Abstract
With the increasing complexity and difficulty of surgical procedures, there is an urgent need for empirical and high-fidelity surgical teaching tools. Whereas in the past, teaching tools included cadavers and animals, today, with the development of three-dimensional (3D) printing technology, the creation of patient-specific organ models is possible. This technology provides an effective solution for preoperative rehearsal, thus providing surgery residents with a more realistic simulation environment. This study aimed to provide an overview of the use of personalized 3D printing in various types of surgery through a scoping review, outline their bottlenecks, and provide an outlook. Significant advancements have been made in 3D-printed gel organ models for surgical applications. However, future advancements require interdisciplinary collaborations, medical–engineering integration and novel techniques. Addressing challenges in preservation, instrument response, ultrasound performance and mechanical properties is crucial. Enhancing these aspects will improve the capabilities of organ models, benefiting both medical practitioners and patients.
It is crucial to train surgery residents in surgical operations to carry out these surgical treatment procedures safely and successfully in light of the rising difficulty and complexity of surgical procedures in recent years [Citation1,Citation2]. Traditional physical models are limited in variety, expensive and poor in applicability; they can no longer satisfy the enormous industry demand and are unacceptable to the current industry. Traditional biological models are difficult to obtain for long-term preservation, repeated training and risk of contamination [Citation3]. Existing standards based on live animals and cadavers are unlikely to keep up with the growth of da Vinci robotic surgical procedures and the need for standardized competency-based training. Gel models are printed in 3D for surgical training [Citation4], where the models are used to train medical students (medical students are included because they are truly novice learners) and general surgery residents who have never performed surgical operations. Medical 3D printing enables the production of anatomical structures using volumetric datasets, typically derived from computed tomography (CT) scans. This technology facilitates visual examination and hands-on training with tangible models of human anatomy and pathology [Citation5]. 3D printing methods are inexpensive ways to create reproducible and robust models for surgical simulation and training [Citation6]. Despite significant advancements, the current technology in this field still faces several challenges that need to be addressed. One prominent challenge relates to the preservation of the models, as there are limitations in maintaining their structural integrity and long-term stability. Additionally, the response of electrosurgical instruments within these models is not always accurately simulated, leading to a potential discrepancy between the model’s behavior and the real surgical environment. Another aspect that requires improvement is the ultrasound performance of these models. While efforts have been made to replicate the acoustic properties of human tissues, there is still room for enhancement to achieve a more realistic and reliable ultrasound imaging experience.
Moreover, the mechanical properties of the models can be further refined to better mimic the characteristics of actual human tissues. Achieving a more accurate representation of tissue behavior during manipulation and surgical procedures is essential for effective training and skill development. Addressing these challenges will contribute to the advancement of technology, leading to more realistic and comprehensive training models that closely resemble the clinical setting.
We present an overview of current 3D printing technologies and discuss how they can be used to create patient-specific organ models and surgical training models, among other things, with a focus on the materials that can be used in the manufacturing process. The main emphasis is on a summary of the application of flexible materials such as silicone and hydrogel, as well as an outlook on the application of surgical physical and mechanical properties of gel models, simulation of cutting hemostasis (reactivity of electrosurgical instruments), ultrasound performance and preservation issues in several major blocks as well as bottlenecks, and tissue simulation materials that can be used to develop organ models and evaluate the efficacy of surgical procedure methods.
This review incorporates research that includes additive manufacturing (AM) and 3D printing for surgical training applications. Inclusion criteria were publications from January 1999 through July 2023, and all study designs were published in English. First, the techniques and materials used are discussed to give the reader an insight into additive manufacturing and 3D printing. A summary of 3D printed models in various surgical fields is then presented, and finally, some of the current bottlenecks of 3D printed models are explored, their strengths and weaknesses are summarized, and an outlook is provided. The included studies were searched using article databases such as PubMed and Web of Science. The search terms were ‘additive manufacturing and/or 3D printing’, ‘hydrogel 3D printing’, ‘3D printing and surgical training’ and ‘bioprinting’. These articles are listed and read by the authors. Any conflicts in the inclusion of articles were resolved by consulting other experts in the field. A total of 4574 studies were searched and 319 duplicates were removed, the authors screened subjects for titles and abstracts, an initial 120 studies were identified to assess whether the full text met the inclusion criteria and 88 studies were ultimately included.
