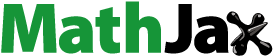
ABSTRACT
Land use change has led to drastic soil degradation and greenhouse gas emissions with implications for sustainable agriculture and climate change. Here, we report soil carbon stock and nutrient compositions of four land use types in the forest-savanna transition agro-ecological zone of Ghana. These were cashew, mango, oil palm and arable land. Soil samples were collected at 0–15 cm and 15–30 cm depths from each land use type. Soil nutrients and organic carbon content were greater in the tree-based land use types than in the arable land. Total soil organic carbon (SOC) ranged from 1.71% under cashew to 1.12% under the palms at the 0–15 cm depth. Soil carbon stock under the cashew was 12.5% greater than that of mango (56 Mg C/ha), and 40% more than that under oil palm. However, active carbon or permanganate oxidizable carbon was greatest (~130 mg/kg) under mango and least (~92.6 mg/kg) in arable land in the surface soil. Overall, active carbon was dependent on soil total carbon in the land use types (r = 0.81–0.91). Soil microbial biomass carbon was least in arable land (p < 0.05) and similar among the tree-based systems (p > 0.05) at 0–15 cm depth. No clear trend was observed in the exchangeable base compositions in the surface soils but cashew and mango systems appeared to show significantly greater levels of exchangeable calcium and magnesium, respectively. We conclude that strong nexus between soil microbial biomass carbon, nitrogen and phosphorus, and active soil carbon may drive soil carbon dynamics in land use systems of tropical forest-savanna ecotone.
1. Introduction
Productivities of tropical cropping systems especially in sub-Saharan Africa (SSA) are threatened as a result of climate change and ecosystem degradation amidst increasing human population. Currently, one of the utmost environmental challenges confronting the region and other parts of the world is inappropriate land use and changes in land cover. Land use or alterations have unlimited influence on climate, land productivity, soil quality, food security and ecosystem services at large (Kang & Kanniah, Citation2022; Lambin et al., Citation2003). Although agriculture has been reported to contribute about 29% to greenhouse gas emissions (GHGs), with land-use changes accounting for 12–20% of human-induced GHG emissions, it also offers opportunities for reducing the emissions through improved land use management practices and carbon capture and storage (IPCC, Citation2007; Vermeulen et al., Citation2012). There is therefore, the need to consider suitable policies and strategies that make agriculture less detrimental (Lal, Citation2003).
Different agricultural land use systems have significant impact on soil properties through strong influence on nutrient availability and soil productivity (Aluko & Fagbenro, Citation2000; Liu et al., Citation2010; Pal et al., Citation2013). Carbon storage in soil organic matter is a vital strategy in climate change mitigation and adaptation as well as enhancing land productivity. However, soil organic carbon content is greatly influenced by different management practices (Leifeld et al., Citation2011) and the type of vegetation cover with losses in most ecosystems resulting in 10–15% yield reduction (Marks, Citation2019). According to Lasco and Pulhin (Citation2004), land use management and carbon pools are extensively linked. Improper land use and alterations therefore lead to global impact resulting in global warming, loss of biodiversity and desertification, rendering the soil one of the most vulnerable natural resources (FAO and Intergovernmental Technical Panel on Soils (ITPS), Citation2015). Consequently, this makes land-use and soil organic carbon management key components in the mitigation of carbon buildup in the atmosphere and global warming (Houghton, Citation2005; Logah et al., Citation2020).
Many countries over recent years have discussed a number of policies aimed towards mitigation of greenhouse gases. The use of carbon sink as a means of offsetting emission was one of the important schemes, among others (UNFCCC, Citation1997). International programs and protocols such as the clean development mechanisms and the REDD+ (reducing emissions from deforestation and degradation) strategies have been established with the help of the United Framework Convention on Climate Change (UNFCCC) to look into land-use systems and climate change influences on mitigation and support carbon sequestration and reduction of greenhouse gases resulting from land-use systems (Dayamba et al., Citation2016; DERM, Citation2011).
The forest-savanna transition agro-ecological zone of Ghana, with mean annual rainfall amount of 1300 mm and a bimodal rainfall regime, presents farmers with opportunities to cultivate key arable and tree crops. Thus, cashew, oil palm and mango are predominantly cultivated in the zone mostly on marginal lands in addition to arable crops. However, most soil carbon inventory studies of land use or vegetation in SSA have focused extensively either on the forest agro-ecologies (e.g. Logah et al., Citation2020; Anokye et al., Citation2021, etc.) or the Guinea and Sudan savannas (Djagbletey et al., Citation2018; Hounkpatin et al., Citation2018; Saiz et al., Citation2012) with less emphasis on the forest-savanna ecotone. Thus, there is less documented contribution of the forest-savanna ecotone in SSA to the global carbon budget. This study, therefore, evaluates comparatively soil carbon stock, labile carbon pools and selected soil fertility indicators of four land-use types in a less studied forest-savanna ecotone of West Africa.
