Abstract
Background. In acute renal failure (ARF) renal tubular cell death and detachment can be induced by necrotic and apoptotic mechanisms. Several studies have demonstrated some benefits of the use of growth factors in experimental models of ARF. Methods. MDCK cells were cultured in a glucose-free medium for 24 h and were submitted to hypoxia (pO2 around 35 mmHg) for additional 24 h. To evaluate the possible protective role of growth factors, EGF, IGF-I or HGF were added to the medium (20 ng mL). LDH release, viability (acridine orange and ethidium bromide dyes) and quantification of apoptotic cells (Hoechst 33342 dye fluorescence) were determined. Results. In the injury group, an increase on LDH release (60% vs. 3%) and on number of apoptotic cells (22% vs. 0.2%) which was associated with a reduced cell viability (61% vs. 94%) when compared with controls. Only HGF, not EGF or IGF-I, was able to protect cells from injury. HGF caused a significant reduction on LDH release (30%) and on number of apoptotic cells (5%), with an increase on viability cellular (79%). Conclusions. HGF decreases cell death on MDCK cells after hypoxic-induced injury, probably acting in both necrotic and apoptotic mechanisms.
Introduction
Acute renal failure (ARF) of ischemic etiology remains as the main cause of ARF in the adult population, presenting high rate of morbidity and mortality as shown by a recent multicentric and prospective study in which approximately 60% of patients with ischemic ARF died during the hospital admission.Citation[[1]]
ARF picture is a result of the sum of hormonal, hemodynamic, tubular, cellular and molecular events, which in the end, lead to the sudden reduction of glomerular filtration rate. In situations of great tubular injury, the rate of cellular exfoliation surpass that of formation of new cells, resulting in areas of gaps along the tubular basal membrane seen in histological sections, the nonreplacement phenomenon.Citation[[2]] The cellular detachment causes the formation of tubular casts, increase of intratubular pressure and the back leak of glomerular filtrate. Mechanisms of sub lethal injury and, depending on the extent of cell injury, apoptosis and necrosis are involved in this process.
Apoptosis is a process of cell death that occurs with gene activation and requires protein synthesis. In the beginning, the chromatin is condensed in the nucleus periphery, the nucleolus disintegrates and the nucleus retracts. The total volume of cells is reduced; the cytoplasm and the organelles are compacted causing the formation of apoptotic bodies that may be phagocytosed by macrophages or nearby cells.Citation[[3]]
The other mechanism of cellular death is necrosis. Necrosis is a passive process beginning with cell swelling, rupture of the cell membrane associated with the nuclear autolisys with random fragmentation of chromatin. Unlike the apoptosis, a strong inflammatory reaction occurs in the necrosis areas.Citation[[4]]
The cellular response to the ischemic injury is quite heterogeneous, while some cells are taken by apoptosis or necrosis, others survive, apparently intact or show signs of intense DNA synthesis and cell proliferation. The accurate knowledge of this pleomorphic response remains obscure and the identification of the paths and factors that may induce it or inhibit it shall determine the potential therapy site in ARF.
The growth factors, whether by autocrine, paracrine or endocrine action are capable to stimulate the cellular migration (motogenic activity), to regulate the passage of quiescent state (GO) to the active phase of DNA synthesis, to stimulate the cellular proliferation (mitogenic activity), in addition to promoting the tubulogenesis (morphogenic activity). Some of these growth factors have the capacity to inhibit or retard the apoptosis process. Based on these evidences, we decided to develop an ischemic model of ARF in vitro (gaseous hypoxia) and evaluate the possible effects of the growth factors EGF (epidermal growth factor), IGF-I (insulin-like growth factor-I) or HGF (hepatocyte growth factor) in this model.
