Abstract
Aim
To evaluate the effects of small interference RNA protein kinase C-alpha (siRNA-PKCα) on experimental proliferative vitreoretinopathy (PVR) induced by dispase in mice.
Methods
C57BL/6 mice PVR models (4–6 weeks old) were induced by intravitreal injection of dispase and then equally divided into six groups. After 1 week, the five treatment groups received 2 μL, intravitreal injections of siRNA-PKCα at a concentration of 250 nM, 500 nM, 750 nM, 1000 nM, and 1500 nM, respectively, while the negative control group received 2 μL of 500 nM no-silencing siRNA. SiRNA-PKCα was transfected by a square wave electroporator. Postoperative ophthalmic observations of lens clarity and the fundus of the eyes were performed periodically. The eyeballs of the mice were enucleated and imbedded in optimal cutting temperature to perform histological and immunofluorescence analysis at the end of a 4-week observation period.
Results
Four weeks after the siRNA-PKCα injections, there are 100% lens dissolution and 100% PVR in the 250 nM group and 70%, 70%, 70%, and 50% PVR in the 500 nM, 750 nM, 1000 nM, and 1500 nM groups, respectively, which is significantly different from the negative group. Abnormalities in fundus appearance were related to the concentrations of siRNA-PKCα; a higher concentration of siRNA-PKCα resulted in a more normal fundus. Histological sections by hematoxylin-eosin staining of the eyes support the clinical observation. Immunofluorescence analysis showed that RPE65, glutamine synthase, glial acidic fibrillary protein, and α-smooth muscle actin were increasing in the retina with the decreasing concentration of siRNA-PKCα, indicating that intraocular siRNA-PKCα can partly inhibit changes of markers for glia cells, fibroblast cells, retinal pigment epithelium cells, and Müller cells in the process of PVR.
Conclusion
Gene therapy with siRNA-PKCα could effectively inhibit PVR in mice and provide us with a novel therapeutic target on PVR.
Introduction
Occurring in 5%–11% of patients, proliferative vitreoretinopathy (PVR) is the most common cause of recurrent retinal detachment after retinal detachment repair.Citation1,Citation2 Basic research has indicated that PVR is characterized by the formation of scar-like fibrous tissue containing myofibroblasts derived from transdifferentiated retinal pigment epithelial (RPE) cells, as well as other cell types, such as glial cells, which have entered the vitreous and induced contraction of cellular membranes within the vitreous cavity and on both detached retinal surfaces.Citation3 This non-neoplastic intraocular proliferation, along with other entities such as proliferative diabetic retinopathy or posttraumatic sequelae, represents some of the most important causes of blindness in developed countries.Citation4 The pathogenesis of this multifactorial condition in PVR is still not completely understood.Citation5
Protein kinase C (PKC) is a multigene family of phospholipid-dependent serine-threonine kinases that mediates the phosphorylation of numerous protein substrates in signal transduction and plays a central role in cellular processes such as proliferation, differentiation, mitosis, and inflammatory reaction.Citation6,Citation7 It has been well documented that the PKC family is involved in the processes of proliferation, migration, phagocytosis, and gel contraction in RPE cells,Citation8–Citation14 which are all reportedly implicated in the pathogenesis of PVR. We have also found that hypericin, a specific inhibitor of PKC, has potential as a therapeutic drug for PVR through its inhibition of the Ca2+ influx pathway.Citation15 Both Tahara et alCitation16 and our groupCitation17 found that intravitreal injection of hypericin was a safe and effective means of reducing PVR in rabbit eyes. To date, at least 12 isoforms of PKC have been cloned, all displaying different enzymatic properties, tissue expression, and intracellular localization.Citation18,Citation19 Consequently, specific inhibitors of PKC may inhibit all the PKC isoforms in which most of them did not involve in the PVR. Our previous studies characterized the expression pattern of all 12 PKC isoforms and showed that ten isoforms (PKCα, PKCβI, PKCβII, PKCδ, PKCɛ, PKCθ, ΡΚΟμ, PKCζ, PKCλ, and PKCτ) were present in cultured human RPE cells,Citation20 and this demonstrated that only PKCα affects cell cycle progression and proliferation in human RPE cells through the downregulation of cyclin-dependent kinase inhibitor, p27kip1.21 Therefore, we hypothesize that the PKCα inhibitor has potential as a therapeutic target for PVR and is a much more special target than that of the PKC inhibitor.
Small interference RNA (siRNA) is an effective strategy for inhibiting the expression of a gene. Considering that we can create greater stability of siRNA with chemical modification, in this study, we further investigated the effects of siRNA-PKCα (siRNA-PKCα) with methylation modification on the experimental PVR in mice and tried to find a novel therapeutic strategy to inhibit PVR.