2. Materials and methods
2.1. Experimental site
The study was conducted in the Kintampo metropolitan area of Ghana. Kintampo serves as a transit point between Ghana’s southern and northern parts (Bessah, Citation2014). It falls within the forest-savanna transition zone of Ghana, characterized by a bimodal rainfall pattern. The average rainfall is about 1,300 mm/year. The major raining season starts from Mid-March to July, followed by a short dry spell in August and a minor season spanning from September to mid-November (SEA, Citation2010).
2.2. Selection of land use types and plot layout
We selected three land use types of economically cultivated agricultural/cash crops, viz cashew, mango and oil palm at similar ages of establishment within the study area. As a control, we used a nearby arable land under cultivation of maize for 5 years. The arable land (8.1704° N, 001.5642° W) benefitted from mineral NPK fertilizer applications over the period but without direct organic fertilizer inputs. Farmers in the area plant maize twice a year at two seeds per hill at average planting distance of ca. 75 × 40 cm. The cashew farm lies on latitude 8.1762° N and longitude 1.5693° W and has been under cultivation for 17 years and the mango (8.1294° N, 001.6981° W) for 15 years. Oil palm lies on latitude 8.1761° N and longitude 1.5631° W being under cultivation for 19 years.
The tree-based systems (viz. cashew, mango and palm) benefitted from periodic agronomic management practices including pruning, manual weeding and pest control. Apart from natural leaf litter fall and accumulation, pruning activities in the tree-based systems added organic matter to the soils. The soils of the land-use types are generally Ferric Lixisol. In each land use, a plot measuring 40 m × 40 m was demarcated, which was subdivided into four 20 × 20 m subplots or replicates.
2.3. Soil sampling
Soil samples were taken at two different depths (0–15 cm and 15–30 cm) with the help of an auger. On each of the 20 × 20 m subplots, five soil cores were taken from four georeferenced points using the Y-shape design. These were then bulked and thoroughly mixed together to obtain one composite sample for each individual subplot and depth. A total of 10 soil samples (i.e. five samples per depth) were obtained for each land-use type, making an overall total of 40 composite samples.
Part of the fresh soil samples were kept in the refrigerator at 4°C for microbial analysis whilst the remaining of each sample was air-dried, ground and sieved through a 2 mm mesh for chemical analysis. Bulk density soil samples were taken at both depths using cylindrical cores (Blake & Hartge, Citation1986) at five points in each land use type.
2.4. Soil analysis
All laboratory analysis was carried out following standard protocols in the Soil Science Laboratory of the Department of Crop and Soil Sciences, Kwame Nkrumah University of Science Technology, Kumasi, Ghana. Soil pH was determined using a PHS-3E 510 pH meter in 1:2.5 soil–water ratio. The hydrometer method as described by Anderson and Ingram (Citation1993) was used in determining particle size distribution. Soil moisture content was measured using the gravimetric method (Allen, Citation1989), and bulk density was determined by oven drying soil samples at 105 °C for 24 hr.
The chloroform fumigation and extraction method as described by Ladd and Amato (Citation1989) was employed in determining the soil microbial biomass C, N and P. Organic carbon and nitrogen were determined using the elemental analyzer (Vario Macro cube, Germany), while available P was extracted by the Bray -1 method (Bray & Kurtz, Citation1945). Exchangeable bases were extracted with 1.0 N ammonium acetate. Subsequently, the EDTA (Ethylenediaminetetraacetic acid) titration method was used to determine exchangeable magnesium and calcium, and the flame photometry procedure for sodium and potassium (Mclean, Citation1965). Hydrogen and aluminum were also extracted using 1.0 N KCl (Mclean, Citation1965). Active soil carbon was determined following the procedure of Culman et al. (Citation2021).
2.5. Estimation of soil carbon stocks
Soil organic carbon (SOC) stock was estimated using the method described by Donovan (Citation2012) as follows:
BD = Bulk density
V = Volume of soil
SOC = Soil organic carbon concentrations (divided by 100)
2.6. Statistical analysis
All data collected were subjected to one-way analysis of variance (ANOVA) using R statistical package (version 4.1.3). Differences in means were separated using Tukey HSD at 5% probability. Pearson’s correlation and regression analysis were also performed to determine the relationships between key soil parameters.
3. Results
3.1. Soil physical properties of the land use types
Soil particle size distribution varied significantly among the land-use types at both depths of sampling (Figures ). Mango land use had the highest sand fractions of 88.7 and 85.34% at 0–15 and 15–30 cm, respectively, whereas the least fractions were observed under cashew and oil palm (Figure ). Conversely, the clay fractions (Figure ) under cashew at both depths (13.23 and 12.62%) were significantly (p < 0.05) greater than fractions recorded in mango (7.66 and 9.37%) and arable fields (9.93 and 10.43%). The clay fraction decreased in the order: cashew > oil palm > arable land > mango across depths. Significant differences (p < 0.05) were observed in average silt content in the land use types irrespective of sampling depths (Figure ). Soil texture was loamy sand in the top 0–15 cm and 15–30 cm depth across all the land-use systems.