Materials and Methods
Cells-MDCK cells (Madin-Darby Canine Kidney) obtained from ATCC (American Type of Culture Collection), cultivated in 5 mL DMEM culture medium (Dulbecco's Modified Eagle's Medium), supplemented with 5% bovine fetal serum (Cultilab, Campinas-São Paulo-Brazil), NaHCO3 2 g/L, HEPES 2.6 g/L, 10.000 UI/L penicillin, and 100 mg/L neomycin were all maintained into 25 cm2 polystyrene bottles in the incubator at 37°C, humidified in a gaseous air mixture (95%) and carbon dioxide (5%).
Hypoxia
Each culture bottle was tapped with a silicone cork and sealed with paraffin. We used two 30 × 7 mm needles to transfix the cork and through one of these needles, a system to infuse the gaseous mixture containing 95% nitrogen (N2) and 5% carbon dioxide (CO2) was connected. During 20 min, 3 to 4 L per minute of this gaseous mixture were infused with the purpose to reduce the partial pressure of oxygen from the culture media.
Establishing the Protocol of Cell Injury
Preliminarily, through the lactic dehydrogenase (LDH) release to the extracellular media, we analyzed the behavior of the cells before several situations of hypoxia and we noticed that the cells became susceptible to hypoxia injury only when they were deprived of glucose.
In the first experiment, cells cultivated in culture media with glucose were submitted to hypoxia and the bottles kept sealed for 24 h in the incubator.
In the second situation, cells were washed with PBS and we added glucose-free culture media. We performed the hypoxia and the bottles were immediately kept sealed for 24 h in the incubator.
In the final protocol of injury, cells were previously incubated with glucose-free culture media during 24 h. After elapsing this period, the cells were submitted to hypoxia and thereafter the bottles were kept sealed in the incubator for additional 24 h, thereby completing 48 h incubation in a glucose-free culture media, the last 24 h of which were associated with the hypoxia situation.
Cell Viability
Cell viability was analyzed through the method of exclusion by utilizing two fluorescent dyes. After trypsinization the cells were resuspended in 1 mL PBS. A solution of acridine orange (100 µg/mL) and ethidium bromide (100 µg/mL) was added (0.3 µL) to 10 µL of cell sample. The ethidium bromide and the acridine orange excite the orange and green fluorescence, respectively, when they are intermingled within the DNA. The ethidium bromide is eliminated when the plasma membrane is integral and therefore, only the acridine orange cross the membrane, leading to the green fluorescence, while, when both stains are absorbed, the fluorescence, because of ethidium bromide, is more intense, giving an orange brilliance to the cell.Citation[[5]] This suspension of stained cells was immediately seen in the fluorescence microscope (40×). Approximately 150 to 200 cells were counted, and considered viable when they were stained green and nonviable when they were stained orange. Results were expressed as percentage (%) of viable cells.
Apoptosis
The morphologic analysis of apoptosis was obtained by utilizing the die Hoechst 33342 fluorescent.Citation[[5]] The brief exposure of the cells to this dye at low concentrations leads to a strong marking of apoptotic cells, probably as a reflection of an increase of permeability of the plasma membrane of apoptotic cells to Hoechst 33342 dye, giving the intense blue stain. After trypsinization, the cells were resuspended in 1 mL of PBS. A solution of Hoechst 33342 (100 ug/mL) was added (10 µL) to 10 µL of cell sample and was visualized in the fluorescent microscope (40×). Approximately 150 to 200 cells were counted and considered apoptotic when they showed fragmentation of DNA and chromatin condensation, that is, a morphologic aspect of apoptotic bodies. The results are expressed in percentage (%) of apoptotic cells.
Permeability of Cellular Membrane
The release of LDH was utilized as lesion marker of the cellular membrane. In the experiment, the conversion of pyruvate into lactate, associated with the stoichiometric oxidation of NADH and NAD was followed spectrophotometrically against a white at 340 nm. Throughout the whole reading, the samples were maintained under agitation and at constant temperature of 25°C.Citation[[6]]
Growth Factors
Three growth factors were added isolate to the culture media in the beginning of the injury protocol, at the concentration of 20 ng/mL: EGF, IGF-I or HGF (recombinant-Human, Sigma Chemical Co., Saint Louis, USA). This dose has been previously reported to be efficient in inducing cell proliferation.Citation[[7]] Cell viability, apoptosis and LDH release observed in the presence of each growth factor were compared with the results obtained without the addition of growth factors.