Materials and methods
Mice
C57BL/6 mice, 4–6 weeks old, were purchased from the South Medical University Animal Center. Animal husbandry and experimental procedures were approved by the Animal Research Committee of Zhongshan Ophthalmic Center, Sun Yat-sen University. All animals were housed in a specific pathogen-free biohazard level 2 facility maintained by the Zhongshan Ophthalmic Center, Sun Yat-sen University (Guangzhou, People’s Republic of China) in accordance with Association of Assessment and Accreditation of Laboratory Animal Care guidelines.
In vivo model of PVR induced by dispase intravitreal injection
Sixty C57BL/6 mice PVR models were induced by dispase (Gibco®, Life Technologies, Carlsbad, CA, USA), as previously described by both Cantó Soler et al and Iribarne et alCitation22,Citation23 and our group.Citation24,Citation25 Mice were anesthetized with 4.3% chloral hydrate (0.01 mL/g) (The Affiliated Ophthalmic Hospital of Sun Yat-sen University, Guangzhou, People’s Republic of China). Their pupils were dilated with 0.5% tropicamide (Shenyang Sinqi Pharmaceutical Co, Ltd., Shenyang, People’s Republic of China). Intravitreal injections were made in the dorsonasal quadrant (1 o’clock) of the right eye. In addition, 3 μL of dispase at the concentration of 0.2 U/μL was injected into vitreous cavity with a Hamilton syringe, fitted with a 30 G needle. Control animals (n = 10) were injected with 3 μL of sterile saline.
SiRNA-PKCα intravitreal injection and transfection
One week after the dispase injections, the 60 mice were equally divided into six groups. The five treatment groups received 2 μL of intravitreal injection at concentrations of 250 nM, 500 nM, 750 nM, 1000 nM, and 1500 nM siRNA-PKCα with 2′-O-methylation modification (sense 5′-GAAUGAGAGCAA ACAGAAAdTdT-3′, antisense 5′-UUUCUGUUUG CUCUCAUUCdTdT-3′; Ruibo Biotech Co, Ltd, Guangzhou, People’s Republic of China), respectively. Assuming that the volume of the vitreous cavity is 10 μL, the final concentrations of vitreous cavity in the eye were 50 nM, 100 nM, 150 nM, 200 nM, and 300 nM. The negative control group received 2 μL of 500 nM no-silencing siRNA. After Hypromellose Eye Drops (The Affiliated Ophthalmic Hospital of Sun Yat-sen University, GuangZhou, People’s Republic of China) was dropped into the eyes, the corneas were touched by the electrode (CUY650P7) of the square wave electroporator (CUY21EDIT, ΝΕΡΑ GENE Co, Ltd, Chiba, Japan). Then, the siRNA-PKCα in the vitreous cavity was transfected according to the following parameters: resistance: 0.8–1.5 kohm; volt: 80–100 V; pon: 50 ms; poff: 950 ms; number: 5; and ampere: 0.080.15 A. The mice were then observed for 4 weeks. All experimental procedures adhered to the Association for Research in Vision and Ophthalmology Resolution on the Use of Animals in Ophthalmic and Vision Research.
Follow-up examinations
The injected eyes were examined and assessed with a surgical microscope or direct ophthalmoscopy, including the corneas, lens opacities, intravitreal hemorrhage, and the fundus for 4 weeks after intravitreal siRNA-PKCα injection. Because intravitreal hemorrhages and cataracts have often occurred in previous studies,Citation26 clinical PVR-like signs were defined as the presence of one of the following three symptoms: retinal folds, epiretinal membranes, and an uneven iris at 1 week, 2 weeks, and 4 weeks in our previous study and in this experiment.Citation24,Citation25 This evaluation system was modified from Cantó Soler et al and Iribarne et al.Citation22,Citation23
Retinal PKCα levels after siRNA-PKCα injection
In order to prove the efficacy of siRNA-PKCα, retinal PKCα levels at 2 weeks after a 1500 nM siRNA-PKCα injection were detected using reverse transcription-polymerase chain reaction (RT-PCR) and Western blot analysis, as we previously reported.Citation27
RT-PCR
Total ribonucleic acid (RNA) in treated eyes (n = 5) and control eyes (n = 3) was extracted using Trizol® reagent according to the manufacturer’s procedure (Life Technologies). The integrity of the RNA was checked by 2% agarose gel electrophoresis. Approximately 1 μg of RNA was reverse-transcribed following the protocol of the SuperScript® (Life Technologies) first-strand synthesis system. Complementary DNA encoding PKCα and glyceraldehyde-3-phosphate dehydrogenase (GAPDH) genes were amplified by PCR as follows: denaturation at 94°C for 30 seconds, annealing at 63°C for 30 seconds, and elongation at 72°C for 45 seconds. Primer sequence was designed using Primer3 (http://frodo.wi.mit.edu/cgibin/primer3/primer3www.cgi. For PKCα, the forward primer was 5′-GTTTACCCGGCCAACGACT-3′ and the reverse primer was 5′-TCTTTCACCTCATG-CACGTTC-3′. A housekeeping gene, GAPDH, was used as the internal control. The forward primer was 5′-TTGTCAT-GGGAGTGAACGAGA-3′, and the reverse primer was 5′-CAGGCAGTTGGTGGTACAGG-3′. PCR products were analyzed by agarose (2%) gel electrophoresis. Quantifications of signal intensity were confirmed using a specific computer program (Image J 1.43U software; Wayne Rasband, National Institutes of Health, Bethesda, MD, USA).