Figure 1. Sand particle distribution in the land use types. Bars with different alphabets are significantly different from one another (Tukey HSD, p<0.05).

Figure 2. Silt particle distribution in the land use types. Bars with different alphabets are significantly different from one another (Tukey HSD, p<0.05).

Figure 3. Clay particle distribution in the land use types. Bars with different alphabets are significantly different from one another (Tukey HSD, p<0.05).

Soil bulk density varied significantly (p = 0.016) among the land use types and depths (Table ). In the top 0–15 cm depth, soils under cashew had the lowest bulk density (p = 0.004). Bulk density increased from the arable land < oil palm < mango in the surface soil (Table ). Bulk density in the 15–30 cm depth was significantly lower in the cashew and arable land than in the oil palm. Furthermore, bulk density significantly increased with increasing soil depth in all the land use types. Our results showed that soil moisture content decreased with increasing depth across the land use types. Soil moisture content of cashew land use was greater than that of the oil palm and arable land in the subsoil (Table ).
Table 1. Variations of soil properties under land use systems in the forest-savanna transition zone of Ghana
3.2. Soil chemical properties
The pH of soils among the land use systems was slightly acidic, ranging from 6.12 to 6.35 (Table ). There were no significant differences in pH among the various land-use systems in both depths sampled. Soil total nitrogen content at the 0–15 cm depth decreased from 0.13% under cashew to 0.09% in arable field. Total nitrogen content in oil palm field was significantly (p < 0.05) greater than that of the arable land in the subsoil (Table ). Generally, we observed significant differences (p < 0.05) in soil exchangeable cations (Ca, Mg, Na, H, Al) and ECEC among the land-use types only in the 15–30 cm depth. The highest exchangeable cations (Ca and Mg) and ECEC were recorded under cashew and the least in oil palm field at both depths. Exchangeable Na and H content were higher in mango field than all land use types in the 15–30 cm depth.
3.3. SOC, C stock, active carbon and microbial biomass pools of the land use types
The SOC varied significantly (p = 0.003) across the land use types and depths. Cashew land had the highest SOC content of 1.71%, which was 53 and 39% greater than values observed in the oil palm and arable land, respectively. Soil organic carbon stocks ranged from 36 Mg/ha in cashew field to 26 Mg/ha under oil palm at 0–15 cm depth with the latter being consistently lower (18 Mg/ha) at the 15–30 cm depth (Figure ). Soil carbon stocks decreased in the order: cashew > mango > arable field > oil palm at both depths. The active carbon measured as permanganate oxidizable carbon (POXC) varied significantly (p = 0.001) among the land use types. It ranged from ~130 mg/kg in mango field to ~92.6 mg/kg in the arable land at the 0–15 cm depth (Figure ). Conversely, we did not observed differences (p = 0.133) in the subsoil (15–30 cm).
Figure 4. Soil carbon stock of land use system at the 0–15 and 15–30 cm depth. Bars with different alphabets are significantly different from one another (Tukey HSD, p<0.05).

Figure 5. Active carbon pool under land use types at 0–15 and 15–30 cm depths. Bars with different alphabets are significantly different from one another (Tukey HSD, p<0.05).

Soil microbial biomass carbon decreased among the land use types in the order: cashew (197.6 mg/kg) > oil palm (182.7 mg/kg) > mango (181.1 mg/kg) > arable land (142.8 mg/kg) at the top 0–15 cm depth (Figure ). However, values were similar (p > 0.05) among the tree-based systems. Comparatively, the mango and cashew fields had higher (p = 0.002) microbial biomass C (104.71 and 95.50 mg/kg, respectively) in the subsoil, which were both 30% more than for the arable land (Figure ). The cashew had higher microbial biomass N (p = 0.002; Figure ) than all the land use types in the surface soil. Microbial biomass phosphorus was significantly (p = 0.001) higher in the mango field (16.47 mg/kg soil), being 35 and 37% more than in the arable land (10.42 mg/kg) and the oil palm (10.77 mg/kg soil), respectively, at the 0–15 cm depth. A similar trend was observed in the subsoil (15–30 cm) with the mango farm being 23 and 26% higher (p = 0.011) in biomass phosphorus than arable land and oil palm systems, respectively (Figure ).
Figure 6. Soil microbial biomass carbon in the land use types at the 0–15 and 15–30 cm depth. Bars with different alphabets are significantly different from one another (Tukey HSD, p<0.05).