Statistical Analysis
Data are expressed as average ± standard deviation. The Mann-Whitney t test and Kruskal-Wallis' variance analysis were used as appropriate to compare the groups. When the difference between groups was significant, the analysis was complemented with multiple comparison test (Bonferromi's). In all tests the level of significance was established in 0.05 or 5%.
Results
After the incubation period, there was significant reduction (N = 25, p<0.0001) of pO2 in the culture medium of cells submitted to hypoxia (35 ± 4 mmHg), when compared with the control group (135 ± 15 mmHg).
Establishing Injury Protocol Through LDH Release
In the presence of glucose there was no difference (N = 12, NS) between the control group (3.2 ± 1.2%) and that submitted to hypoxia during a period of 24 h (3.2 ± 1.4%) ().
Figure 1. Effects of hypoxia on LDH release in MDCK cells. The cells were cultivated in culture media with glucose and were submitted to hypoxia and the bottles kept sealed for 24 h in the incubator. Data are mean ± SD of culture bottle compared to control group (NS = not significant).
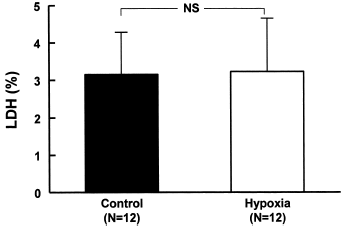
The lack of glucose during 24 h associated with hypoxia caused a significant increase (N = 12, p<0.05) in the release of LDH (10.9 ± 2.3%) when compared with the group without glucose (8.9 ± 2.4%) ().
Figure 2. LDH release of MDCK cells deprived of glucose for 24 h and submitted to hypoxia. The cells were washed with PBS and glucose-free culture media was added. Hypoxia was performed and the bottles were immediately sealed to wait 24 h in the incubator. Data are mean ± SD of culture bottle compared to cells deprived glucose only; p<0.05.
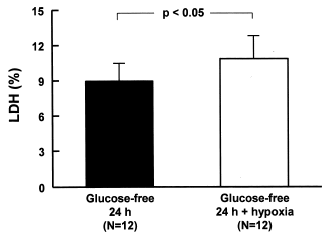
We also evaluated the effect of hypoxia on cells deprived from glucose during 48 h. The association with hypoxia in the last 24 h resulted in significant increase (N = 12, p<0.05) in the release of LDH (69.8 ± 12.1%) when compared to the group without glucose (54.2 ± 5.2%) (). The last group, because of the results indicating the highest distress was selected as the final protocol of injury and it shall be the object of comparison with other groups ().
Figure 3. LDH release of MDCK cells deprived of glucose for 48 h and submitted to hypoxia. The cells were incubated with glucose-free culture media during 24 h, then the cells were submitted to hypoxia and the bottles were kept sealed for additional 24 h, thereby completing 48 h incubation in a glucose-free culture media, the last 24 h of which were associates with the hypoxia situation. Data are mean ± SD of culture bottle compared to cells deprived glucose only; p<0.05.
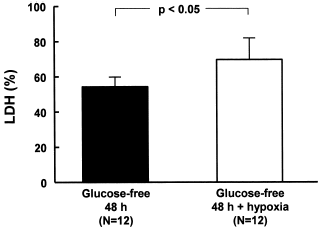
Evaluation of Growth Factors on LDH Release
The addition of EGF to the experiment did not change (p > 0.05) the release of LDH (59.4 ± 8.6%, N = 15) when compared with the injury group. Likewise, the use of IGF-1 did not change significantly (p>0.05) the release of LDH (51.0 ± 12.3%, N = 16) in the presence of the injury group.