Western blot analysis
The retinal samples in treated eyes (n = 5) and control eyes (n = 3) were lysed with the sample buffer that contained 60 mM Tris, pH 6.8, 2% (w/v) sodium dodecyl sulfate, 100 mM 2-mercaptoethanol, and 0.01% (w/v) bromophenol blue. The lysate was then incubated on ice for 30 minutes. The lysate was scraped using a cell scraper and harvested using a pipettor, then centrifuged at 4°C for 30 minutes. The supernatant was collected and boiled for 5 minutes and stored at −20°C. Cellular extracts from human RPE cells were processed for Western blot analysis. Briefly, 30 μg of protein per well was loaded on a 10% sodium dodecyl sulfate-polyacrylamide gel electrophoresis gel. The protein was electrotransferred to polyvinylidene difluoride membranes (Merck Millipore, Billerica, MA, USA) for 2 hours at 250 mA, then blocked with Tris-buffered saline containing 5% of nonfat milk and 0.1% of Tween-20 (TBST) for 1 hour, and incubated with rabbit anti-PKCα (Cell Signaling Technology, Inc, Danvers, MA, USA) overnight. After three washes with TBST, the membranes were incubated with horseradish peroxidase conjugated secondary antibody for 1 hour at room temperature and washed with TBST. Expression of the proteins was detected by chemiluminescence using the ECL kit (Cell Signaling Technology, Inc) following the manufacturer’s instructions. GAPDH (Cell Signaling Technologies, Inc) was used as an internal control.
Tissue preparation and histological and immunofluorescence analysis
Mice for histochemical studies were killed at 4 weeks after siRNA-PKCα injection and dissected eyes were cryopreserved using optimal cutting temperature (OCT; Sakura Finetek USA, Inc, Torrance, CA, USA). For hematoxylineosin (HE) and immunofluorescent staining, consecutive 6 μm-thick sections of each sample were cut and thaw-mounted onto poly-L-lysine-coated glass slides. For confocal microscopy, double immunostaining was done using two primary antibodies incubated, respectively, for about 20 hours at room temperature and then secondary antibodies for about 1 hour, in the dark. Primary antibodies served as markers for RPE cells (RPE65), Müller cells (glutamine synthase [GS]), astroglial cells (glial acidic fibrillary protein [GFAP]), and fibroblast cells (α-SMA). Dilutions and the source of each primary antibody are described in . Negative controls were made by omitting the primary antibodies. There are three secondary antibodies in this study: R-phycoerythrin conjugated goat anti-rat immunoglobulin (Ig)G (1:10; Southern Biotechnology Associates, Inc, Birmingham, AL, USA), R-phycoerythrin-conjugated goat antimouse IgG (1:10; Southern Biotechnology Associates, Inc), and fluorescein isothiocyanate-labeled goat antirabbit IgG (1:10; KPL, Kirkegaard and Perry Laboratories, Inc, Gaithersburg, MD, USA). Sections were washed four times in phosphate-buffered saline (5 minutes each time) and mounted under coverslips in Antifade solution (Applygen Technologies Inc, Beijing, People’s Republic of China) for observation with a Zeiss laser scanning confocal microscope (LSM 510 META; Carl Zeiss Meditec AG, Jena, Germany).
Table 1 Characteristics of primary antibodies used in this study
Statistical analysis
Results are expressed as mean ± standard deviation. The Kruskal-Wallis test and one-way analysis of variance (ANOVA) were used to determine significant differences between the treated groups and the control groups. A value of Ρ < 0.05 was considered significant.