3.4. Relationship between soil properties
We observed significant and strong positive relationship between soil microbial carbon and active carbon pool (r = 0.883, Table ). The active carbon and microbial biomass carbon showed positive correlations with soil organic carbon. There was a weak correlation (although significant) between available P and microbial biomass P across land use types and soil depths. Soil organic carbon (OC) had a moderate and significant positive relationship with ECEC (p ≤ 0.001; r = 0.646, Table ). Similarly, soil available P showed moderate and significant positive correlations with ECEC (r = 0.509). Soil pH weakly correlated with soil variables in the land use systems.
Table 2. Pearson correlation analysis on selected soil properties and microbial biomass properties
4. Discussion
4.1. Soil physical properties under the land use types
We observed variations in soil physical properties (viz. bulk density and soil moisture content) among the land use types (Table ), which is consistent with earlier studies (Malik et al., Citation2018; Purswani & Pathak, Citation2017). These differences are attributable to management practices under the land use systems (Bationo et al., Citation2006; Murty et al., Citation2002) in addition to plant characteristics, leading to differential inputs of soil organic matter (Bougma et al., Citation2022; Mesele et al., Citation2023), which modulates these properties. Thus, bulk density, a proxy for soil compaction (Ocloo et al., Citation2014) increased with depth in all instances (Table ) due to the decline in soil organic carbon with depth (Bessah, Citation2014; Grüneberg et al., Citation2014). Similarly, the lower bulk densities observed under cashew trees may be due to its relatively higher organic carbon content (Table ). Though soil moisture content was similar in the surface soils (Table ), a somewhat marked variation (p < 0.05) was observed in the subsoil among the land use types, being greater under cashew.
4.2. Soil chemical properties of the land use types
Soil pH was slightly acidic (see Landon, Citation1996) indicating the suitability of the study area for wide range of agricultural crops. According to Mostara and Roy (Citation2008), most agricultural soils are productive with good nutrient availability when soil pH is within the range of 5.5 and 6.5. The soil total N in the topsoil of the arable land (<0.1%) was low (see Landon, Citation1996) while all other land use types had moderate amounts of the nutrient. The low N levels of the arable land may be due to frequent tillage activity, leading to faster decomposition of organic matter under high tropical temperatures. Nitrogen has been reported as a key-limiting nutrient to plant growth in most Ghanaian soils (Bationo et al., Citation2018).
Although soil available P contents did not differ among the land use types, the concentrations were high (>20 mg/kg) as per the ratings of CSIR-SRI (Citation2007), with arable land and oil palm recording the least values, which could be explained by their relatively lower SOC contents (Table ). Conversely, some studies reported higher P content in arable fields despite low organic carbon content, which has been attributed to fertilizer applications and the fact that the bulk of P fertilizers applied remain in the soil (Power & Prasad, Citation1997).
In general, exchangeable Ca, Mg and ECEC were relatively higher under cashew, which had relatively greater organic matter content, confirming the significant positive correlation (r = 0.691) observed between ECEC and OC (Table ).
4.3. Soil carbon stock, active carbon and microbial biomass carbon
Land use type influences soil organic carbon stock, turnover rate and soil microbial biome (Vieira et al., Citation2007) with significant impact on ecosystem sustainability. Soil organic carbon contents of all the land use types were moderate (1–2%) in the topsoil and low (<1%) in the sub soil as per the ratings of Boerma et al. (Citation1995). We observed higher soil organic carbon under tree crops-cashew and mango than the arable land, which may be due to accumulation of leaf litter and its decomposition to release soil organic carbon in the tree-based systems. The low SOC in arable land is ascribable to tillage practices, which exposes SOC through layer inversion to microbial attack leading to higher rate of decomposition. It also depicts the generally low organic carbon content of the study area, which has been reported to be ca. 1.0% or less (Bationo et al., Citation2018; Logah et al., Citation2011).
Higher rates of decomposition and mineralization of organic matter with soil depth (Maitima et al., Citation2009) may explain the decline of SOC at the 15–30 cm depth (Table ). Earlier studies also reported decreases in SOC with depth across various land uses (Agboadoh, Citation2011; Bessah, Citation2014) with the extent and direction of influence due to land use types (Lal, Citation2015), soil moisture and biomass quality and quantity returned to the soil (Offiong et al., Citation2009). Management practices employed by farmers, climate and land cover have greater influence on topsoil carbon (Chen et al., Citation2018).
