ABSTRACT
Plastids are DNA-containing organelles responsible for various metabolic processes in plants, including photosynthesis. In a process called plastid inheritance, proplastids are transmitted from the parental to the filial generation through reproductive tissues. Plastid inheritance varies in different plant lineages and can be studied using reciprocal test crosses and genetic markers. Uniparental plastid inheritance is common in green algae and land plants, with mechanisms that eliminate plastids and their DNA either before or after fertilisation. Post-fertilisation elimination of mating type minus plastids in Chlamydomonas reinhardtii and paternal plastid elimination during male gametogenesis in Nicotiana tabacum are under nuclear control. In the genus Oenothera, however, biparental plastid inheritance may be followed by plastid-controlled elimination. Most angiosperms, including major crops, show dominant maternal inheritance, although some degree of transmission through the paternal parent, called leakage, appears to be universal. Exclusion of plastids from the pollen sperm cell provides a natural containment system for genetically modified plastid DNA in transplastomic plants, enabling diverse applications from insect resistance to pharmaceutical production. This review highlights recent findings and ongoing research in this area. Research into plastid inheritance and the exploitation of its full potential holds great promise for the advancements of agriculture.
Introduction
Plants, algae and cyanobacteria, use oxygenic photosynthesis and are the major producers of oxygen and organic matter on Earth. Oxygenic photosynthesis, one of the most important metabolic pathways to have evolved on Earth, was the basis for the emergence of complex life forms. In fact, algae and plants are descended from a lineage that arose from an endosymbiotic relationship between a protist and a cyanobacterium. The descendants of this ancient symbiotic cyanobacterium are the chloroplasts of green algae and embryophytes, and indeed, during the evolutionary transition from cyanobacteria to chloroplasts, the overall organisation and function of the photosynthetic machinery was retained. In contrast to green algae, embryophytes (also known as land plants) have evolved a number of different types of plastids, including chloroplasts. Plastids are remarkably dynamic, capable of interconversion in response to specific developmental and environmental cues, and several studies have provided evidence for a huge diversity of plastid subtypes with specific properties (Sierra et al. Citation2023), such as starch-containing amyloplasts or pigment-containing chromoplasts of fruits.
Plastids contain a genome (plastome or ptDNA) separate from that in the nucleus, making them semi-autonomous organelles. Compared to their cyanobacterial ancestors, plastid genomes have lost most of their genes, either definitively or through a significant amount of gene transfer to the nuclear genome. As a result of this endosymbiotic gene transfer, the proteome is a mosaic of nuclear and plastome-encoded proteins and is estimated to contain about 3000 proteins in Arabidopsis thaliana (Arabidopsis) chloroplasts (Fristedt Citation2017). To give an order of magnitude, plastid genomes encode only between 80 and 230 proteins compared to today’s cyanobacterial genomes, which encode between 1,800 and 12,000 proteins (Dagan et al. Citation2013). The first complete plastid genome sequences were published in 1986 from Nicotiana tabacum (tobacco) (Shinozaki et al. Citation1986) and Marchantia polymorpha (liverwort) (Ohyama et al. Citation1986), and since then more than 300 plastid genomes have been deposited in the National Center for Biotechnology Information (NCBI) organellar database. Plastid genomes have retained a core set of genes from their cyanobacterial ancestor, some of which encode thylakoid membrane proteins required for the light reactions of photosynthesis. Other plastid gene functions are related to transcription and translation, such as ribosomal RNAs, tRNAs, ribosomal proteins or subunits of plastid-encoded RNA polymerase (Green Citation2011; Wicke et al. Citation2011). The large subunit of ribulose-1,5-bisphosphate carboxylase/oxygenase (Rubisco) is also encoded in the plastid genome. However, all other genes required for carbon fixation reactions are located in the nuclear genome (Martin and Schnarrenberger Citation1997).
In plants, proplastids develop into chloroplasts with the characteristic thylakoid structure. Few proplastids are present in meristematic cells, whereas over 50 chloroplasts can be found in an Arabidopsis mesophyll cell, and over 100 chloroplasts per cell in tobacco leaves (Greiner et al. Citation2020). The number of plastome copies per plastid ranges from about 16 in meristematic tissue to about 90 in mature Arabidopsis leaves, so that the number of plastome copies reaches several thousand in a mature mesophyll cell (Greiner et al. Citation2020). Microscopic studies have shown that the plastomes are usually condensed into more or less distinct DNA regions (nucleoids) within the organelle stroma. During cell division, plastids must be duplicated, the plastid genomes replicated, and a subpopulation of plastids segregated into daughter cells (Chen et al. Citation2018). Plastids do not arise de novo and are therefore passed on as proplastids, including their plastomes, from one generation to the next during plant reproduction. More than 100 years ago, in 1909, two studies reported the non-Mendelian inheritance of chlorophyll deficiency in Mirabilis jalapa and Pelargonium zonale (Hagemann Citation2010) and the theory of plastid inheritance was subsequently developed. In many genera, proplastids are mainly transmitted uniparentally through the ovule, which is called maternal inheritance. In some genera, proplastids are transmitted through both gametes (sperm and egg cells), representing a biparental mode of inheritance, and others show paternal plastid inheritance.
Plastid inheritance is a complex process that plays a critical role in the survival and growth of plant and algal cells. Elucidating the mechanisms of plastid inheritance is part of developing new strategies to improve crop productivity and to understand the evolution of photosynthetic organisms. The study of plastid inheritance is a rapidly developing field and new discoveries have been made recently. The regulation of plastid inheritance is influenced by a complex network of interactions between the plastid, the nucleus and also by environmental factors. This review provides an overview and update on how plastids with their plastomes are inherited in plants, and studies from green algae provide insights into evolutionary aspects.
Mode of plastid inheritance
Important information on plastid inheritance in plants has been obtained from reciprocal test crosses involving ptDNA polymorphisms or mutations (). Mutations in the plastome are typically recognised by pale or bleached leaf areas in variegated plants (Park et al. Citation2023) and were first used by Correns and Baur (Hagemann Citation2010). Other plastid mutations provide selectable markers for antibiotic or herbicide resistance that are widely used in molecular biology and biotechnology. For example, naturally occurring mutations of the plastome-encoded psbA gene confer resistance to herbicides commonly used in agriculture (El-Lithy et al. Citation2005; Powles and Yu Citation2010; Matzrafi et al. Citation2014). Molecular markers derived from ptDNA and complete plastid genomes are popular for tracking plastid transmission in reciprocal crosses to support modern breeding programs, as in Cucumis sativus (cucumber) (Park et al. Citation2021), in the genus Populus (poplar) (Zhu et al. Citation2018) or in the genus Saccharum (sugarcane) (Zhu et al. Citation2014). One application of ptDNA markers, in combination with nuclear and/or mitochondrial markers, could be to determine the genetic purity of F1 seed material, thereby avoiding the traditional grow-out tests carried out by seed companies.
Table 1. Inheritance of plastids in species and genera mentioned throughout the text. The frequencies of transmission of plastids through leakage is given in percentages. n.d. not determined, n.a. not applicable.
In many branches of the phylogenetic tree, plastid transmission modes range from maternal to biparental to paternal inheritance (). Even within a genus, multiple modes of inheritance can be observed. A study of the genus Passiflora revealed clade-specific patterns of plastid inheritance in three subgenera, such as predominantly paternal plastid inheritance in the subgenera Astrophea and Passiflora, or maternal and biparental inheritance in the subgenus Decalabo (Shrestha et al. Citation2021). In the latter case, biparental inheritance was restricted to cotyledons and initial leaves, and only a single parental plastid genotype was detectable in mature plants (Shrestha et al. Citation2021). In many other angiosperms, maternal inheritance dominates over the others, cytological studies suggest that approximately 80% of all angiosperms show predominantly maternal inheritance (Zhang et al. Citation2003), and this assumption has been confirmed by many reciprocal test crosses in different species and genera (). Only a few species, such as Actinidia deliciosa (kiwi), have a paternal mode of inheritance (Cipriani et al. Citation1995) and some species, such as in the genus Medicago, show biparental inheritance (Matsushima et al. Citation2008; Dudas et al. Citation2012). In cryptogams, such as mosses and ferns, maternal inheritance is also the dominant mode (Gastony and Yatskievych Citation1992; Guillon and Raquin Citation2000; Natcheva and Cronberg Citation2007; Zhang and Sodmergen Citation2010; Kuo et al. Citation2018) although the variability is difficult to assess due to the limited number of species studied. In conifers, the largest and economically most important group of gymnosperms, including several species of the genera Picea and Pinus, paternal inheritance is the dominant mode of inheritance (Sutton et al. Citation1991; Dong and Wagner Citation1994).