Results
PVR development after siRNA-PKCα injection
Intravitreal injection and electricity transfection by square wave electroporator were shown to be flexible (). After dispase injection, severe hemorrhage in 17% (10/60), mild hemorrhage in 67% (40/60), and no hemorrhage in 17% (10/60) were observed in the dispase-injected eyes in the first week. Four weeks after the siRNA-PKCα injection, 100% (10/10) lens dissolution and PVR were found in the 250 nM group; however, 70% (7/10), 70% (7/10), 70% (7/10), and 50% (5/10) PVR were found in the 500 nM, 750 nM, 1000 nM, and 1500 nM groups, respectively, which is significantly different from those in the 250 nM group and the negative group (100%) (). Abnormalities in fundus appearance were related to the concentrations of siRNA-PKCα; a higher concentration of siRNA-PKCα resulted in a more normal fundus. The PVR percentages among the five treatment groups and one negative group were statistically significantly different (Kruskal—Wallis test, x2 = 5.5543, Ρ = 0.0187, ).
Figure 1 siRNA-PKCα intravitreal injection and transfection in mice. (A) Intravitreal injection with a Hamilton syringe, fitted with a 30 G needle. (B) Electricity transfection by square wave electroporator.
Abbreviation: siRNA-PKCα, small interference RNA-protein kinase C-alpha.
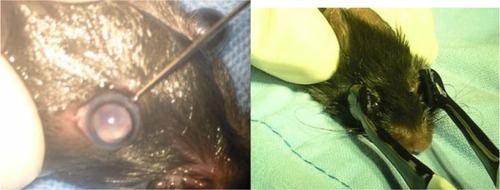
Figure 2 PVR development at 4 weeks after siRNA-PKCα injection. (A) Clinical PVR fundus photographs in the 250 nM and 1500 nM siRNA-PKCα, and in the negative control at the end of the 4-week observation period. Obvious retinal folds, epiretinal membranes, and uneven irises are observed in the 250 nM siRNA-PKCα treatment group, similar to those in the negative group; however, the radial distribution of the retinal arteries and veins are shown in the 1500 nM siRNA-PKCα. (B) Percentage in the five treatment groups and negative control.
Note: The percentages in the 250 nM and negative groups are significantly different from those in the other groups. *P < 0.05.
Abbreviations: PVR, proliferative vitreoretinopathy; siRNA-PKCα, small interference RNA-protein kinase C-alpha.
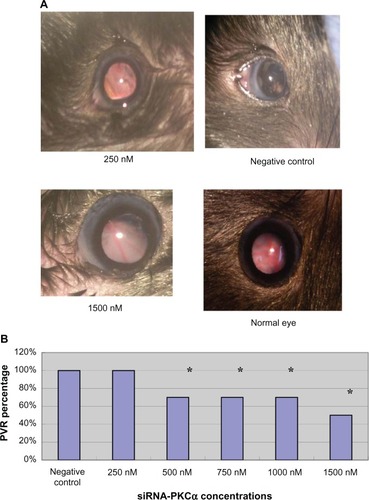
Retinal PKCα expressions after siRNA-PKCα injection
As shown in and , RT-PCR results showed that PKCα messenger RNA (mRNA) was significantly down-regulated among the siRNA-PKCα-injected group compared with those of dispase-injected and control groups (ANOVA, Ρ = 0.00018 < 0.01, Ρ = 0.00010 < 0.01). Consistent with a change at the mRNA level, the PKCα protein after siRNA-PKCα injection decreased compared with the dispase-injected and control groups (ANOVA, Ρ = 0.00220 < 0.01, Ρ = 0.00490 < 0.01). These data indicate that siRNA-PKCα can decrease retinal PKCα expression after siRNA-PKCα injection.
Figure 3 RT-PCR analysis after injection of 1500 nM siRNA-PKCα.
Notes: PKCα messenger RNA was significantly down regulated following siRNA-PKCα injection when compared with those that were dispase-injected or in the control groups (ANOVA, **P = 0.00018 < 0.01, *P = 0.00010 < 0.01). The GAPDH band is used for quantitation.
Abbreviations: RT-PCR, reverse transcription polymerase chain reaction; siRNA-PKCα, small interference RNA-protein kinase C-alpha; PKCα, protein kinase C-alpha; RNA, ribonucleic acid; ANOVA, analysis of variance; GAPDH, glyceraldehyde 3-phosphate dehydrogenase.
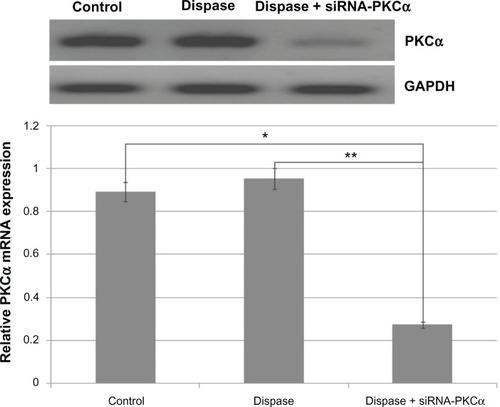
Figure 4 PKCα protein changes after siRNA-PKCα injection.