Land use type and management practices towards soil organic carbon sequestration are key components in mitigating global warming through reduction in carbon buildup in the atmosphere (Houghton, Citation2005; Logah et al., Citation2020). Soil carbon stocks in the top 0–15 cm depth ranged from 36 Mg/ha under cashew to 26 Mg/ha under oil palm with the latter being consistently lower (18 Mg/ha) in the 15–30 cm depth. Our results were similar to the findings of Dowuona and Adjetey (Citation2010) who reported SOC stocks as ranging from 18.0 to 44.89 Mg/ha and 20.89 to 52.54 Mg/ha in 2007 and 2009, respectively, although in the interior savanna zone of Ghana. The greater SOC content under cashew led to its greater SOC stocks (Table ), contradicting the findings of Bessah (Citation2014) who reported the contrary (lower SOC in cashew fields and higher SOC in arable fields). The observation, however, agrees with that of Boakye et al. (Citation2014) who reported lower SOC stock in arable fields due to complete removal of crop residues and low application of organic or inorganic fertilizers. In their study, Anderson et al. (Citation2009) reported significant impact of land-use conversion on SOC stock amounts. However, other authors reported no significant variation in SOC stocks under different land-use systems (Dawoe, Citation2009; Yao et al., Citation2010).
The active carbon pool, which is a small proportion of total soil organic matter, undergoes rapid turnover due to its availability to soil microbial decomposition and responds to changes in management and agronomic practices (Lal, Citation2002). Though cashew had greater total soil carbon than mango, its active carbon pool was smaller than that of mango, but overall soil active carbon shows positive correlations with soil total carbon in the land use types (Table ). Soils with higher active carbon pool may correlate to crop productivity (Lal, Citation2006). Haynes (Citation2005) reported that the most sensitive portion of SOM pool available relatively in small proportion is the labile pool (active pool), which is easily affected by fluctuation in environmental conditions and rapidly oxidizes easily with changes in land use practice. The non-labile pool also known as the passive pool being a more stable form of SOM decomposes slowly by microbial activities (Wiesenberg et al., Citation2010). We also observed variations in soil microbial biomass C, N and P among the land use types (Figures ). The consistently greater soil microbial biomass C and N pools under cashew is an indication of higher microbial activity with direct impact on soil carbon sequestration (Post & Kwon, Citation2000). There were stronger and significant correlations (r = 0.81–0.91) between soil microbial biomass C, N, P and active soil carbon, signifying the nexus thereof in driving soil carbon dynamics in the land use systems.
5. Conclusion
The study shows the relevance of tree-based land use types in enhancing soil carbon storage in tropical forest-savanna ecotone. Though cashew had greater total soil carbon than mango, its active carbon pool was smaller than that of mango, but overall soil active carbon was dependent on soil total carbon in the land use types. Strong nexus between soil microbial biomass C, N, P and active soil carbon may drive soil carbon dynamics in land use systems of the forest-savanna ecotone.
Disclosure statement
No potential conflict of interest was reported by the author(s).
Data availability statement
Data sharing is not applicable to this article as no new data were created in this study.
Additional information
Funding
References
- Agboadoh, M. D. Y. (2011). Soil Organic Carbon Stocks in Croplands of the Bechem Forest Municipal, Ghana. MSc Thesis Submitted to the Faculty of GeoInformation Science and Earth Observation of the University of Twente, Netherland and the Faculty of Renewable Natural Resources of the Kwame Nkrumah University of Science and Technology,
- Allen, S. E. (1989). Chemical analysis of ecological materials. Blackwell Scientific Publications.
- Aluko, A. P., & Fagbenro, J. A. (2000). The role of tree species and land-use systems in organic matter and nutrient availability in degraded ultisol of Onne, Southeastern Nigeria. Proceedings of the 26th Annual Conference Soil Science, Ibadan, Nigeria (pp. 220–12).
- Anderson, B. J., Armsworth, P. R., Eigenbrod, F., Thomas, C. D. G., Heinemeyer, S., Roy, A., D. B., & Gaston, K. J. (2009). Spatial covariance between biodiversity and other ecosystem service priorities. The Journal of Applied Ecology, 46(4), 888–896. https://doi.org/10.1111/j.1365-2664.2009.01666.x
- Anderson, J. M., & Ingram, J. S. I. (1993). A handbook of methods. In CAB International (Vol. 221, pp. 62–65). Wallingford, Oxfordshire. https://doi.org/10.2307/2261129
- Anokye, J., Logah, V., & Opuku, A. (2021). Soil carbon stock and emission: Estimates from three land-use systems in Ghana. Ecological Processes, 10(1), 1–13. https://doi.org/10.1186/s13717-020-00279-w
- Bationo, A., Fening, J. O., & Kwaw, A. (2018). Assessment of Soil Fertility Status and Integrated Soil Fertility Management in Ghana. In A. Bationo, D. Ngaradoum, S. Youl, F. Lompo, & J. Fening(Eds.), Improving the Profitability, Sustainability and Efficiency of Nutrients Through Site Specific Fertilizer Recommendations in West Africa Agro-Ecosystems. Springer. https://doi.org/10.1007/978-3-319-58789-9_7
- Bationo, A., Hartemink, A. E., Lungu, O., Naimi, M., Okoth, P. F., Smaling, E. M. A., & Thiombiano, L. (2006). African soils: Their productivity and profitability of fertilizer use. Proceedings of the African Ferilizer Summit, 9-13 June, Abuja, Nigeria.