In recent years, the assumption of strict maternal plastid inheritance in angiosperms has been revised because most species appear to exhibit some degree of leakage when sampled carefully enough. The variation in leakage rates is not well understood and the frequencies vary quantitatively and cover several orders of magnitude () in Arabidopsis (Schneider et al. Citation2015), Nicotiana sylvestris (Thyssen et al. Citation2012), tobacco (Ruf et al. Citation2007; Svab and Maliga Citation2007), Petunia hybrida (petunia) (Horn et al. Citation2017), Helianthus verticillatus (sunflower) (Ellis et al. Citation2008), Silene vulgaris (campion) (McCauley et al. Citation2007), Daucus carota (carrot) (Mandel et al. Citation2020), Setaria italica (foxtail millet) (Wang et al. Citation2004) and Lens culinaris (lentil) (McMurray et al. Citation2021). Similarly, paternal inheritance does not appear to be as strict in conifers either (Cato and Richardson Citation1996). Furthermore, the determination of paternal leakage depends on whether cotyledons or mature plants are examined. For example, is the paternal leakage rate into embryonic tissue higher (Azhagiri and Maliga Citation2007) than into a mature Arabidopsis plant, where the paternal plastids must have entered the meristematic cells first (Schneider et al. Citation2015).
The mechanisms by which maternal plastid transmission is achieved in angiosperms often involve the exclusion of paternal plastids prior to fertilisation (Hagemann and Schroder Citation1989). The diploid microspore mother cell in a young angiosperm anther undergoes meiosis to produce four haploid microspores, initiating pollen development. Each microspore then divides mitotically (=pollen mitosis I) to produce two unequal products: a smaller generative cell and a larger vegetative cell, the latter eventually producing the pollen tube. Species belonging to the Lycopersicon type, like Solanum lycopersicum (tomato), exclude plastids during pollen mitosis I, producing the vegetative cell, which receives all plastids, and the generative cell, which normally receives no plastids. The generative cell divides again (pollen mitosis II) to produce the sperm cells, so the male parent does not normally transfer plastids to the egg cell. The elimination of plastids at a later stage of gametogenesis occurs in Solanum tuberosum (potato) and is named accordingly (Solanum type). Species of the Solanum type contain few plastids in their generative cell, which are degraded prior to pollen mitosis II and pollen tube outgrowth (Hagemann and Schroder Citation1989). In both types, plastid exclusion is under strict paternal gamete control, and the mechanisms are sometimes referred to as “killing one’s own cytoplasm” (Greiner et al. Citation2015). A third model (Triticum type) was based on classical studies in Triticum aestivum (wheat) and Hordeum vulgare (barley) which suggested that the cytoplasm of sperm cells, containing some mitochondria and plastids, remains just outside the egg and central cells during fertilisation, the so-called cytoplasmic stripping (Mogensen Citation1996). Later studies showed that plastids in barley are maternally inherited according to the Lycopersicon type (Sodmergen et al. Citation2002), and in wheat they are inherited more like the Solanum type (Primavesi et al. Citation2017).
The terms maternal inheritance and paternal inheritance apply to species where the two types of gametes are morphologically distinct, such as sperm and egg. However, uniparental inheritance is not restricted to anisogamous organisms, but is also common in isogamous species, such as in several genera of green algae, where the two types of gametes are morphologically similar. Sexual reproduction in the model algae Chlamydomonas reinhardtii (Chlamydomonas) involves the fusion of haploid cells of mating type (mt) plus, which initiates fusion, and mating type (mt) minus (Nishimura Citation2010). The plastome is inherited only from the mt plus cell in over 95% of zygotes (Nishiyama et al. Citation2004), which is achieved by a series of events that actively eliminate mt minus derived ptDNA within 1–2 hours of mating (Nishimura et al. Citation2012). Others, such as the anisogamous Volvox cateri, progressively reduce the amount of ptDNA at each cell division during male gametogenesis (Miyamura Citation2010). Overall, these mechanisms are widespread in green algae: digestion of ptDNA during male gametogenesis, during or after fertilisation; followed by disintegration or fusion of the “empty” chloroplast with the intact one in the zygote (Miyamura Citation2010).
Why does maternal inheritance predominate?
A simple explanation that gamete size determines plastid inheritance in a quantitative way, e.g. female gametes are much larger and contain more plastids, is not sufficient. Since uniparental inheritance first evolved in isogamous organisms and was then enforced by anisogamous organisms to regulate uniparental inheritance via a single gamete, it is thought that uniparental inheritance must have arisen once the cyanobacterial endosymbiont evolved into a fully integrated component of the host cell (Zhang and Sodmergen Citation2010). The transfer of genetic material from the cyanobacterium to the host genome established the endosymbiotic relationship to ensure neither loss nor overproliferation of the engulfed bacterium. However, the development of meiosis would threaten such a system if the fusion of two gametes produced diploid zygotes containing twice the amount of plastid genomes. Without a way to return to a single plastome content, the next round would produce the quadruple amount, and so on (Goodenough and Heitman Citation2014). This dilemma suggests a hypothesis for the origin of uniparental inheritance. It is thought that the organelles in the mt plus and mt minus gametes are differentially labelled to allow the elimination of one type of plastome in the zygote after fertilisation, thereby regulating the plastome content.
It is therefore conceivable that a system controlling plastome content played a role in establishing uniparental inheritance, but in most modern egg/sperm systems, an egg cell can contain hundreds of plastid genomes, whereas a sperm cell has only a limited content of cytoplasma. Even without active exclusion of plastids from sperm cells, only few plastid genomes would be transferred from the cytoplasma of a male gamete. Other advantages must have come into play to explain why maternal inheritance has prevailed in evolution, and several models are discussed, which are not necessarily mutually exclusive. One common model is called the genomic conflict model. In this model, the two plastid genomes (maternal and paternal) enter into direct competition and evolutionary forces are supposed to prevent conflict and damage from forming a cytoplasmic mixture. The extent to which heteroplasmy is eliminated by genetic bottlenecks was analysed in an Arabidopsis mutant defective in organellar DNA repair. In this mutant, numerous (mitochondrial) and plastid heteroplasmies occur, which can be transmitted through reproductive tissues (Wu et al. Citation2020). The dynamics of heteroplasmic sorting was very fast in plastids and sorting out to homoplasmy was achieved within one to a few generations and showed tight effective bottlenecks (Broz et al. Citation2022). Naturally occurring heteroplasmy due to biparental inheritance, such as in Medicago truncatula, has also been reported to be completely sorted out within the first generation and only one of the two parental plastid genotypes is found in mature plants (Matsushima et al. Citation2008). This shows that even if the initial inheritance was biparental, there is a strong tendency towards a single plastid type in the mature plant. Another model, the co-adaptation model, based on close co-evolution and co-adaptation of plastid and nuclear genomes and the emergence of plastome-genome incompatibility, has been extensively studied in the genus Oenothera, manifesting as leaf pigmentation defects or even lethality (Greiner and Bock Citation2013). However, most models fail to explain why the uniparental paternal mode of plastid inheritance is rare and the maternal mode is preferred. A hypothesis that takes physiology into account (Greiner et al. Citation2015) suggests that in many species the mutation load of paternal ptDNA is much higher than that of maternal ptDNA in egg cells. In this scenario, oxidative stress resulting from high energy demands during male gametogenesis generates the mutational load factor, which may be combined with additional factors such as the availability of fewer plastid genomes in the male parent. Under these circumstances, paternal control of plastid transmission is consistent and results in the exclusion of plastids from sperm cells. Interestingly, when plastids are inherited paternally their plastomes are more compact and smaller than maternally and biparently inherited plastid genomes (Crosby and Smith Citation2012). If paternally inherited ptDNAs have a higher mutation rate due to male-biased mutation pressure, this may mean that there is a greater “burden” associated with carrying excess DNA in plastid genomes (Crosby and Smith Citation2012).
As maternal inheritance of plastid genomes is apparently dominant, it may have contributed to evolutionary dynamics and processes. Strict uniparental inheritance does not allow for the generation of novel allelic combinations through recombination and was theoretically expected to lead to an accumulation of deleterious mutations. Therefore, plastomes should suffer from impaired adaptive evolution due to the lack of recombination. However, empirical studies and computer modelling suggest the opposite, i.e. that cytoplasmic genomes can undergo effective adaptive evolution (Christie and Beekman Citation2017). Uniparental inheritance of cytoplasmic genomes reduces competition between different beneficial substitutions (clonal interference), favours the accumulation of these substitutions and also facilitates selection against deleterious substitutions (Christie and Beekman Citation2017). Positive selection or adaptive evolution has been shown to have operated on several chloroplast genes during evolution in the Poaceae family (grass family) (Hasegawa et al. Citation2009; Zhong et al. Citation2009) or in the genus Panax L. (ginseng) (Jiang et al. Citation2018). In shade-tolerant versus sun-loving Oryza (rice) species, 14 positively selected plastid genes relevant for photosynthesis were identified, suggesting that evolution of plastid genomes can promote adaptation to different ecological habitats (Gao et al. Citation2019).