Gel 3D printing technology
Various 3D printing techniques have recently been developed for a wide range of materials, including metals, ceramics, other inorganic materials and even polymeric materials [Citation7]. Can even be used for in-situ 3D printing of tissues and organs based on the precise positioning of living cells, growth factors and biomaterials [Citation8,Citation9]. The following 3D printing techniques for polymeric materials can be classified: extrusion printing; inkjet printing with low viscosity inks; stereolithography with photopolymerizable prepolymer solutions; and laser-assisted printing methods Each of these 3D printing methods require a different range of material viscosities to successfully build 3D structured hydrogels.
A gel precursor or viscoelastic polymer solution is obtained from a thermoplastic filament and pushed through a nozzle onto a print bed in extrusion 3D printing. As a result, a continuous flow of extruded material is obtained, or cured in a post-printing process (heat or UV irradiation), resulting in a 3D-printed model.
Inkjet 3D printing, on the other hand, necessitates the use of low-viscosity inks that contain only low-mass fractions of polymers and typically have a low molar mass. As a result, printed structures with low elastic modulus and poor mechanical properties will be produced [Citation10,Citation11]. By depositing a sodium hydroxide (NaOH) solution onto polyacrylamide (PAAm) hydrogels, a similar driving strategy based on inkjet 3D printing was developed by Zhang et al. [Citation12], and the resulting hydrogels can be reversibly driven into complex shapes by varying the pH and other solution parameters.
The objects obtained by stereolithography 3D printing are polymerized layer by layer as the print bed moves in the z-direction, allowing the printing of complex geometries and even organ models. The print also has chemistry constraints, as it requires photosensitive monomers capable of sufficiently fast photopolymerization. The high polymerization rate of acrylate monomers makes them a better material for this printing method. Another limitation of this method is that the composition cannot be changed from layer to layer, as with extrusion 3D printing technology. Furthermore, because the preferred medium for hydrogels is an aqueous solution, many photoinitiators are poorly water soluble and thus do not disperse well in the reaction medium, resulting in inhomogeneous polymer networks and materials with low elastic modulus and poor mechanical properties. Two-photon lithography is a subset of stereolithography that uses a pulsed femtosecond laser in the visible or near-infrared range as the light source. Xiong et al. [Citation13] designed a hydrogel actuator consisting of poly (acrylamide-CO-2-acrylamido-2-methyl-1-propanesulfonic acid) as a body of 6 arms, These hydrogel arms bend to the left when immersed in water and reverse the direction of bending when immersed in NaCl aqueous solution. Due to the extremely high cost of such systems, only a few hydrogel actuators based on this technology have been reported thus far, and thus the utility of this technology in this field has not been fully appreciated.
The direct 3D printing method, as the name suggests, uses a 3D printer to print the model itself, but it has several limitations, including model specifications, support material requirements and printing ink requirements. As a result, printing times are extended, and consumables are used more frequently. Material jetting, material extrusion, and reduced photopolymerization are the most commonly used methods. Kuroda et al. [Citation14]. used direct printing’s material jetting method to create a 3D printed model of intrahepatic vessels to aid in liver cancer resection. While both methods, material extrusion and reduced photopolymerization, produce visually perfect models, their rigid nature limits their further application [Citation15]. For example, polyvinyl alcohol (PVA) and Thermoplastic polyurethanes (TPU) polymers are commercially available in material technology, but the softness of the models produced differs greatly from real tissue.