- Bessah, E. (2014). Assessment of soil organic carbon stocks under various land use/land cover types in the Kintampo North Municipal, Ghana. MTech Thesis, Department of Geography, Federal University of Technology,
- Blake, G. R., & Hartge, K. H. (1986). Bulk density. In A. Klute (Ed.), Methods of soil analysis. Part 1. Physical and mineralogical methods (2nd ed., pp. 363–375). American Society of Agronomy and Soil Science Society of America.
- Boakye, D. J., Antwi, E. K., Saito, O., Abekoe, M. K., & Takeuchi, K. (2014). Impact of farm management practices and agricultural land use on soil organic carbon storage potential in the savannah ecological zone of Northern Ghana. Journal of Disaster Research, 9(4), 484–500. https://doi.org/10.20965/jdr.2014.p0484
- Boerma, J. A. K., Guobao, L., & Huang, B. (1995). People's Republic of China: Reference soils of Songnen Plain Heilongjiang Province. Soil Brief CN 12. Institute of Soil Science- Academica Sinica, Nanjing and International Soil Reference and Information Centre.
- Bougma, A. B., Ouattara, K., Compaore, H., Nacro, H. B., Melenya, C., Mesele, S. A., Logah, V., Azeez, J. O., Veenendaal, E., & Lloyd, J. (2022). Soil aggregate stability of forest islands and adjacent ecosystems in West Africa. Plant and Soil, 473(1–2), 533–546. https://doi.org/10.1007/s11104-022-05302-x
- Bray, R. H., & Kurtz, L. T. (1945). Determination of total, organic, and available forms of phosphorus in soils. Soil Science, 59(1), 39–46. https://doi.org/10.1097/00010694-194501000-00006
- Chen, S., Manuel, M. P., Saby, N. P. A., Wlater, C., Angers, D. A., & Arrouys, D. (2018). Fine resolution map of top-and subsoil carbon sequestration potential in France. The Science of the Total Environment, 630, 389–400. https://doi.org/10.1016/j.scitotenv.2018.02.209
- Council for Scientific and Industrial Research-Soil Research Institute (CSIR-SRI). (2007). Soil nutrient (mineral) content factsheet.
- Culman, S. W., Hurisso, T. T., & Wade, J. (2021). Permanganate oxidizable carbon: An indicator of biologically active soil carbon. In Karlen, D. L., Stott, D. E. & Mikha, M. M. (Eds.), Laboratory Methods for Soil Health Analysis (pp. 152–175). ASA, ASSA. 9780891189831. https://doi.org/10.1002/9780891189831.ch9
- Dawoe, E. (2009). Conversion of Natural Forest to Cocoa Agroforest in Low-land Humid Ghana: Impact on plant biomass production, organic carbon and nutrient dynamics ( PhD Thesis). Department of Agroforestry, Faculty of Renewable Natural Resources, Kwame Nkrumah University of Science and Technology,
- Dayamba, S. D., Djoudi, H., Zida, M., Sawadogo, L., & Verchot, L. (2016). Biodiversity and carbon stocks in different land-use types in the sudanian zone of Burkina Faso, West Africa. Agriculture, Ecosystems and Environment, 216, 61–72. https://doi.org/10.1016/jagee.2015.09.023
- Department of Environment and Resource Management (DERM). (2011). Climate change: Adaptation for Queensland. State of Queensland, Office of Climate Change, Issue Paper Government,
- Djagbletey, E. D., Logah, V., Ewusi-Mensah, N., & Tuffour, H. O. (2018). Carbon stocks in the Guinea savanna of Ghana: Estimates from three protected areas. Biotropica, 50(2), 225–233. https://doi.org/10.1111/btp.12529
- Donovan, P. (2012). Measuring soil carbon change. A flexible, practical, local method. Retrieved February 13, 2012 https://soilcarboncoalition.org/files/MeasuringSoilCarbonChange.pdf.
- Dowuona, G. N. N., & Adjetey, E. T. (2010). Assessment of carbon storage insome savanna soils under different land-use systems in Ghana. Proceedings of the ICID+18 International conference, Fortaleza, Brazil.DFID, UK, August 16-20.