Notes: Western blot analysis shows that PKCα decreased compared to those from the dispase-injected and control groups (ANOVA, *P = 0.00220 < 0.01, **P = 0.00490 < 0.01). The β-actin band with 42 kDa is used for quantitation.
Abbreviations: PKCα, protein kinase C-alpha; siRNA-PKCα, small interference RNA-protein kinase C-alpha; ANOVA, analysis of variance.
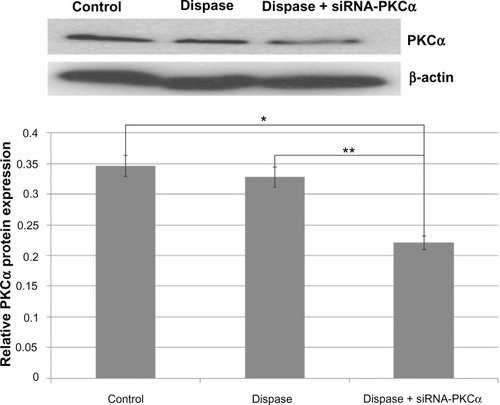
Pathologic changes after siRNA-PKCα injection
All mice at 4 weeks after siRNA-PKCα injection were further confirmed by histology. HE-stained frozen sections of eyes showed 100% lens dissolution and severe retinal detachment in the 250 nM and negative control groups; 70% (7/10), 70% (7/10), 70% (7/10), and 50% (5/10) retinal detachment in the 500 nM, 750 nM, 1000 nM, and 1500 nM groups, respectively (). HE results also showed that two eyes, two eyes, two eyes, and three eyes with siRNA-PKCα injection showed normal morphology in the 500 nM, 750 nM, 1000 nM, and 1500 nM groups, respectively. Therefore, histological sections of the eyes further support the clinical observation. Altogether, the data similar to the clinical examinations indicated that a high concentration of siRNA-PKCα resulted in a more normal eye structure and can partly inhibit the onset of PVR.
Figure 5 HE staining of eyes at 4 weeks after siRNA-PKCα injection.
Notes: A proliferative membrane and retinal detachment in the vitreous cavity were observed in the negative control, 250 nM, 500 nM, and 750 nM groups; however, normal retinal structures were found in the 1000 nM and 1500 nM groups when compared with normal eyes. Scale bar: 100 μm.
Abbreviations: HE, hematoxylin and eosin; siRNA-PKCα, small interference RNA-protein kinase C-alpha.
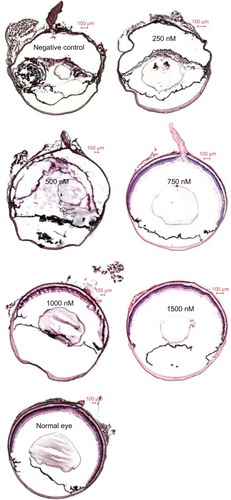
HE-stained frozen sections clearly showed marked proliferative membranes and retinal detachment between the RPE and the sensory retinas, as well as destructed retinas and lenses. In the process of PVR development, the RPE65, GS, GFAP, and α-SMA labeled cells were involved in the PVR eyes of mice. There are faint expressions of RPE65 and GS in normal retinas, indicating that RPE cells and Müller cells exist in the retinas of mice. These expressions seemed more pronounced in the negative control and the 250 nM, 500 nM, and 750 nM groups than in the 1000 nM and 1500 nM groups, as shown in . Similarly, there are weak expressions of GFAP and α-SMA in normal retinas, indicating that fibroblast cells and astroglial cells exist in the retinas of mice. These expressions seemed stronger in the negative control and in the 250 nM, 500 nM, and 750 nM groups when compared to the 1000 nM and 1500 nM groups, as shown in .
Figure 6 Immunofluorescence analysis of RPE65 and GS 4 weeks after siRNA-PKCα injection.
Notes: There are faint expressions of RPE65 (red) and GS (green) in normal retinas; these expressions seemed most pronounced in the epiretinal membranes in the negative control, as well as in the 250 nM, 500 nM, and 750 nM groups when compared to the 1000 and 1500 nM groups. Scale bar: 100 μm.
Abbreviations: RPE, retinal pigment epithelium; GS, glutamine synthetase.
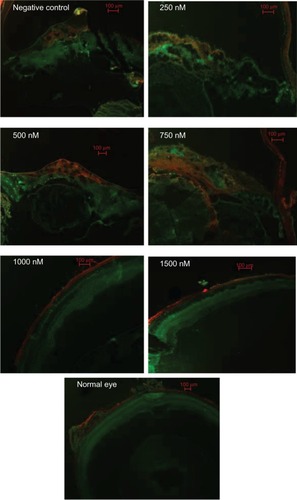
Figure 7 Immunofluorescence analysis of GFAP and α-SMA at 4 weeks after siRNA-PKCα injection.