Factors affecting plastid transmission
Despite a wealth of interesting descriptive knowledge, the molecular bases for uniparental inheritance in various taxa remain poorly understood. In Chlamydomonas, a breakthrough has been made in understanding how uniparental organelle inheritance has evolved through a selfish mechanism that favours one organelle over the other. The molecular key player in the molecular events leading to the selective degradation of ptDNA derived from mt minus gametes in Chlamydomonas zygotes has recently been identified (Joo et al. Citation2022). Otubain protein 2 (OTU2p), an otubain-like deubiquitinase encoded in the MT plus locus, acts as a protector of mt plus chloroplasts (). OTU2p prevents proteasome-mediated degradation of the preprotein Translocase of the Chloroplast outer membrane (TOC) during gametogenesis. In an elegant series of experiments, the authors showed that using OTU2p knockouts and proteasome inhibitor treatments, selective ptDNA degradation was enforced in chloroplasts with reduced TOC levels, regardless of mating type (Joo et al. Citation2022). They concluded that mt minus ptDNA is degraded because the corresponding mt minus derived chloroplast is delayed in importing repair components. This process ensures a mating type-linked organelle quality control mechanism and drives uniparental chloroplast inheritance. In addition, if mt plus ptDNA copies and/or integrity are compromised and functional ptDNA levels fall below a threshold, degradation of mt minus ptDNA can be withheld to ensure zygote maturation under harsh conditions.
Figure 1. Plastids are eliminated by different mechanisms. (A). The Octubain Protein2 (Otu2p) encoded in the mating type locus MT plus of Chlamydomonas reinhardtii acts as protector of the mt plus chloroplast by preventing proteasome-mediated degradation of the envelope preprotein translocase (EP) during gametogenesis allowing successful import of repair components after fertilisation. Otu2p is lacking a chloroplast transit peptide, therefore it probably does not enter the chloroplast stroma. (B). In the genus Oenothera subsection Oenothera five plastome types can be grouped into inheritance strength: type I and III strong, type IV and V weak, type II intermediate (not shown). Polymorphisms in the regulatory region of acetyl-CoA carboxylase (accD) influence lipid synthesis and profile of the plastid envelope membrane. After biparental inheritance plastid competitiveness results in elimination of the weak type (VI or V). (C). during male gametogenesis the microspore divides unequally during pollen mitosis I (PM I) into the vegetative cell (VC) and generative cell (GC). The nuclear encoded exonuclease defective in pollen organelle DNA degradation 1 (DPD1) is active in GC and possibly in VC (indicated by a question mark). Plastids disintegrate as DNA degrades. In Nicotiana tabacum mild chilling stress (10°C) and mutation in DPD1 increased entry of intact plastids into the GC and paternal transmission. N = haploid nuclear genome.
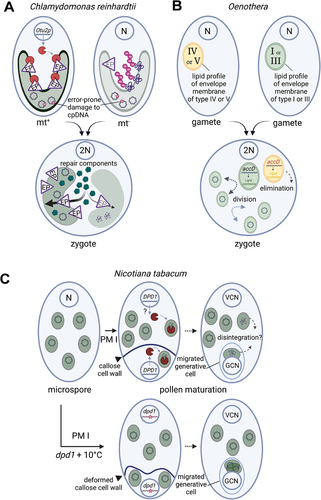
Because of the complexity of plastid inheritance and transmission in land plants, these traits are certainly under the control of several nuclear and plastid genes. The influence of nuclear genes on plastid inheritance can be studied in hybrid plants, which show biparental transmission. Using different Pelargonium cultivars, it has been shown that the hybrid of Pelargonium zonale and Pelargonium inquinans exhibits patterns of yellowish/whitish and green sectors due to impaired chloroplast development and chlorophyll deficiency of Pelargonium inquinans chloroplasts under the control of the hybrid nuclear genome in the yellowish/whitish sectors (Weihe et al. Citation2009). Nonetheless, segregation of variegation independent of plastid type occurs in Pelargonium, and already 50 years ago a two-interacting gene model was postulated to regulate plastid inheritance in this genus (Tilney-Bassett et al. Citation1992). A recent study using multiple interspecific crosses between 12 closely related crop wild relatives and the ornamental Pelargonium hortorum extended the model to rather three epistatically interacting genes (Breman et al. Citation2020). The variegated and chlorotic F2 plants did not show Mendelian inheritance under a one- or two-allele model and could also involve lethal interaction between two or more alleles (Breman et al. Citation2020). With today’s advances in sequencing and other high-throughput technologies and data analysis tools, it will certainly be possible in the future to approach the molecular basis of the respective alleles.
Biparental plastid inheritance is thought to promote competition, with only one parental plastid genotype being passed on to subsequent generations. Many studies on plastid competition and genetic incompatibility have been carried out in the genus Oenothera, subsection Oenothera, which comprises 13 species that can be crossed with each other (Greiner et al. Citation2011). Subsection Oenothera has a genetic make-up of entire haploid genomes: three haploid nuclear genomes (A; B; C) have been identified, which occur either in homozygous states (forming nuclear genotypes AA, BB, CC) or in different stable heterozygous states (forming nuclear genotypes AB, AC, BC), constituting the diploid organism. Homologous recombination and free segregation of chromosomes during meiosis are suppressed therefore an entire haploid genome is inherited as one linkage group (Rauwolf et al. Citation2008, Citation2011). The six nuclear genotypes can be combined with five distinguishable plastome types (I to V), resulting in 30 theoretical combinations. Of these, only 12 combinations are compatible and therefore produce green plants; the other combinations produce phenotypes ranging from greyish green to white plants (Greiner et al. Citation2008a, Citation2008b; Greiner and Bock Citation2013). It is worth noting that combinations of distinct nuclear genomes with plastome types represent an important factor in species definition, e.g. Oenothera grandiflora possesses combination BB-III and Oenothera elata possesses AA-I; across the 13 species seven combinations can be found. In Oenothera, biparental inheritance is the rule, but it was found that plastome types I or III can outcompete a second plastome in the F1 hybrid plant (Sobanski et al. Citation2019). Differences in the competitive behaviour of plastid genomes have been reported to be based mainly on polymorphisms in the regulatory region of the gene encoding the plastid-encoded subunit of acetyl-CoA carboxylase (accD), which catalyzes the first and rate-limiting step of lipid biosynthesis. It was hypothesised that differences in lipid biosynthesis influence the lipid profile of the plastid envelope membrane and may contribute to differential plastid division and turnover rates (Sobanski et al. Citation2019). Thus, competitiveness may result in uniparental plastid transmission to subsequent generations by eliminating the “weak” plastid type ().
There is increasing evidence that infrequent biparental inheritance of plastid genomes, also known as leakage (), is common in many plant species, and that occasional paternal leakage of ptDNA could provide a pathway for recombination that slows the accumulation of deleterious mutations. However, why and how this phenomenon occurs is not fully understood. Paternal plastid transmission could occur due to a failure in the selective elimination of ptDNA during pollen development. In principle, the presence or absence of plastids in the mature generative cell is determined not only by the elimination of plastids from the generative cell after pollen mitosis I as described above, but also by the behaviour of ptDNA in the young generative cell. It has been shown that in species with maternal inheritance of plastids, ptDNA in generative/sperm cells gradually decreases and eventually disappears (Nagata et al. Citation1999; Nagata Citation2010), suggesting that degradation of ptDNA in sperm organelles may be involved in the mechanisms of maternal inheritance. Already in 2011, a genetic screen identified an Arabidopsis mutant with increased levels of ptDNA (and mitochondrial DNA) in mature pollen grains (Matsushima et al. Citation2011). The mutation was identified in a gene encoding a conserved Mg2+-dependent exonuclease (DPD1) which is highly active in developing pollen and targeted to plastids (and mitochondria), indicating active ptDNA degradation in the male gametophyte. However, when the transmission of ptDNA from the male parent was tested, it was found that maternal plastid inheritance was unaffected in dpd1 Arabidopsis mutants, at least in a 102 scale (Matsushima et al. Citation2011). Instead, DPD1 was proposed to facilitate phosphate mobilisation from ptDNA under phosphorus starvation conditions (Takami et al. Citation2018). The function of DPD1 in maternal inheritance was recently re-examined in tobacco by generating a dpd1 knock-out mutant line using CRISPR/Cas9 editing (Chung et al. Citation2023). The dpd1 tobacco mutant did not show significant growth differences, but had reduced pollen viability and retained ptDNA in mature pollen grains. Large-scale inheritance assays showed an >80-fold increase in paternal plastid transmission compared to wild-type tobacco. It was also found that the presence of paternal plastids in the shoot apical meristem allows them to enter the germline and be passed on to progeny through maternal transmission (Chung et al. Citation2023). The same study also showed that plastid inheritance is influenced by an environmental factor. Maternal plastid inheritance is disrupted when male gametogenesis takes place at low temperatures and the frequency of paternal leakage is increased >150-fold compared to unstressed controls, corresponding to a biparental transmission frequency of 0.24%. Chilling conditions promote the incorporation of paternal plastids into the generative cell, accompanied by an irregular shape of the transiently formed callose cell wall (). Thus, chilling stress during gametogenesis may lead to incomplete exclusion of plastids during pollen mitosis I. In a current model, the exonuclease DPD1 serves to degrade the ptDNA of those plastids that managed to escape this exclusion process (). Frequencies between 2% and 3% of paternal plastid transmission were found when both factors were combined (Chung et al. Citation2023). Since the vegetative cell relies on a functioning plastid metabolism to support pollen tube germination (Niewiadomski et al. Citation2005), it is unclear to what extent ptDNA degradation is tolerable in this cell type.