- FAO ITPS. (2015). Status of the world’s soil resources (SWSR): Main report. Retrieved from http://www.fao.org/documents/card/en/c/c6814873-efc3-41db-b7d3-2081a10ede50/
- Grüneberg, E., Ziche, D., & Wellbrock, N. (2014). Organic carbon stocks and sequestration rates of forest soils in G ermany. Global Change Biology, 20(8), 2644–2662. https://doi.org/10.1111/gcb.12558
- Haynes, R. J. (2005). Labile organic matter fractions as central components of the quality of agricultural soils. Advances in Agronomy, 85, 221–268. https://doi.org/10.1016/S0065-2113(04)85005-3
- Houghton, R. A. (2005). Aboveground forest biomass and the global carbon balance. Global Change Biology, 11(6), 945–958. https://doi.org/10.1111/j.1365-2486.2005.00955
- Hounkpatin, O. K. L., Hipt, F. O., Bossa, A. Y., Welp, G., & Amelung, W. (2018). Soil organic carbon stocks and their determining factors in the dano catchment (Southwest Burkina Faso). Catena, 166, 298–309. https://doi.org/10.1016/j.catena.2018.04.013
- IPCC. (2007). Climate change (2007) synthesis report. contribution of working group I, II, and III to the fourth assessment report of the Intergovernmental Panel on climate change (Pachauri, R. K. & Reisinger, A. Eds). Retrieved from. http://www.ipcc.ch.
- Kang, C. S., & Kanniah, K. D. (2022). Land use and land cover change and its impact on river morphology in Johor River Basin, Malaysia. Journal of Hydrology: Regional Studies, 41, 101072. https://doi.org/10.1016/j.ejrh.2022.101072
- Ladd, J. N., & Amato, M. (1989). Relationship between microbial biomass carbon in soils and absorbance (260 nm) of extracts of fumigated soils. Soil Biology and Biochemistry, 21(3), 457–459. https://doi.org/10.1016/0038-0717(89)90160-0
- Lal, R. (2002). Soil carbon sequestration in China through agricultural intensification, and restoration of degraded and desertified ecosystems. Land Degradation & Development, 13(6), 469–478. https://doi.org/10.1002/ldr.531
- Lal, R. (2003). Soil erosion and the global carbon budget. Environment International, 29(4), 437–450. https://doi.org/10.1016/S0160-4120(02)00192-7
- Lal, R. (2006). Enhancing crop yields in the developing countries through restoration of the soil organic carbon pool in agricultural lands. Land Degradation & Development, 17(2), 197–209. https://doi.org/10.1002/ldr.696
- Lal, R. (2015). Restoring soil quality to mitigate soil degradation. Sustainability, 7(5), 5875–5895. https://doi.org/10.3390/su7055875
- Lambin, E. F., Geist, H. J., & Lepers, E. (2003). Dynamics of land-use and land-cover change in tropical regions. Annual Review of Environment and Resources, 28(1), 205–241. https://doi.org/10.1146/annurev.energy.28.050302.105459
- Landon, J. R. (1996). Booker tropical soil Manual. A handbook for soil survey and agricultural land evaluation in the tropics and sub-tropics (Reprint ed.). Longman.
- Lasco, R. D., & Pulhin, F. B. (2004). Carbon budgets of tropical forest ecosystems in Southeast Asia: Implications for climate change. Forests for poverty reduction: Opportunities with clean Development mechanism, Environmental Services and biodiversity. FAO− RAP Publication.
- Leifeld, J., Ammann, C., Neftel, A., & Fuhrer, J. (2011). A comparison of repeated soil inventory and carbon flux budget to detect soil carbon stock changes after conversion from cropland to grasslands. Global Change Biology, 17(11), 3366–3375. https://doi.org/10.1111/j.1365-2486.2011.02471.x
- Liu, X. L., He, Y. Q., Zhang, H. L., Schroder, J. K., Li, C. L., Zhou, J., & Zhang, Z. Y. (2010). Impact of land use and soil fertility on distributions of soil aggregate fractions and some nutrients. Pedosphere, 20(5), 666–673. https://doi.org/10.1016/S1002-0160(10)60056-2
- Logah, V., Ewusi-Mensah, N., & Tetteh, F. K. M. (2011). Soil organic carbon and crop yield under different soil amendments and cropping systems in the semi-deciduous forest zone of Ghana. Journal of Plant Sciences, 6(4), 165–173. https://doi.org/10.3923/jps.2011.165.173
- Logah, V., Tetteh, E. N., Adegah, E. Y., Mawunyefia, J., Ofosu, E. A., & Asante, D. (2020). Soil carbon stock and nutrient characteristics of Senna siamea grove in the semi-deciduous forest zone of Ghana. Open Geosciences, 12(1), 443–451. https://doi.org/10.1515/geo-2020-0167
- Maitima, J. M., Mugatha, S. M., Reid, R. S., Gachimbi, L. N., Majule, A., Lyaruu, H., & Mugisha, S. (2009). The linkages between land-use change, land degradation and biodiversity across East Africa. African Journal of Environmental Science and Technology, 3(10), 310–325.