The use of HGF reduced considerably (p<0.001, N = 16) the release of LDH (29.8 ± 8.2%, N = 16) when compared with the injury group (56.9 ± 11.8%, N = 42) ().
Evaluation of Growth Factors on Cell Viability
The injury protocol caused considerable reduction (p<0.001) on the cellular viability when compared with the control group (61.4 ± 4.0%, N = 16 vs. 93.5 ± 2.5%, N = 12, respectively). Neither the addition of EGF (64.3 ± 6.5%, N = 16) nor of IGF-I (68.5 ± 7.7%, N = 16) improved (p>0.05) the cellular viability in relation to the injury group. Only the HGF (79.39 ± 3.76%, N = 12) caused significant increase (p<0.001) of the cellular viability ().
Figure 6. Effects of growth factors EGF (20 ng/mL), IGF-I (20 ng/mL) or HGF (20 ng/mL) on viability (acridine orange plus ethidium bromide) in MDCK cells submitted to hypoxic-injury. Data are mean ± SD of culture bottle compared to controls and untreated injury group; *p<0.001 vs. Control; †p<0.01 vs. Injury; †p<0.05 vs. EGF.
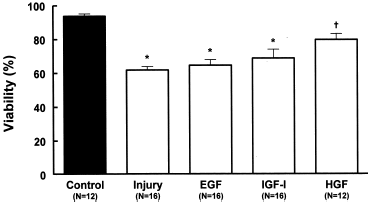
Evaluation of Growth Factors on Apoptosis Morphological Analysis
During the injury there was considerable increase (p<0.001) in the quantification of apoptotic cells in relation to the control group (22.2 ± 4.3%, N = 13 vs. 0.2 ± 0.3%, N = 12, respectively). Both EGF and IGF-I did not cause significant reduction (p>0.05) in the apoptosis morphological analysis (16.6 ± 6.8%, N = 12 and 13.3 ± 5.2%, N = 12, respectively).
The addition of HGF to the injury protocol caused significant reduction (p<0.001) in the occurrence of apoptotic cells (5.40 ± 1.97%, N = 12) ().
Figure 7. Effects of growth factors EGF (20 ng/mL), IGF-I (20 ng/mL) or HGF (20 ng/mL) on morphologic analysis of apoptosis (Hoechst 33342) in MDCK cells submitted to hypoxic injury. Data are mean ± SD of culture bottle compared to controls and untreated injury group; *p<0.001 vs. Control; †p<0.01 vs. Injury; †p<0.05 vs. EGF.
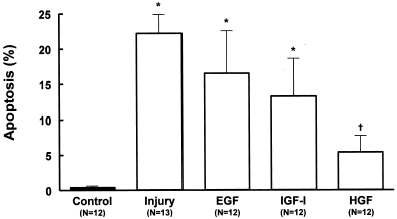
Discussion
When establishing the present injury protocol, it was first noted that the MDCK cells incubated in a culture media with glucose were resistant to injury probably because the pO2 was insufficiently low to completely block the oxidant phosphorylation mechanism.Citation[[8]]
Several cell lines, including primary cultures have high glycolytic capacity in the presence of glucose and hence they present reduced susceptibility to injury in situations of low content of oxygen or of chemical blockade of the oxidative phosphorylation.Citation[[9]]
In the present protocol, only after the cells were cultivated in a media without glucose, they became susceptible to hypoxia. An approximate increase of 22% to 29% in the LDH release occurred in the cells submitted to hypoxia when they were deprived of energetic substrate during 24 and 48 h, respectively. Therefore, susceptibility to hypoxia depends not only on its intensity and duration but also on the cellular type studied, the dependence on the ATP mitochondria production and on the integrity of its glycotylic pathway. In vivo studies, allied with these intrinsic metabolic differences, regional differences of perfusion and the workload are added in several segments of the nephron, making the determination of cellular susceptibility to ATP depletion more complex.