Transgenic application
Transplastomic plants refer to transgenic plants in which the foreign genes are stably inserted into the plastid genome and are generally considered to be a natural biocontainment system. Compared to the 100% transmission of (homozygous) nuclear transgenes via pollen, the risk of gene flow via pollen in transplastomic plants is negligible in species with predominant maternal plastid inheritance. Another advantage of transplastomic plants is the high level of protein expression due to the presence of multiple copies of plastomes in each cell, making them an attractive platform for the production of pharmaceutical proteins or industrial enzymes (Li et al. Citation2021). Since the 1990s, there have been numerous reports of transplastomic lines conferring herbicide and insect resistance, improved tolerance to abiotic stresses and increased nutritional value (Wani et al. Citation2015). Today, the development of a sustainable bioeconomy is a high priority, and the conversion of non-food crops into efficient biofactories is expected to be a strong asset in this process. The non-food status and high-yield capacity make the plant genus Nicotiana one of the most suitable candidates, and transplastomic lines can be used as a platform for metabolic engineering and molecular farming (Molina-Hidalgo et al. Citation2021; Bock Citation2022). Crop plants that are amenable to transplastomic approaches and, importantly, produce homoplastomic progeny also belong to the Solanaceae family (tomato, potato) and the Brassicaceae family, such as Brassica oleracea (cabbage) (summarised in (Yarra Citation2020)) and Brassica napus (oilseed rape) (Schneider et al. Citation2015). Glycine max (soybean) (Dufourmantel et al. Citation2004, Citation2006), Lactula sativa (lettuce) (Ruhlman et al. Citation2007; Ruhlman Citation2021), Gossypium hirsutum (cotton) (Kumar et al. Citation2004), Beta vulgaris (sugar beet) (De Marchis et al. Citation2014; De Marchis and Bellucci Citation2021), and Populus alba (poplar) (Wu et al. Citation2021) have been reported to produce stable plastid transformants and possess the typical maternal plastid inheritance mode (). In cereals, such as rice, stable homoplasmy was not achieved (Lee et al. Citation2006).
Agronomically important traits in vegetable crops that confer herbicide or insect resistance can thus be expressed from the plastid genome, and vertical gene flow from transgenic plants to non-transgenic counterparts can be suppressed. In addition, the protein that confers herbicide resistance is transcribed and translated directly in the chloroplast. Through recombination of the bar gene into the ptDNA of tobacco (summarised in (Wani et al. Citation2015)) or oilseed rape (Schneider et al. Citation2015) an acetyltransferase is synthesised that detoxifies the herbicide phosphinothricin (also known as glufosinate), preventing its inhibitory effect on chloroplast-localised glutamine synthetase. Similarly, the expression of Bacillus thuringiensis Cry insecticidal proteins via transplastomic approaches is very effective in controlling arthropod pests, particularly lepidopteran caterpillars. Transplastomic tobacco lines accumulation high levels of Cry1A(c) showed strong resistance to several lepidopteran caterpillars (McBride et al. Citation1995). In addition, soybean (Dufourmantel et al. Citation2005) and poplar (Xu et al. Citation2020) lines expressing Cry genes in their plastid genomes showed strong insecticidal activity against velvetbean caterpillars and chrysomelid beetle larvae, respectively.
Another promising application of transplastomic crop plants is RNA interference (RNAi)-mediated pest control, which uses double-stranded RNA (dsRNA) to silence target genes in the insect of interest (Zhang et al. Citation2017). When dsRNA is expressed in plants and ingested by insects, it triggers RNAi, which silences the expression of essential insect genes, leading to impaired growth and pest mortality. Efficient uptake of long dsRNAs or hairpin RNAs (hpRNAs) is critical for RNAi in insects. If dsRNA would be expressed in the nucleus, the plant’s endogenous RNAi machinery can process it into small interfering RNAs (siRNAs), reducing its effectiveness when ingested by insects. Potato lines expressing a long double-stranded RNA (dsRNA) in the plastid genome, targeting the β–Actin gene of the Colorado potato beetle (Leptinotarsa decemlineata), accumulated significant amounts of dsRNA in leave chloroplasts. This led to a more robust response in the potato beetle compared to dsRNA expressed from the potato nuclear genome (Zhang et al. Citation2015). In tobacco, this method has been used to control non-chewing pests such as western flower thrips (Wu et al. Citation2022). Expression of dsRNA and hpRNA targeting 4 essential genes in the insect allowed the thrips to ingest the dsRNA, resulting in gene silencing and increased mortality. Transplastomic tobacco lines showed higher resistance and better protection against this insect than nuclear transgenic lines (Wu et al. Citation2022). Other successful examples (Bally et al. Citation2016; Dong et al. Citation2022; Wu et al. Citation2023) highlight this promising strategy for improving insect resistance.
Future perspectives
Despite many advantages and promising applications, transplastomic plants have not yet been commercialised. Furthermore, there are few reports of field studies using transplastomic plants. The few field studies have used transplastomic tobacco lines to investigate the accumulation of various proteins, such as a bacterial cellulase (Schmidt et al. Citation2019), a pathogenesis-related protein (Boccardo et al. Citation2019), a thermostable xylanase (Pantaleoni et al. Citation2014), thioredoxin f (Farran et al. Citation2014) or an interferon-alpha2b (Arlen et al. Citation2007). These transplastomic lines were evaluated for their agronomic performance to rule out possible negative effects of the overexpressed protein, which itself is intended for downstream processes such as cellulosic fuel production (Schmidt et al. Citation2019), but data on transgene containment were not collected. As potential gene flow from GM crops to conventional or organic crops has long been criticised, more emphasis needs to be placed on field studies to assess paternal leakage rates () of transplastomic plants, especially as environmental factors may influence the frequency (Chung et al. Citation2023). Only one study looked at paternal leakage from a transplastomic plant under field conditions. The transplastomic petunia line T16 (cultivar Pink Wave) (Horn et al. Citation2017) served as pollen donor and a white cultivar (Mitchell, W115) as recipient. Perhaps due to extensive drought, hybrid formation was very low, but a paternal transmission event was observed in about 160 cases (Horn et al. Citation2017).
Another challenge will be the identification of novel nuclear factors involved in plastid inheritance, more specifically the generation of nuclear mutations and the establishment of genetic screens to identify the “paternal leakage” phenotype (). The commonly used model plant tobacco may not be suitable in this respect because it is an allotetraploid plant, meaning that most genes have two functional copies, known as homeologs. although EMS mutagenesis is possible in tobacco (Udagawa et al. Citation2021), the phenotype of loss-of-function mutations in any single tobacco mutant is likely to be masked by gene redundancy and thus remain hidden in a genetic screen. In the model plant Arabidopsis, mutagenesis by ethyl methanesulfonate (EMS) is straightforward, homozygous loss-of-function mutations are routinely generated, and paternal plastids can be tagged with psbA-controlled herbicide resistance (Schneider et al. Citation2015) or even transplastomic plants can be generated (Ruf et al. Citation2019). However, a genetic screen (involving several hundred EMS mutant lines) could be very labour intensive, given the very low frequency of paternal leakage at whole plant level (1 in 50,000 cases, ). Even a 100-fold increase in a single mutant line would mean that at least 500 F1 progeny would have to be generated in a single test cross to identify the paternal leakage phenotype. A search for more suitable model plants is needed, and petunia may be a good candidate (). Petunia is amenable to genetic manipulation of the plastid genome (Zubkot et al. Citation2004), endogenous transposons allow mutagenesis of the nuclear genome (Gerats et al. Citation2013), and the frequency of paternal leakage provides a good starting point ().