Notes: There are faint expressions of GFAP (red) and (green) in normal retinas; these expressions seemed more pronounced in the epiretinal membranes in the negative control, as well as in the 250 nM, 500 nM, and 750 nM groups than in the 1000 nM and 1500 nM groups. Scale bar: 100 μm.
Abbreviations: GFAP, glial fibrillary acidic protein; α-SMA, α-smooth muscle antibody; siRNA-PKCα, small interference RNA-protein kinase C-alpha.
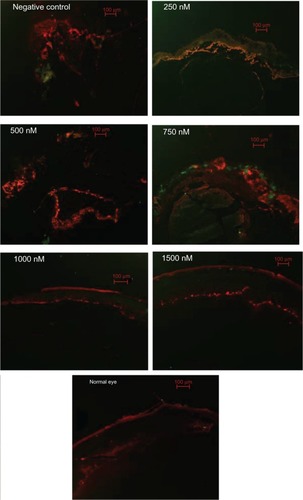
Immunofluorescence analysis showed that RPE65, GS, GFAP, and α-SMA were increasing in the retinas with the decreasing concentration of siRNA-PKCα, indicating that intraocular siRNA-PKCα can partly inhibit changes of markers for glia, fibroblast, RPE, and Müller cells in the process of PVR.
Discussion
In the present study, we generated a PVR model induced by dispase in mice and found that PVR can be partly inhibited by high concentrations of siRNA-PKCα injection.
Recently, there has been an increasing trend in inducing the PVR model in the eyes of mice and rabbits using dispase.Citation22–Citation26,Citation28,Citation34 Dispase, a neutral protease isolated from Bacillus polymyxa, is able to harvest and culture cells due to its ability to cleave the basal membrane in various tissues, and can be used as an intravitreal injection material to induce PVR. Our previous data showed that neutrophils in the anterior chamber and PVR-like signs in the retinas were found, and that specific immune reactions were not involved after intravitreal dispase injection in mice.Citation24,Citation25 Therefore, the dispase PVR model in mice or in rabbits is an ideal model for researching the pathogenesis of PVR.
PKC is an effective biologic target to inhibit the PVR animal model. Rabbit models have also shown that intravitreal injection of special PKC inhibitor (hypericin) was a safe and effective means of reducing experimental PVR.Citation16,Citation17 Our previous studies showed that ten isoforms were present in cultured human RPE cells,Citation20 and they also demonstrated that only PKCα affects cell cycle progression and proliferation in human RPE cells.Citation21 Moreover, PKCα was abundant and further selected to treat PVR.
Due to the limitation of the PKCα inhibitor, siRNA is a very popular method for inhibiting the PKCα. Instability is a major obstacle in RNA therapeutic applications. Without any chemical modification, the siRNA is easily degraded by nuclease and its internal half-life is short, so it cannot effectively inhibit the expression of the target gene. It is feasible to appropriately chemically modify chemically synthesized siRNA. After chemical modification, the stability of the siRNA can be enhanced and the internal half-life prolonged. This can effectively inhibit the expression of the target gene and help achieve gene therapy. Therefore, to enhance the stability of the siRNA, in this study we introduced 2’-O-methylation modification when chemically synthesizing the siRNA, and used Polyacrylamide gel electrophoresis for the purification process.
The effective concentration of siRNA-PKCα is crucial to therapeutic targets for PVR. In our previous in vitro studies, RPE cells incubated with 100 nM siRNA-PKCα alone or released from a foldable capsular vitreous body successfully inhibited the expression of PKCα and exhibited the growth rate at about half the rate of the control cells, offering us a new way to prevent PVR.Citation21,Citation27 Based on the above data, five concentrations from 250 nM to 1500 nM (final ocular concentrations: 50 nM, 100 nM, 150 nM, 200 nM, and 300 nM respectively) chosen to determine which concentration was the most effective in the eye. Current data from and – showed that three concentrations of siRNA-PKCα (500 nM, 1000 nM, and 1500 nM) can partly inhibit PVR in mice, and that 1500 nM is the most effective among the three concentrations.