It was recently demonstrated that the apoptosis may also contribute to the development of ischemic or nephrotoxic ARF. Many factors are considered potential causes of apoptosis of renal tubular cells in acute renal failure, mainly those directly involved in the cellular injury, such as: ischemia, hypoxia and anoxia. Various experimental studies have demonstrated the presence of apoptosis and necrosis in the renal tissue exposed to different ischemic time length.Citation[[10]], Citation[[11]]
Liao et al.Citation[[12]] demonstrated apoptosis phenomenon during the reoxygenation period, after the cells were submitted to hypoxia situation. The absence of serum and the oxidative stress may also induce apoptosis into renal epithelial cells.Citation[[13]]
Through the chemical hypoxia, Wiegele et al.Citation[[14]] studied the necrosis and apoptosis mechanisms in culture of LLC-PK1 and MDCK cells. In the initial phases of ATP depletion, there was a rapid reduction of cellular proliferation associated with signs of apoptosis in a small proportion of cells, while the extended depletion of ATP led to cellular death by necrosis. The behavior of these cell lines was no different. Both necrosis and apoptosis were also demonstrated in MDCK cells submitted to atmospheric hypoxia and in biopsies from patients with acute renal failure of ischemic etiology.Citation[[15]]
All these findings corroborate the hypothesis that both mechanisms contributed to cellular death that follows ischemic renal injury and that the intensity and the duration of ATP depletion, associated with the individual susceptibility, determines the death mechanism of severely injured cells.
In the present study, the injury protocol caused the cellular death both by necrosis and apoptosis mechanisms, just like in several experimental studies in vivo and in vitro and in certain situations of clinical ARF.
The exogenous administration of such factors, especially EGF, IGF-I and HGF lessens the renal injury, accelerates the recovery of function and the renal structure and reduces the mortality in several experimental protocols.Citation[[16]], Citation[[17]], Citation[[18]]
In this study, the incubation of cells with EGF or IGF-I growth factors was not able to prevent the cellular injury as the data of LDH release to the extracellular element and the cellular viability indicate. Also, the presence of these growth factors only determined a tendency towards a reduction of apoptosis, especially the IGF-I.
The type, the intensity and the duration of injury, associated with the administration time of these growth factors and with the cellular phenotype, are factors that justify the differences in results and even the absence of benefits from the growth factors. Unfortunately, the beneficial effects were not clearly demonstrated in the clinical studies, when the IGF-I was employed, both prophylactically and during established ARF.Citation[[9]]
In the recent years it was established that HGF, through a paracrine action is, a strong factor involved in the renal regeneration after the ARF picture.Citation[[20]] The HGF expression also increases in organs distant from renal injury such as lung, spleen and liver and it seems to be mediated by protein production that was initially called injurine.Citation[[21]] The blood levels of HGF increases rapidly after the renal damage and the same occur with the urinary excretion of this growth factor in patients with ARF.Citation[[22]] Allied with paracrine action, the HGF may also act as an endocrine mediator during the recovery of renal tissue.
The mechanism by which the action of systemic HGF is directed specifically towards the injured organ is hardly known. Some evidences suggest that the circulating HGF is activated only within the injured organ.Citation[[23]] The increase in the number of its receptors in the injury area would be another alternative to justify this mechanism. The increase in the receptor expression was also induced in culture of renal tubular cells by HGF itself and by other growth factors such as EGF and IGF-I.Citation[[24]] Despite the increase in levels of circulating HGF, the administration of exogenous HGF has additional beneficial effect on the experimental acute renal failure. After the ARF establishment, the infusion of HGF increased the DNA synthesis, preserved the histological architecture of the injured renal tissue and anticipated the recovery of renal function. Following the same line, when administered prophylactically, HGF showed the same beneficial effects and was able to lessen the development of ARF in the treated animals.Citation[[20]]
Although convincing evidences have been demonstrated that the HGF accelerates the recovery and lessens the installation of ARF picture, the mechanisms of such benefits lack better explanations. The HGF seems to act stimulating the cellular proliferation, migration and differentiation, promoting in this way, the reconstitution of renal architecture through the tubulogenesis.Citation[[7]] More recent works have pointed out the effects of HGF on the apoptosis. This growth factor protected the renal tubular cells from toxicity by cisplatinum. The incubation of the cells with growth factor inhibited the apoptosis phenomenon, but could not protect them from necrosis.Citation[[25]] The HGF also inhibited the apoptosis in renal epithelial cells deprived of serum and also prevented the injury of endothelium cells induced by the alpha tumor necrosis factor and by dexamethasone.Citation[[26]]
In the present study, the use of HGF improved all the analyzed parameters. It increased the cellular viability and preserved the integrity of plasma membrane, suggesting a reduction in the mechanism of cellular death by necrosis, in addition to presenting anti-apoptotic action.