Figure 2. Screening for nuclear mutations affecting plastid inheritance. (A). Strategy to identify mutant plants in which the elimination of plastids during the gametogenesis of the male parent is suppressed. Any wild-type line is used as the maternal parent. (B). Petunia hybrida as a model plant in this strategy. To generate a male parent with both requirements (traceable plastid marker and mutagenised nuclear gnome), the available transplastomic line T16 (Zubkot et al. Citation2004) is introgressed as female parent into line W138 containing multiple transposable dTph1 elements (Bombarely et al. Citation2016). The maternal parent in subsequent test crosses can be the standard white variety Mitchell (W115) and paternally transmitted plastids can be detected with a PCR marker specific for uidA (Horn et al. Citation2017).
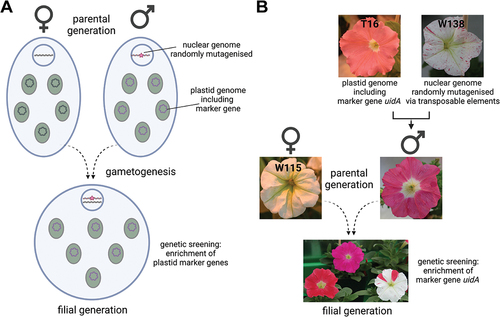
In contrast to maternal inheritance, where the exclusion of paternal plastids is controlled by the male gametophyte genome, it is not clear how biparental plastid inheritance is controlled. Biparental inheritance, detectable as heteroplasmy during early plant development, often leads to homoplasmy by sorting out one type of plastid at later stages during plant development. It remains to be determined whether the plastome-controlled elimination of one type of plastid, as observed in the genus Oenothera (), is also relevant in other genera with a biparental mode of inheritance (). The genus Passiflora, with its clade-specific modes of inheritance modes and sorting mechanisms in some subgenera, may be of interest in this respect (Shrestha et al. Citation2021).
Concluding remark
Plastid inheritance is an intricate process that is of immense importance for the survival and growth of both plants and algal cells. Understanding the regulation of plastid inheritance is a complex endeavour, involving a web of interactions between the plastid and the nucleus, which are further influenced by environmental factors. As researchers continue to explore this field, new discoveries are emerging that are expanding our knowledge and providing new perspectives on plastid inheritance. The ongoing exploration of plastid inheritance promises to open up new avenues for agricultural progress, and harnessing the full potential of this research is helping to develop innovative strategies not only in agriculture, but also in engineering and pharmaceutical applications.
Acknowledgments
Figures 1 and 2 were created with BioRender.com. The author would like to thank Dario Leister with the initial idea of this review article.
Disclosure statement
No potential conflict of interest was reported by the author(s).
Additional information
Funding
References
- Arlen PA, Falconer R, Cherukumilli S, Cole A, Cole AM, Oishi KK, Daniell H. 2007. Field production and functional evaluation of chloroplast-derived interferon-alpha2b. Plant Biotechnol J. 5(4):511–14. doi: 10.1111/j.1467-7652.2007.00258.x.
- Azhagiri AK, Maliga P. 2007. Exceptional paternal inheritance of plastids in Arabidopsis suggests that low-frequency leakage of plastids via pollen may be universal in plants. Plant J. 52(5):817–823. doi: 10.1111/j.1365-313X.2007.03278.x.
- Bally J, McIntyre GJ, Doran RL, Lee K, Perez A, Jung H, Naim F, Larrinua IM, Narva KE, Waterhouse PM. 2016. In-Plant protection against helicoverpa armigera by production of long hpRNA in chloroplasts. Front Plant Sci. 7:1453. doi: 10.3389/fpls.2016.01453.
- Boccardo NA, Segretin ME, Hernandez I, Mirkin FG, Chacon O, Lopez Y, Borras-Hidalgo O, Bravo-almonacid FF. 2019. Expression of pathogenesis-related proteins in transplastomic tobacco plants confers resistance to filamentous pathogens under field trials. Sci Rep. 9(1):2791. doi: 10.1038/s41598-019-39568-6.
- Bock R. 2022. Transplastomic approaches for metabolic engineering. Curr Opin Plant Biol. 66:102185. doi: 10.1016/j.pbi.2022.102185.
- Bombarely A, Moser M, Amrad A, Bao M, Bapaume L, Barry CS, Bliek M, Boersma MR, Borghi L, Bruggmann R, et al. 2016. Insight into the evolution of the Solanaceae from the parental genomes of petunia hybrida. Nat Plants. 2(6):16074. doi: 10.1038/nplants.2016.74.
- Breman FC, Snijder RC, Korver JW, Pelzer S, Sancho-Such M, Schranz ME, Bakker FT. 2020. Interspecific hybrids between Pelargonium x hortorum and species from P. Section ciconium reveal biparental plastid inheritance and multi-locus cyto-nuclear incompatibility. Front Plant Sci. 11:614871. doi: 10.3389/fpls.2020.614871.
- Broz AK, Keene A, Fernandes Gyorfy M, Hodous M, Johnston IG, Sloan DB. 2022. Sorting of mitochondrial and plastid heteroplasmy in Arabidopsis is extremely rapid and depends on MSH1 activity. Proc Natl Acad Sci USA. 119(34):e2206973119. doi: 10.1073/pnas.2206973119.
- Cato SA, Richardson TE. 1996. Inter- and intraspecific polymorphism at chloroplast SSR loci and the inheritance of plastids in Pinus radiata D. Don. Theor Appl Genet. 93(4):587–592. doi: 10.1007/BF00417952.
- Chen C, MacCready JS, Ducat DC, Osteryoung KW. 2018. The molecular machinery of chloroplast division. Plant Physiol. 176(1):138–151. doi: 10.1104/pp.17.01272.
- Chen J, Tauer G, Huang Y. 2002. Paternal chloroplast inheritance patterns in pine hybrids detected with trnL-trnF intergenic region polymorphism. Theor Appl Genet. 104(8):1307–1311. doi: 10.1007/s00122-002-0893-5.
- Chiu WL, Stubbe W, Sears BB. 1988. Plastid inheritance in Oenothera - organelle genome modifies the extent of biparental plastid transmission. Curr Genet. 13(2):181–189. doi: 10.1007/Bf00365653.
- Christie JR, Beekman M. 2017. Uniparental inheritance promotes adaptive evolution in cytoplasmic genomes. Mol Biol Evol. 34(3):677–691. doi: 10.1093/molbev/msw266.
- Chung KP, Gonzalez-Duran E, Ruf S, Endries P, Bock R. 2023. Control of plastid inheritance by environmental and genetic factors. Nat Plants. 9(1):68–80. doi: 10.1038/s41477-022-01323-7.
- Cipriani G, Testolin R, Morgante M. 1995. Paternal inheritance of plastids in interspecific hybrids of the genus Actinidia revealed by PCR-amplification of chloroplast DNA fragments. Mol Gen Genet. 247(6):693–697. doi: 10.1007/BF00290400.
- Crosby K, Smith DR. 2012. Does the mode of plastid inheritance influence plastid genome architecture? PloS One. 7(9):e46260. doi: 10.1371/journalpone.0046260.
- Dagan T, Roettger M, Stucken K, Landan G, Koch R, Major P, Gould SB, Goremykin VV, Rippka R, Tandeau de Marsac N, et al. 2013. Genomes of stigonematalean cyanobacteria (subsection V) and the evolution of oxygenic photosynthesis from prokaryotes to plastids. Genome Biol Evol. 5(1):31–44. doi: 10.1093/gbe/evs117.
- De Marchis F, Bellucci M. 2021. Plastid transformation in sugar beet: an important industrial crop. Methods Mol Biol. 2317:283–290. doi: 10.1007/978-1-0716-1472-3_16.
- De Marchis F, Pompa A, Bellucci M. 2014. Obtainment of fertile and genetically stable transplastomic sugar beet plants over three generations. Minerva Biotecnol. 26(4):247–255.
- Dong J, Wagner DB. 1994. Paternally inherited chloroplast polymorphism in Pinus: estimation of diversity and population subdivision, and tests of disequilibrium with a maternally inherited mitochondrial polymorphism. Genetics. 136(3):1187–1194. doi: 10.1093/genetics/136.3.1187.
- Dong Y, Wu M, Zhang Q, Fu J, Loiacono FV, Yang Y, Wang Z, Li S, Chang L, Bock R, et al. 2022. Control of a sap-sucking insect pest by plastid-mediated RNA interference. Mol Plant. 15(7):1176–1191. doi: 10.1016/j.molp.2022.05.008.
- Dudas B, Jenes B, Kiss GB, Maliga P. 2012. Spectinomycin resistance mutations in the rrn16 gene are new plastid markers in Medicago sativa. Theor Appl Genet. 125(7):1517–1523. doi: 10.1007/s00122-012-1930-7.
- Dufourmantel N, Pelissier B, Garcon F, Peltier G, Ferullo JM, Tissot G. 2004. Generation of fertile transplastomic soybean. Plant Mol Biol. 55(4):479–489. doi: 10.1007/s11103-004-0192-4.