- Malik, A. A., Puissant, J., Buckeridge, K. M., Goodall, T., Jehmlich, N., Chowdhury, S., Gweon, H. S., Peyton, J. M., Mason, K. E., Van Agtmaal, M., Bland, A., Clar, I. M., Whitaker, J., Pywell, R. F., Ostle, N., Gleixner, G., & Griffiths, R. I. (2018). Land use driven change in soil pH affects microbial carbon cycling processes. Nature Communications, 9(1), 3591. https://doi.org/10.1038/s41467-018-05980-1
- Marks, J. C. (2019). Revisiting the fates of dead leaves that fall into streams. Annual Review of Ecology, Evolution, and Systematics, 50(1), 547–568. https://doi.org/10.1146/annurev-ecolsys-110218-024755
- Mclean, E. O. (1965). Aluminium. Methods of soil analysis. In C. Black (Ed.), America Soil Science Society. Agronomy Madison, Wisconsin. Agronomy no. 9, part 2 pp. 978–998. https://doi.org/10.2134/agronmonogr9.2.c16
- Mesele, S. A., Melenya, C., Bougma, A., Azeez, J. O., Ajiboye, G. A., Dubbin, W., Logah, V., Compaore, H., Veenendaal, E. M., & Lloyd, J. (2023). Soil mineralogical and nutrient characteristics of forest islands and surrounding ecosystem types in West Africa suggest anthropogenic soil improvement. Plant and Soil. https://doi.org/10.1007/s11104-023-06042-2
- Motsara, M., & Roy, R. N. (2008). Guide to Laboratory Establishment for Plant Nutrient Analysis. Food and Agriculture Organization of the United Nations.
- Murty, D., Kirschbaum, M., Mcmurtrie, E., & Mcgilvray, H. (2002). Does conversion of forest to agricultural land change soil carbon and nitrogen? A review of the literature. Global Change Biology, 8(2), 105–123. https://doi.org/10.1046/j.1354-1013.2001.00459.x
- Ocloo, C. Y., Quansah, C., Logah, V., & Amegashie, B. K. (2014). The impact of different levels of soil compaction on soil physical properties and root growth of maize and soybean seedlings. West African Journal of Applied Ecology, 22(2), 17–30.
- Offiong, R. A., Atu, J. E., Njar, G. N., & Iwara, A. I. (2009). Effects of land use change on soil Physico-chemical properties in a South-Southern Nigeria. African Journal of Environment, Pollution and Health, 7(2), 47–51.
- Pal, S., Panwar, P., & Bhardwaj, D. R. (2013). Soil quality under forest compared to other land uses in acid soil of North-Western Himalaya, India, Ann. For Research, 56(1), 187–19.
- Post, W. M., & Kwon, K. C. (2000). Soil carbon sequestration and land-use change: Processes and potential. Global Change Biology, 6(3), 317–327. https://doi.org/10.1046/j.1365-2486.2000.00308.x
- Power, J. F., & Prasad, R. (1997). Soil fertility management for sustainable agriculture. CRC Press.
- Purswani, E., & Pathak, B. (2017). Assessment of soil characteristics in different land-use systems in Gandhinagar, Gujarat. International Academy of Ecology and Environmental Sciences, 8(3), 162–17. 2018.
- Saiz, G., Bird, M. I., Domingues, T. F., Schrodt, F., Schwarz, M., Feldpausch, T. R., Veenendaal, E. M., Djagbletey, G., Hien, F., Compaore, H., Diallo, A., & Lloyd, J. (2012). Variation in soil carbon stocks and their determinants across a precipitation gradient in West Africa. Global Change Biology, 18(5), 1670–1683. https://doi.org/10.1111/j.1365-2486.2012.02657.x
- SEA. (2010). Kintampo Municipal Assembly, strategic Environmental Assessment (SEA). Environmental Protection Agency.
- UNFCCC. (1997). Kyoto protocol to the united nations framework convention on climate change. Document found at http://unfccc.int/resource/docs/convkp/kpeng.html.
- Vermeulen, S. J., Campbell, B. M., & Ingram, J. S. (2012). Climate change and food systems. Annual Review of Environment and Resources, 37(1), 195–222. https://doi.org/10.1146/annurev-environ-020411-130608
- Vieira, F. C. B., Bayer, C., Zanatta, J. A., Dieckow, J., Mielniczuk, J., & He, Z. L. (2007). Carbon management index based on physical fractionation of soil organic matter in an Acrisol under long-term no-till cropping systems. Soil and Tillage Research, 96(1–2), 195–204. https://doi.org/10.1016/j.still.2007.06.007
- Wiesenberg, G. L. B., Dorodnikov, M., & Kuzyakov, Y. (2010). Source determination of lipids in bulk soil and soil density fractions after four years of wheat cropping. Geodarma, 156(3–4), 267–277. https://doi.org/10.1016/j.geoderma.2010.02.026
- Yao, M. K., Angui, P. K. T., Konate, S., Tondoh, J. E., Tano, Y., Abbadie, L., & Bernest, D. (2010). Effects of land use types on soil organic carbon and nitrogen dynamics in mid-West Côte d’Ivoire. European Journal of Scientific Research, 40(2), 211–222.