The HGF protective mechanisms evidenced in the present protocol were not determined and need further investigations. New studies must attempt to correlate these beneficial effects to stimulate cellular proliferation, the maintenance of ATP stocks and the homeostasis of intracellular calcium concentration and the preservation of the cytoskeleton structure. The mechanism by which it inhibits the apoptosis also remains uncertain. The HGF may be involved in regulating the expression of pro-apoptotic and anti-apoptotic factors and in the modulation of determined paths signs.Citation[[27]]
The data of these studies demonstrate the strong therapy effect of HGF to improve and prevent the acute renal failure. Irrespective of the increase of DNA synthesis and of cellular proliferation, clearly evidenced in the literatures, the reduction of cellular necrosis as well as the inhibition of the apoptosis phenomenon is possible relevant factors in the protection of renal tubular cells at the initial phase of renal injury.
References
- Liano F., Pascual J. Epidemiology of acute renal failure: a prospective, multicenter, community-based study. Madrid acute renal failure study group. Kidney Int. 1996; 50(3)811–818
- Solez K., Morel-Maroger L., Sraer J.D. The morphology of “acute tubular necrosis” in man: analysis of 57 renal biopsies and a comparison with the glycerol model. Medicine (Baltimore) 1979; 58(5)362–376
- Arends M.J., Wyllie A.H. Apoptosis: mechanisms and roles in pathology. Int. Rev. Exp. Pathol. 1991; 32: 223–254
- Lieberthal W., Koh J.S., Levine J.S. Necrosis and apoptosis in acute renal failure. Semin. Nephrol. 1998; 18(5)505–518
- Cotter T.M. Techniques in Apoptosis: A User's Guide. Portland Press Ltd., London 1996
- Beutler E. Red Cell Metabolism: A Manual of Biochemical Methods. Grune & Stratton, New York 1971
- Santos O.F.P., Barros E.J., Yang X.M., Matsumoto K., Nakamura T., Park M., Nigam S.K. Involvement of hepatocyte growth factor in kidney development. Dev. Biol. 1994; 163(2)525–529
- Brezis M.R., Epstein F.H. Acute renal failure due to ischemia (acute tubular necrosis). Acute Renal Failure, 3rd Ed., J.B. Lazarus, B.M. Brenner. Churchill Livingstone, New York 1993; 207–230
- Ardaillou R.R., Rondeau E. Biology of renal cells in culture. The Kidney, 5th Ed., B.M. Brenner. W.B. Saunders, Philadelphia 1996; 99–192
- Schumer M., Colombel M.C., Sawczuk I.S., Gobe G., Connor J., O'Toole K.M., Olsson C.A., Wise G.J., Buttyan R. Morphologic, biochemical, and molecular evidence of apoptosis during the reperfusion phase after brief periods of renal ischemia. Am. J. Pathol. 1992; 140(4)831–838
- Nogae S., Miyazaki M., Kobayashi N., Saito T., Abe K., Saito H., Nakane P.K., Nakanishi Y., Koji T. Induction of apoptosis in ischemia-reperfusion model of mouse kidney: possible involvement of Fas. J. Am. Soc. Nephrol. 1998; 9(4)620–631
- Liao H.J., Chen X.M., Cui S.W. Morphologic and molecular evidence of apoptosis during reperfusion phase after brief period of renal ischemia. Chung Hua I Hsueh Tsa Chih 1994; 74(10)629–631