- Dufourmantel N, Tissot G, Garcon F, Pelissier B, Dubald M. 2006. Stability of soybean recombinant plastome over six generations. Transgenic Res. 15(3):305–311. doi: 10.1007/s11248-005-5262-0.
- Dufourmantel N, Tissot G, Goutorbe F, Garcon F, Muhr C, Jansens S, Pelissier B, Peltier G, Dubald M. 2005. Generation and analysis of soybean plastid transformants expressing Bacillus thuringiensis Cry1Ab protoxin. Plant Mol Biol. 58(5):659–668. doi: 10.1007/s11103-005-7405-3.
- Ellis JR, Bentley KE, McCauley DE. 2008. Detection of rare paternal chloroplast inheritance in controlled crosses of the endangered sunflower Helianthus verticillatus. Heredity (Edinb). 100(6):574–580. doi: 10.1038/hdy.2008.11.
- El-Lithy ME, Rodrigues GC, van Rensen JJ, Snel JF, Dassen HJ, Koornneef M, Jansen MA, Aarts MG, Vreugdenhil D. 2005. altered photosynthetic performance of a natural Arabidopsis accession is associated with atrazine resistance. J Exp Bot. 56(416):1625–1634. doi: 10.1093/jxb/eri157.
- Farran I, Fernandez-San Millan A, Ancin M, Larraya L, Veramendi J. 2014. Increased bioethanol production from commercial tobacco cultivars overexpressing thioredoxin f grown under field conditions. Mol Breeding. 34(2):457–469. doi: 10.1007/s11032-014-0047-x.
- Fristedt R. 2017. Chloroplast function revealed through analysis of GreenCut2 genes. J Exp Bot. 68(9):2111–2120. doi: 10.1093/jxb/erx082.
- Gao LZ, Liu YL, Zhang D, Li W, Gao J, Liu Y, Li K, Shi C, Zhao Y, Zhao YJ, et al. 2019. Evolution of Oryza chloroplast genomes promoted adaptation to diverse ecological habitats. Commun Biol. 2(1):278. doi: 10.1038/s42003-019-0531-2.
- Gastony GJ, Yatskievych G. 1992. Maternal inheritance of the chloroplast and mitochondrial genomes in Cheilanthoid Ferns. Am J Botany. 79(6):716–722. doi: 10.1002/j.1537-2197.1992.tb14613.x.
- Gerats T, Zethof J, Vandenbussche M. 2013. Identification and applications of the petunia class II Act1/dTph1 transposable element system. Methods Mol Biol. 1057:223–237. doi: 10.1007/978-1-62703-568-2_16.
- Goodenough U, Heitman J. 2014. Origins of eukaryotic sexual reproduction. Cold Spring Harb Perspect Biol. 6(3):a016154–a016154. doi: 10.1101/cshperspect.a016154.
- Green BR. 2011. Chloroplast genomes of photosynthetic eukaryotes. Plant J. 66(1):34–44. doi: 10.1111/j.1365-313X.2011.04541.x.
- Greiner S, Bock R. 2013. Tuning a menage a trois: co-evolution and co-adaptation of nuclear and organellar genomes in plants. Bioessays. 35(4):354–365. doi: 10.1002/bies.201200137.
- Greiner S, Golczyk H, Malinova I, Pellizzer T, Bock R, Borner T, Herrmann RG. 2020. Chloroplast nucleoids are highly dynamic in ploidy, number, and structure during angiosperm leaf development. Plant J. 102(4):730–746. doi: 10.1111/tpj.14658.
- Greiner S, Rauwolf U, Meurer J, Herrmann RG. 2011. The role of plastids in plant speciation. Mol Ecol. 20(4):671–691. doi: 10.1111/j.1365-294X.2010.04984.x.
- Greiner S, Sobanski J, Bock R. 2015. Why are most organelle genomes transmitted maternally? Bioessays. 37(1):80–94. doi: 10.1002/bies.201400110.
- Greiner S, Wang X, Herrmann RG, Rauwolf U, Mayer K, Haberer G, Meurer J. 2008a. The complete nucleotide sequences of the 5 genetically distinct plastid genomes of Oenothera, subsection Oenothera: II. A microevolutionary view using bioinformatics and formal genetic data. Mol Biol Evol. 25(9):2019–2030. doi: 10.1093/molbev/msn149.
- Greiner S, Wang X, Rauwolf U, Silber MV, Mayer K, Meurer J, Haberer G, Herrmann RG. 2008b. The complete nucleotide sequences of the five genetically distinct plastid genomes of Oenothera, subsection Oenothera: I. sequence evaluation and plastome evolution. Nucleic Acids Res. 36(7):2366–2378. doi: 10.1093/nar/gkn081.
- Guillon JM, Raquin C. 2000. Maternal inheritance of chloroplasts in the horsetail equisetum variegatum (Schleich.). Curr Genet. 37(1):53–56. doi: 10.1007/s002940050008.
- Hagemann R. 2010. The foundation of extranuclear inheritance: plastid and mitochondrial genetics. Mol Genet Genomics. 283(3):199–209. doi: 10.1007/s00438-010-0521-z.
- Hagemann R, Schroder MB. 1989. The cytological basis of the plastid inheritance in angiosperms. Protoplasma. 152(2–3):57–64. doi: 10.1007/Bf01323062.
- Hasegawa M, Zhong B, Zhong Y. 2009. Adaptive evolution of chloroplast genomes in ancestral grasses. Plant Signal Behav. 4(7):623–624. doi: 10.4161/psb.4.7.8914.
- Horn P, Nausch H, Baars S, Schmidtke J, Schmidt K, Schneider A, Leister D, Broer I. 2017. Paternal inheritance of plastid-encoded transgenes in Petunia hybrida in the greenhouse and under field conditions. Biotechnol Rep (Amst). 16:26–31. doi: 10.1016/j.btre.2017.11.001.
- Jiang P, Shi FX, Li MR, Liu B, Wen J, Xiao HX, Li LF. 2018. Positive selection driving cytoplasmic genome evolution of the medicinally important ginseng plant genus Panax. Front Plant Sci. 9:359. doi:10.3389/fpls.2018.00359.
- Joo S, Kariyawasam T, Kim M, Jin E, Goodenough U, Lee JH. 2022. Sex-linked deubiquitinase establishes uniparental transmission of chloroplast DNA. Nat Commun. 13(1):1133. doi: 10.1038/s41467-022-28807-6.
- Kohzuma K, Sato Y, Ito H, Okuzaki A, Watanabe M, Kobayashi H, Nakano M, Yamatani H, Masuda Y, Nagashima Y, et al. 2017. The non-Mendelian green cotyledon gene in soybean encodes a small subunit of photosystem II. Plant Physiol. 173(4):2138–2147. doi: 10.1104/pp.16.01589.
- Kumar S, Dhingra A, Daniell H. 2004. Stable transformation of the cotton plastid genome and maternal inheritance of transgenes. Plant Mol Biol. 56(2):203–216. doi: 10.1007/s11103-004-2907-y.
- Kuo LY, Tang TY, Li FW, Su HJ, Chiou WL, Huang YM, Wang CN. 2018. Organelle genome inheritance in Deparia ferns (Athyriaceae, Aspleniineae, Polypodiales). Front Plant Sci. 9:486. doi: 10.3389/fpls.2018.00486.
- Lee SM, Kang KS, Chung H, Yoo SH, Xu XM, Lee SB, Cheong JJ, Daniell H, Kim M. 2006. Plastid transformation in the monocotyledonous cereal crop, rice (Oryza sativa) and transmission of transgenes to their progeny. Mol Cells. 21(3):401–410.
- Li S, Chang L, Zhang J. 2021. Advancing organelle genome transformation and editing for crop improvement. Plant Commun. 2(2):100141. doi: 10.1016/j.xplc.2021.100141.
- Mandel JR, Ramsey AJ, Holley JM, Scott VA, Mody D, Abbot P, Chapman M. 2020. Disentangling complex inheritance patterns of Plant organellar genomes: an example from Carrot. J Hered. 111(6):531–538. doi: 10.1093/jhered/esaa037.
- Martin W, Schnarrenberger C. 1997. The evolution of the Calvin cycle from prokaryotic to eukaryotic chromosomes: a case study of functional redundancy in ancient pathways through endosymbiosis. Curr Genet. 32(1):1–18. doi: 10.1007/s002940050241.
- Matsushima R, Hu Y, Toyoda K, Sodmergen, Sakamoto W. 2008. The model plant Medicago truncatula exhibits biparental plastid inheritance. Plant Cell Physiol. 49(1):81–91. doi: 10.1093/pcp/pcm170.
- Matsushima R, Tang LY, Zhang L, Yamada H, Twell D, Sakamoto W. 2011. A conserved, Mg(2)+ dependent exonuclease degrades organelle DNA during Arabidopsis pollen development. Plant Cell. 23(4):1608–1624. doi: 10.1105/tpc.111.084012.