Delivery of siRNA can be used in vitro and in vivo to target specific RNAs and to reduce the levels of the specific protein product in the targeted cells. The style of transfection is very important for the siRNA. Lipofectamine 2000 and retrovirus were commonly used as gene carriers. In our study, Lipofectamine 2000 was used to transfect siRNA-PKCα released from a foldable capsular vitreous body into human RPE cells, and it successfully inhibited the expression of PKCα.Citation27 Virus-mediated delivery of siRNA would sustainably replicate in in vivo, and would induce long-term lower levels of mRNA, which is not good for normal pathologic recovery. Electroporation is the most widely used physical method and can change the permeability of membranes to make exogenous genes enter the cell. The exact mechanism is unknown, but it is supposed that a short electrical pulse disturbs cell membranes and makes holes in the membrane through which nucleic acids can pass. Because electroporation is easy and rapid, it is able to transfect a large number of cells in a short time once optimum electroporation conditions are determined.Citation35 It has its advantages, including high efficacy, simplicity, ease of practice, good repeatability, safety, and wide application when compared with other methods.Citation36 Therefore, many important targeting genes can be delivered, including vascular endothelial growth factor (VEGF) and hypoxia-inducible factor-1.Citation37–Citation42 For example, Reich et alCitation37 reported that siRNAs directed against human VEGF effectively and specifically inhibit hypoxia-induced VEGF levels in human cell lines after adenoviral-induced human VEGF transgene expression in vivo. Our results also showed that high-concentration siRNA-PKCα delivered by electroporation has good knockdown efficacy at gene and protein levels ( and ). PVR can be partly inhibited by high concentrations of siRNA-PKCα injection; thus, electroporation was involved in this study. A further study is in progress to optimize siRNA-PKCα and evaluate its pharmacokinetics in the much bigger eyes of rabbits or monkeys.
In conclusion, gene therapy with siRNA-PKCα could effectively inhibit PVR in mice and provide us with a novel therapeutic target of PVR.
Acknowledgments
This study was supported by the National Science and Technology Program of People’s Republic of China and funded by the Ministry’s 12th Five-Year Plan (2012BAI08B00).
Disclosure
The authors report no conflicts of interest in this work.
References
- GrigoropoulosVGBensonSBunceCCharterisDGFunctional outcome and prognostic factors in 304 eyes managed by retinectomyGraefes Arch Clin Exp Ophthalmol2007245564164917119994
- QuiramPAGonzalesCRHuWOutcomes of vitrectomy with inferior retinectomy in patients with recurrent rhegmatogenous retinal detachments and proliferative vitreoretinopathyOphthalmology2006113112041204716952397
- PastorJCde la RúaERMartinFProliferative vitreoretinopathy: risk factors and pathobiologyProg Retin Eye Res200221112714411906814
- RyanSJThe pathophysiology of proliferative vitreoretinopathy in its managementAm J Ophthalmol198510011881934014372
- AgrawalRNHeSSpeeCCuiJZRyanSJHintonDRIn vivo models of proliferative vitreoretinopathyNat Protoc200721677717401340
- ClemensMJTraynerIMenayaJThe role of protein kinase C isoenzymes in the regulation of cell proliferation and differentiationJ Cell Sci1992103Pt 48818871487501
- NishizukaYStudies and perspectives of protein kinase CScience198623347613053123014651
- KishiHMishimaHKYamashitaUGrowth regulation of retinal pigment epithelial (RPE) cells in vitroCurr Eye Res19941396616687805397
- HarrisMSSakamotoTKimuraHHypericin inhibits cell growth and induces apoptosis in retinal pigment epithelial cells: possible involvement of protein kinase CCurr Eye Res19961532552628654105
- QiaoHSakamotoTHintonDRInterferon beta affects retinal pigment epithelial cell proliferation via protein kinase C pathwaysOphthalmologica2001215640140711741104
- KishiHMishimaHKYamashitaUInvolvement of the protein kinase pathway in melanin synthesis by chick retinal pigment epithelial cellsCell Biol Int2000242798310772766
- MurphyTLSakamotoTHintonDRMigration of retinal pigment epithelium cells in vitro is regulated by protein kinase CExp Eye Res19956066836957641851
- SheuSJSakamotoTOsuskyRTransforming growth factor-beta regulates human retinal pigment epithelial cell phagocytosis by influencing a protein kinase C-dependent pathwayGraefes Arch Clin Exp Ophthalmol1994232116957017531168
- OsuskyRSorianoDYeJRyanSJCytokine effect on fibronectin release by retinal pigment epithelial cellsCurr Eye Res19941385695747956309