- Lieberthal W., Triaca V., Koh J.S., Pagano P.J., Levine J.S. Role of superoxide in apoptosis induced by growth factor withdrawal. Am. J. Physiol. 1998; 275: F691–F702, (5 Pt 2)
- Wiegele G., Brandis M., Zimmerhackl L.B. Apoptosis and necrosis during ischaemia in renal tubular cells (LLC-PK1 and MDCK). Nephrol. Dial. Transplant. 1998; 13(5)1158–1167
- Bonventre J.V. Mechanisms of ischemic acute renal failure. Kidney Int. 1993; 43(5)1160–1178
- Hirschberg R., Ding H. Growth factors and acute renal failure. Semin. Nephrol. 1998; 18(2)191–207
- Humes H.D., Cieslinski D.A., Coimbra T.M., Messana J.M., Galvao C. Epidermal growth factor enhances renal tubule cell regeneration and repair and accelerates the recovery of renal function in postischemic acute renal failure. J. Clin. Invest. 1989; 84(6)1757–1761
- Miller S.B., Martin D.R., Kissane J., Hammerman M.R. Rat models for clinical use of insulin-like growth factor I in acute renal failure. Am. J. Physiol. 1994; 266: F949–F956, (6 Pt 2)
- Hirschberg R., Kopple J., Lipsett P., Benjamin E., Minei J., Albertson T., Munger M., Metzler M., Zaloga G., Murray M., Lowry S., Conger J., McKeown W., O'Shea M., Baughman R., Wood K., Haupt M., Kaiser R., Simms H., Warnock D. Multicenter clinical trial of recombinant human insulin-like growth factor I in patients with acute renal failure. Kidney Int. 1999; 55(6)2423–2432
- Igawa T., Matsumoto K., Kanda S., Saito Y., Nakamura T. Hepatocyte growth factor may function as a renotropic factor for regeneration in rats with acute renal injury. Am. J. Physiol. 1993; 265: F61–F69, (1 Pt 2)
- Matsumoto K., Tajima H., Hamanoue M., Kohno S., Kinoshita T., Nakamura T. Identification and characterization of “injurin,” an inducer of expression of the gene for hepatocyte growth factor. Proc. Natl. Acad. Sci. U.S.A. 1992; 89(9)3800–3804
- Taman M., Liu Y., Tolbert E., Dworkin L.D. Increase urinary hepatocyte growth factor excretion in human acute renal failure. Clin. Nephrol. 1997; 48(4)241–245
- Miyazawa K., Shimomura T., Naka D., Kitamura N. Proteolytic activation of hepatocyte growth factor in response to tissue injury. J. Biol. Chem. 1994; 269(12)8966–8970
- Liu Y., Tolbert E.M., Lin L., Thursby M.A., Sun A.M., Nakamura T., Dworkin L.D. Up-regulation of hepatocyte growth factor receptor: an amplification and targeting mechanism for hepatocyte growth factor action in acute renal failure. Kidney Int. 1999; 55(2)442–453
- Liu Y., Sun A.M., Dworkin L.D. Hepatocyte growth factor protects renal epithelial cells from apoptotic cell death. Biochem. Biophys. Res. Commun. 1998; 246(3)821–826
- Yo Y., Morishita R., Nakamura S., Tomita N., Yamamoto K., Moriguchi A., Matsumoto K., Nakamura T., Higaki J., Ogihara T. Potential role of hepatocyte growth factor in the maintenance of renal structure: anti-apoptotic action of HGF on epithelial cells. Kidney Int. 1998; 54(4)1128–1138
- Kosai K., Matsumoto K., Nagata S., Tsujimoto Y., Nakamura T. Abrogation of Fas-induced fulminant hepatic failure in mice by hepatocyte growth factor. Biochem. Biophys. Res. Commun. 1998; 244(3)683–690