- Matzrafi M, Gadri Y, Frenkel E, Rubin B, Peleg Z. 2014. Evolution of herbicide resistance mechanisms in grass weeds. Plant Sci. 229:43–52. doi: 10.1016/j.plantsci.2014.08.013.
- McBride KE, Svab Z, Schaaf DJ, Hogan PS, Stalker DM, Maliga P. 1995. Amplification of a chimeric Bacillus gene in chloroplasts leads to an extraordinary level of an insecticidal protein in tobacco. Biotechnology (NY). 13(4):362–365. doi: 10.1038/nbt0495-362.
- McCauley DE, Sundby AK, Bailey MF, Welch ME. 2007. Inheritance of chloroplast DNA is not strictly maternal in Silene vulgaris (Caryophyllaceae): evidence from experimental crosses and natural populations. Am J Bot. 94(8):1333–1337. doi: 10.3732/ajb.94.8.1333.
- McMurray LS, Preston C, Vandenberg A, Munoz-Santa I, Mao DL, Bett KE, Michelmore S, Paull JG. 2021. Paternal leakage inheritance and a fitness cost are associated with the chloroplastic psbA gene controlled metribuzin tolerance in lentil (Lens culinaris). Euphytica. 217(6): doi: 10.1007/s10681-021-02841-9.
- Miyamura S. 2010. Cytoplasmic inheritance in green algae: patterns, mechanisms and relation to sex type. J Plant Res. 123(2):171–184. doi: 10.1007/s10265-010-0309-6.
- Mogensen HL. 1996. The hows and whys of cytoplasmic inheritance in seed plants. Am J Bot. 83(3):383–404. doi: 10.1002/j.1537-2197.1996.tb12718.x.
- Molina-Hidalgo FJ, Vazquez-Vilar M, D’Andrea L, Demurtas OC, Fraser P, Giuliano G, Bock R, Orzaez D, Goossens A. 2021. Engineering metabolism in Nicotiana species: a promising future. Trends Biotechnol. 39(9):901–913. doi: 10.1016/j.tibtech.2020.11.012.
- Nagata N. 2010. Mechanisms for independent cytoplasmic inheritance of mitochondria and plastids in angiosperms. J Plant Res. 123(2):193–199. doi: 10.1007/s10265-009-0293-x.
- Nagata N, Saito C, Sakai A, Kuroiwa H, Kuroiwa T. 1999. The selective increase or decrease of organellar DNA in generative cells just after pollen mitosis one controls cytoplasmic inheritance. Planta. 209(1):53–65. doi: 10.1007/s004250050606.
- Natcheva R, Cronberg N. 2007. Maternal transmission of cytoplasmic DNA in interspecific hybrids of peat mosses, Sphagnum (Bryophyta). J Evol Biol. 20(4):1613–1616. doi: 10.1111/j.1420-9101.2007.01341.x.
- Niewiadomski P, Knappe S, Geimer S, Fischer K, Schulz B, Unte US, Rosso MG, Ache P, Flugge UI, Schneider A. 2005. The Arabidopsis plastidic glucose 6-phosphate/phosphate translocator GPT1 is essential for pollen maturation and embryo sac development. Plant Cell. 17(3):760–775. doi: 10.1105/tpc.104.029124.
- Nishimura Y. 2010. Uniparental inheritance of cpDNA and the genetic control of sexual differentiation in Chlamydomonas reinhardtii. J Plant Res. 123(2):149–162. doi: 10.1007/s10265-009-0292-y.
- Nishimura Y, Shikanai T, Nakamura S, Kawai-Yamada M, Uchimiya H. 2012. Gsp1 triggers the sexual developmental program including inheritance of chloroplast DNA and mitochondrial DNA in Chlamydomonas reinhardtii. Plant Cell. 24(6):2401–2414. doi: 10.1105/tpc.112.097865.
- Nishiyama R, Wada Y, Mibu M, Yamaguchi Y, Shimogawara K, Sano H. 2004. Role of a nonselective de novo DNA methyltransferase in maternal inheritance of chloroplast genes in the green alga, Chlamydomonas reinhardtii. Genetics. 168(2):809–816. doi: 10.1534/genetics.104.030775.
- Nugent GD, ten Have M, van der Gulik A, Dix PJ, Uijtewaal BA, Mordhorst AP. 2005. Plastid transformants of tomato selected using mutations affecting ribosome structure. Plant Cell Rep. 24(6):341–349. doi: 10.1007/s00299-005-0930-3.
- Ohyama K, Fukuzawa H, Kohchi T, Shirai H, Sano T, Sano S, Umesono K, Shiki Y, Takeuchi M, Chang Z, et al. 1986. Chloroplast gene organization deduced from complete sequence of liverwort marchantia-polymorpha chloroplast DNA. Nature. 322(6079):572–574. doi: 10.1038/322572a0.
- Pantaleoni L, Longoni P, Ferroni L, Baldisserotto C, Leelavathi S, Reddy VS, Pancaldi S, Cella R. 2014. Chloroplast molecular farming: efficient production of a thermostable xylanase by Nicotiana tabacum plants and long-term conservation of the recombinant enzyme. Protoplasma. 251(3):639–648. doi: 10.1007/s00709-013-0564-1.
- Park HS, Jeon JH, Cho W, Lee Y, Park JY, Kim J, Park YS, Koo HJ, Kang JH, Lee TJ, et al. 2023. High-throughput discovery of plastid genes causing albino phenotypes in ornamental chimeric plants. Hortic Res. 10(1):uhac246. doi: 10.1093/hr/uhac246.
- Park HS, Lee WK, Lee SC, Lee HO, Joh HJ, Park JY, Kim S, Song K, Yang TJ. 2021. Inheritance of chloroplast and mitochondrial genomes in cucumber revealed by four reciprocal F(1) hybrid combinations. Sci Rep. 11(1):2506. doi: 10.1038/s41598-021-81988-w.
- Powles SB, Yu Q. 2010. Evolution in action: plants resistant to herbicides. Annu Rev Plant Biol. 61(1):317–347. doi: 10.1146/annurev-arplant-042809-112119.
- Primavesi LF, Wu H, Mudd EA, Day A, Jones HD. 2017. Visualisation of plastid degradation in sperm cells of wheat pollen. Protoplasma. 254(1):229–237. doi: 10.1007/s00709-015-0935-x.
- Rajora OP, Dancik BP. 1992. Genetic-characterization and relationships of Populus-alba, P-Tremula, and X P-Canescens, and their clones. Theor Appl Genet. 84(3–4):291–298. doi: 10.1007/Bf00229485.
- Rauwolf U, Golczyk H, Meurer J, Herrmann RG, Greiner S. 2008. Molecular marker systems for Oenothera genetics. Genetics. 180(3):1289–1306. doi: 10.1534/genetics.108.091249.
- Rauwolf U, Greiner S, Mracek J, Rauwolf M, Golczyk H, Mohler V, Herrmann RG, Meurer J. 2011. Uncoupling of sexual reproduction from homologous recombination in homozygous Oenothera species. Heredity (Edinb). 107(1):87–94. doi: 10.1038/hdy.2010.171.
- Rios RD, Saione H, Robredo C, Acevedo A, Colombo N, Prina AR. 2003. Isolation and molecular characterization of atrazine tolerant barley mutants. Theor Appl Genet. 106(4):696–702. doi: 10.1007/s00122-002-1119-6.
- Ruf S, Forner J, Hasse C, Kroop X, Seeger S, Schollbach L, Schadach A, Bock R. 2019. High-efficiency generation of fertile transplastomic Arabidopsis plants. Nature Plants. 5(3):282–289. doi: 10.1038/s41477-019-0359-2.
- Ruf S, Karcher D, Bock R. 2007. Determining the transgene containment level provided by chloroplast transformation. Proc Natl Acad Sci USA. 104(17):6998–7002. doi: 10.1073/pnas.0700008104.
- Ruhlman TA. 2021. Biolistic plastid transformation in lettuce (Lactuca sativa) for oral delivery of biopharmaceuticals. Methods Mol Biol. 2317:267–281. doi: 10.1007/978-1-0716-1472-3_15.
- Ruhlman T, Ahangari R, Devine A, Samsam M, Daniell H. 2007. Expression of cholera toxin B-proinsulin fusion protein in lettuce and tobacco chloroplasts–oral administration protects against development of insulitis in non-obese diabetic mice. Plant Biotechnol J. 5(4):495–510. doi: 10.1111/j.1467-7652.2007.00259.x.
- Schmidt JA, McGrath JM, Hanson MR, Long SP, Ahner BA. 2019. Field-grown tobacco plants maintain robust growth while accumulating large quantities of a bacterial cellulase in chloroplasts. Nat Plants. 5(7):715–721. doi: 10.1038/s41477-019-0467-z.