- GaoQGeJThe inhibition of Ca2+ influx induced by hypericin in cultured human retinal pigment epithelial cells analyzed by confocal imagingOphthalmic Res200537312813515821349
- TaharaYRSakamotoTROshimaYRThe antidepressant hypericin inhibits progression of experimental proliferative vitreoretinopathyCurr Eye Res199919432332910520228
- GaoQHuiYWangYEffects of hypericin on traumatic proliferative vitreoretinopathy in rabbitsYan Ke Xue Bao200218424024515515769
- NishizukaYThe molecular heterogeneity of protein kinase C and its implications for cellular regulationNature198833461846616653045562
- OnoYFujiiTOgitaKKikkawaUIgarashiKNishizukaYThe structure, expression, and properties of additional members of the protein kinase C familyJ Biol Chem19882636184692769322834397
- YuKMaPGeJExpression of protein kinase C isoforms in cultured human retinal pigment epithelial cellsGraefes Arch Clin Exp Ophthalmol2007245799399917124607
- GaoQTanJMaPPKC alpha affects cell cycle progression and proliferation in human RPE cells through the downregulation of p27kip1Mol Vis2009152683269520011080
- Canto SolerMVGalloJEDoddsRASuburoAMA mouse model of proliferative vitreoretinopathy induced by dispaseExp Eye Res200275549150412457862
- IribarneMOgawaLTorbidoniVDoddsCMDoddsRASuburoAMBlockade of endothelinergic receptors prevents development of proliferative vitreoretinopathy in miceAm J Pathol200817241030104218310504
- ZhangWTanJLiuYLiWGaoQLehmannPVAssessment of the innate and adaptive immune system in proliferative vitreoretinopathyEye201226687288122460468
- TanJLiuYLiWGaoQOcular pathogenesis and immune reaction after intravitreal dispase injection in miceMol Vis20121888790022511850
- KralingerMTKieselbachGFVoigtMExperimental model for proliferative vitreoretinopathy by intravitreal dispase: limited by zonulolysis and cataractOphthalmologica2006220421121616785750
- ChenXLiuYJiangZZhouLGeJGaoQProtein kinase Cα downregulation via siRNA-PKCα released from foldable capsular vitreous body in cultured human retinal pigment epithelium cellsInt J Nanomedicine201161303131121753881
- FrenzelEMNeelyKAWalshAWCameronJDGregersonDSA new model of proliferative vitreoretinopathyInvest Ophthalmol Vis Sci19983911215721649761295
- OzerdemUMach-HofacreBChengLThe effect of prinomastat (AG3340), a potent inhibitor of matrix metalloproteinases, on a subacute model of proliferative vitreoretinopathyCurr Eye Res200020644745310980656
- MandavaNBlackburnPPaulDBRibozyme to proliferating cell nuclear antigen to treat proliferative vitreoretinopathyInvest Ophthalmol Vis Sci200243103338334812356843
- FranckeMWeickMPannickeTUpregulation of extracellular ATP-induced Müller cell responses in a dispase model of proliferative vitreoretinopathyInvest Ophthalmol Vis Sci200243387088111867610
- FranckeMUhlmannSPannickeTExperimental dispase-induced retinopathy causes up-regulation of P2Y receptor-mediated calcium responses in Müller glial cellsOphthalmic Res2003351304112566861
- IsiksoySBasmakHKasapoglu DundarEOzerAExpression of proteins associated with cell-matrix adhesion in proliferative vitreoretinopathy designed by Dispase modelEur J Ophthalmol20071718910317294388
- ChenJGaoQLiuYClinical device-related article evaluation of morphology and functions of a foldable capsular vitreous body in the rabbit eyeJ Biomed Mater Res B Appl Biomater201197239640421442743
- KimTKEberwineJHMammalian cell transfection: the present and the futureAnal Bioanal Chem201039783173317820549496
- BaumCForsterPHegewisch-BeckerSHarbersKAn optimized electroporation protocol applicable to a wide range of cell linesBiotechniques1994176105810627873174
- ReichSJFosnotJKurokiASmall interfering RNA (siRNA) targeting VEGF effectively inhibits ocular neovascularization in a mouse modelMol Vis2003921021612789138
- TolentinoMJBruckerAJFosnotJIntravitreal injection of vascular endothelial growth factor small interfering RNA inhibits growth and leakage in a nonhuman primate, laser-induced model of choroidal neovascularizationRetina200424413213815076954
- XiaXBXiongSQSongWTLuoJWangYKZhouRRInhibition of retinal neovascularization by siRNA targeting VEGF(165)Mol Vis2008141965197318978955
- DejnekaNSWanSBondOSKornbrustDJReichSJOcular biodistribution of bevasiranib following a single intravitreal injection to rabbit eyesMol Vis200814997100518523657
- XiaXBXiongSQXuHZJiangJLiYSuppression of retinal neovascularization by shRNA targeting HIF-1alphaCurr Eye Res2008331089290218853324
- ZhaoWWangYSHuiYNInhibition of proliferation, migration and tube formation of choroidal microvascular endothelial cells by targeting HIF-1alpha with short hairpin RNA-expressing plasmid DNA in human RPE cells in a coculture systemGraefes Arch Clin Exp Ophthalmol2008246101413142218523795