Gupta, S., & Bhattacharyya, K. G. (2012). Adsorption of heavy metals on kaolinite and montmorillonite: A review. Physical Chemistry Chemical Physics, 14(19), 6698–6723. https://doi.org/10.1039/c2cp40093f
- Taha, Y., Benzaazoua, M., Hakkou, R., & Mansori, M. (2016). Natural clay substitution by calamine processing wastes to manufacture fired bricks. Journal of Cleaner Production, 135, 847–858. https://doi.org/10.1016/j.jclepro.2016.06.200
- Tovar-Gómez, R., Moreno-Virgen, M., del, R., Moreno-Pérez, J., Bonilla-Petriciolet, A., Hernández-Montoya, V., & Durán-Valle, C. J. (2015). Analysis of synergistic and antagonistic adsorption of heavy metals and acid blue 25 on activated carbon from ternary systems. Chemical Engineering Research and Design, 93, 755–772.
- Tutic, A., Novakovic, S., Lutovac, M., Biocanin, R., Ketin, S., & Omerovic, N. (2015). The heavy metals in agrosystems and their impact on their health and quality of life. DOOEL Skopje Open Access Macedonian Journal of Medical Sciences, 3(2), 345–355. https://doi.org/10.3889/oamjms.2015.048
- Veli, S., & Alyüz, B. (2007). Adsorption of copper and zinc from aqueous solutions using natural clay. Journal of Hazardous Materials, 149(1), 226–233. https://doi.org/10.1016/j.jhazmat.2007.04.109
- Viseras, C., Aguzzi, C., Cerezo, P., & Lopez-Galindo, A. (2007). Uses of clay minerals in semisolid health care and therapeutic products. Applied Clay Science, 36(1–3), 37–50. https://doi.org/10.1016/j.clay.2006.07.006
- Xie, S., Yu, C., Peng, B., Xiao, H., Zhang, W., Zhou, Z., & Åström, M. E. (2022). A re-assessment of metal pollution in the Dexing mining area in Jiangxi province, China: Current status, hydro-geochemical controls, and effectiveness of remediation practices. International Journal of Environmental Science and Technology, 19(11), 10707–10722. https://doi.org/10.1007/s13762-021-03887-x
- Yang, S., Zhao, D., Zhang, H., Lu, S., Chen, L., & Yu, X. (2010). The impact of environmental conditions on the sorption behavior of Pb(II) in Na-bentonite suspensions. Journal of Hazardous Materials, 183(1–3), 632–640. https://doi.org/10.1016/j.jhazmat.2010.07.072
- Yildiz, N., Kapucu, H., & Çalimli, A. (2003). Adsorption of 4-toluene sulfonic acid on modified bentonite: Optimization by response surface methodology. Turkish Journal of Engineering and Environmental Sciences, 27(6), 397–407. https://www.acarindex.com/turkish-journal-of-engineering-and-environmental-sciences/adsorption-of-4-toluene-sulfonic-acid-on-modified-bentonite-optimization-by-response-surface-methodology-422616
- Yong, Y. C., & Ye, H. W. (2012). Removal of chromium (III) from aqueous solutions by adsorption on bentonite from Gaomiaozi, China. Iii. https://doi.org/10.1007/s12665-012-1569-3
- Zhu, S., Xia, M., Chu, Y., Khan, M. A., Lei, W., Wang, F., Muhmood, T., & Wang, A. (2019). Applied clay science adsorption and desorption of Pb (II) on L -Lysine Modi fi ed montmorillonite and the simulation of the Interlayer Structure. Applied Clay Science, 169(December 2018), 40–47.