- Schneider A, Stelljes C, Adams C, Kirchner S, Burkhard G, Jarzombski S, Broer I, Horn P, Elsayed A, Hagl P, et al. 2015. Low frequency paternal transmission of plastid genes in Brassicaceae. Transgenic Res. 24(2):267–277. doi: 10.1007/s11248-014-9842-8.
- Shinozaki K, Ohme M, Tanaka M, Wakasugi T, Hayashida N, Matsubayashi T, Zaita N, Chunwongse J, Obokata J, Yamaguchi-Shinozaki K, et al. 1986. The complete nucleotide sequence of the tobacco chloroplast genome: its gene organization and expression. EMBO J. 5(9):2043–2049. doi: 10.1002/j.1460-2075.1986.tb04464.x.
- Shrestha B, Gilbert LE, Ruhlman TA, Jansen RK. 2021. Clade-specific plastid inheritance patterns including frequent biparental inheritance in Passiflora interspecific crosses. Int J Mol Sci. 22(5):2278. doi: 10.3390/ijms22052278.
- Sierra J, Escobar-Tovar L, Leon P, Dietz K-J. 2023. Plastids: diving into their diversity, their functions, and their role in plant development. J Exp Bot. 74(8):2508–2526. doi: 10.1093/jxb/erad044.
- Sobanski J, Giavalisco P, Fischer A, Kreiner JM, Walther D, Schottler MA, Pellizzer T, Golczyk H, Obata T, Bock R, et al. 2019. Chloroplast competition is controlled by lipid biosynthesis in evening primroses. Proc Natl Acad Sci USA. 116(12):5665–5674. doi: 10.1073/pnas.1811661116.
- Sodmergen, Zhang Q, Zhang Y, Sakamoto W, Kuroiwa T. 2002. Reduction in amounts of mitochondrial DNA in the sperm cells as a mechanism for maternal inheritance in Hordeum vulgare. Planta. 216(2):235–244. doi: 10.1007/s00425-002-0853-y.
- Sutton BC, Flanagan DJ, Gawley JR, Newton CH, Lester DT, El-Kassaby YA. 1991. Inheritance of chloroplast and mitochondrial DNA in Picea and composition of hybrids from introgression zones. Theor Appl Genet. 82(2):242–248. doi: 10.1007/BF00226220.
- Svab Z, Maliga P. 2007. Exceptional transmission of plastids and mitochondria from the transplastomic pollen parent and its impact on transgene containment. Proc Natl Acad Sci USA. 104(17):7003–7008. doi: 10.1073/pnas.0700063104.
- Takami T, Ohnishi N, Kurita Y, Iwamura S, Ohnishi M, Kusaba M, Mimura T, Sakamoto W. 2018. Organelle DNA degradation contributes to the efficient use of phosphate in seed plants. Nat Plants. 4(12):1044–1055. doi: 10.1038/s41477-018-0291-x.
- Thyssen G, Svab Z, Maliga P. 2012. Exceptional inheritance of plastids via pollen in Nicotiana sylvestris with no detectable paternal mitochondrial DNA in the progeny. Plant J. 72(1):84–88. doi: 10.1111/j.1365-313X.2012.05057.x.
- Tilney-Bassett RA, almouslem AB, Amoatey HM. 1992. Complementary genes control biparental plastid inheritance in Pelargonium. Theor Appl Genet. 85(2–3):317–324. doi: 10.1007/BF00222876.
- Udagawa H, Ichida H, Takeuchi T, Abe T, Takakura Y. 2021. Highly efficient and comprehensive identification of ethyl methanesulfonate-induced mutations in Nicotiana tabacum L. by whole-genome and whole-exome sequencing. Front Plant Sci. 12:671598. doi: 10.3389/fpls.2021.671598.
- Wang T, Li Y, Shi Y, Reboud X, Darmency H, Gressel J. 2004. Low frequency transmission of a plastid-encoded trait in Setaria italica. Theor Appl Genet. 108(2):315–320. doi: 10.1007/s00122-003-1424-8.
- Wani SH, Sah SK, Sagi L, Solymosi K. 2015. Transplastomic plants for innovations in agriculture. A review. Agron Sustain Dev. 35(4):1391–1430. doi: 10.1007/s13593-015-0310-5.
- Weihe A, Apitz J, Pohlheim F, Salinas-Hartwig A, Borner T. 2009. Biparental inheritance of plastidial and mitochondrial DNA and hybrid variegation in Pelargonium. Mol Genet Genomics. 282(6):587–593. doi: 10.1007/s00438-009-0488-9.
- Wicke S, Schneeweiss GM, de Pamphilis CW, Muller KF, Quandt D. 2011. The evolution of the plastid chromosome in land plants: gene content, gene order, gene function. Plant Mol Biol. 76(3–5):273–297. doi: 10.1007/s11103-011-9762-4.
- Wu Y, Chang L, Jiang C, Xu L, Zhang J. 2021. Plastid transformation in poplar: a model for perennial trees. Methods Mol Biol. 2317:257–265. doi: 10.1007/978-1-0716-1472-3_14.
- Wu M, Dong Y, Zhang Q, Li S, Chang L, Loiacono FV, Ruf S, Zhang J, Bock R. 2022. Efficient control of western flower thrips by plastid-mediated RNA interference. Proc Natl Acad Sci USA. 119(15):e2120081119. doi: 10.1073/pnas.2120081119.
- Wu Z, Waneka G, Broz AK, King CR, Sloan DB. 2020. MSH1 is required for maintenance of the low mutation rates in plant mitochondrial and plastid genomes. Proc Natl Acad Sci USA. 117(28):16448–16455. doi: 10.1073/pnas.2001998117.
- Wu M, Zhang Q, Dong Y, Wang Z, Zhan W, Ke Z, Li S, He L, Ruf S, Bock R, et al. 2023. Transplastomic tomatoes expressing double-stranded RNA against a conserved gene are efficiently protected from multiple spider mites. New Phytol. 237(4):1363–1373. doi: 10.1111/nph.18595.
- Xu S, Zhang Y, Li S, Chang L, Wu Y, Zhang J. 2020. Plastid-expressed Bacillus thuringiensis (Bt) cry3Bb confers high mortality to a leaf eating beetle in poplar. Plant Cell Rep. 39(3):317–323. doi: 10.1007/s00299-019-02492-0.
- Yarra R. 2020. Plastome engineering in vegetable crops: current status and future prospects. Mol Biol Rep. 47(10):8061–8074. doi: 10.1007/s11033-020-05770-3.
- Zhang Y, Fang Z, Wang Q, Liu Y, Yang L, Zhuang M, Sun P. 2012. Chloroplast subspecies-specific SNP detection and its maternal inheritance in Brassica oleracea L. by using a dCAPS marker. J Hered. 103(4):606–611. doi: 10.1093/jhered/ess006.
- Zhang J, Khan SA, Hasse C, Ruf S, Heckel DG, Bock R. 2015. Pest control. Full crop protection from an insect pest by expression of long double-stranded RNAs in plastids. Sci. 347(6225):991–994. doi: 10.1126/science.1261680.
- Zhang J, Khan SA, Heckel DG, Bock R. 2017. Next-generation insect-resistant plants: RNAi-mediated crop protection. Trends Biotechnol. 35(9):871–882. doi: 10.1016/j.tibtech.2017.04.009.
- Zhang Q, Liu Y, Sodmergen. 2003. Examination of the cytoplasmic DNA in male reproductive cells to determine the potential for cytoplasmic inheritance in 295 angiosperm species. Plant Cell Physiol. 44(9):941–951. doi: 10.1093/pcp/pcg121.
- Zhang Q, Sodmergen. 2010. Why does biparental plastid inheritance revive in angiosperms? J Plant Res. 123(2):201–206. doi: 10.1007/s10265-009-0291-z.
- Zhong B, Yonezawa T, Zhong Y, Hasegawa M. 2009. Episodic evolution and adaptation of chloroplast genomes in ancestral grasses. PloS One. 4(4):e5297. doi: 10.1371/journalpone.0005297.
- Zhu S, Xu M, Wang H, Pan H, Wang G, Huang M. 2018. Study of spontaneous mutations in the transmission of poplar chloroplast genomes from mother to offspring. BMC Genom. 19(1):411. doi: 10.1186/s12864-018-4813-8.
- Zhu JR, Zhou H, Pan YB, Lu X. 2014. Genetic variability among the chloroplast genomes of sugarcane (Saccharum spp) and its wild progenitor species Saccharum spontaneum L. Genet Mol Res. 13(2):3037–3047. doi: 10.4238/2014.January.24.3.
- Zubkot MK, Zubkot EI, van Zuilen K, Meyer P, Day A. 2004. Stable transformation of petunia plastids. Transgenic Res. 13(6):523–530. doi: 10.1007/s11248-004-2374-x.