Rather than printing the model directly, the indirect 3D printing method [Citation16] can first print a mold of the model or the parts used to make the model. 3D printed molds are most commonly used to create organ models, as well as some specific models, such as the stomach and blood vessels, The “sacrificial method” is commonly used, in which the mold material used to make the stomach organ model is printed with a 3D printer and covered by other production materials, and then the sacrificial material is dissolved with a specific solvent to obtain the desired organ model. In terms of 3D printing, the representative Fused Deposition Modeling (FDM) technology has significant advantages in the indirect 3D printing method, with simple structure and lower cost, and has faster printing speed under the condition of not requiring precision, but the sacrificial material’s solubility, as well as the solvent’s safety, will be considered.
Both direct and indirect 3D printing methods have advantages. Witkowski et al. [Citation17], directly 3D printed polylactic acid (PLA) liver vessels and tumors with both methods, printed molds with the indirect 3D printing method, and then molded liver models with transparent silicone molds. Both methods can be combined to improve accuracy and reduce costs, and they can be designed with different materials to meet different performance requirements and adapt to different surgical scenarios.
Model material applications in various surgical fields
With the introduction of 3D printing and related software, it is now possible to convert medical images of various organs into physical models. Continuous advancements in 3D printing technologies and materials, in conjunction with a variety of organ modeling methods, organ models continue to play a significant role in the clinical development and application across various surgical procedures. Additionally, these models find utility beyond surgery, including applications in communication and education. Their widespread use demonstrates their value in enhancing medical practice and advancing knowledge in the field. The fact that different parts of the models focus on different aspects is intriguing. As a result, we concentrate on the use of organ models based on specific parts of the human body in the field of surgery, such as neurosurgery, cardiothoracic surgery, urology, hepatobiliary surgery and others. In this section, we will go over how these models are used and what materials they are made of.
Neurosurgery
Brain and spine models are the most common types of neurosurgical models. Faced with the intricate spatial location of nerves, blood vessels, and sometimes tumors, neurosurgery residents frequently need to make accurate judgments and operate with great care before surgery, and any small mistake can have serious consequences [Citation18]. As a result, the fabrication of brain models typically involves the selection of multiple structures and the use of multiple materials.
Ramirez et al. [Citation19] used 3D printed resin models, produced patient-specific brain models using MRI data. The dura mater consisted of a fast-drying silicone resin paste mixed with grey dye. Blood vessels were made using silicone models generated from MRI data. They introduced a fluid containing chili oil resin dye into the vessels in order to simulate pulsatile movement. The model closely resembles the real structure in terms of dimensions and anatomy, making it an effective tool for training neuroendoscopy skills related to 3D perception and navigation.
D’Urso et al. [Citation20] used Stereo Lithography Appearance (SLA) techniques to create the first 3D models of cerebral aneurysms and cerebral arteriovenous malformations (AVMs) in 1999. Depending on the application, 3D-printed neuroanatomical models may include some specific brain structures while simplifying others. One common example is the utilization of skull models that incorporate blood vessels and tumors. These models feature a cavity that accurately represents the shape of the brain, while the skull is designed with openings of varying shapes to enable direct visualization or simulation of internal structures. Plaster is often chosen as the material for these rigid models due to its bone-like properties, making it an ideal choice for creating realistic anatomical representations [Citation21]. Despite the potential drawback of plaster models made by adhesive jetting being prone to breakage, their inherent advantages outweigh these limitations. Kosterhon et al. [Citation22] used multicolor adhesive jetting to direct 3D print physical models of two different structures for chondrosarcoma patients, and these models were used for surgical approach simulation and tumor removal. They also mentioned that these models are useful for medical students and patient education. Creating complex multicolor models can take a long time, especially if accurate simulations are required. Marks et al. [Citation23] also contributed to educational models by 3D printing models of various stages of Alzheimer’s disease for educational purposes. Other rigid materials, such as thermoplastics and photosensitive polymer resins, have been used for direct 3D printing of such models. Scerri et al. [Citation24] printed physical models of Acrylonitrile Butadiene Styrene (ABS) and photosensitive resins using FDM and SLA techniques, respectively, to aid in surgical planning. They replicated blood vessels up to 1 mm thick and discovered that SLA-printed models were more popular with neurosurgery residents. Indirect 3D printing methods are important for soft models. Nagassa et al. [Citation25] created a model of a middle cerebral aneurysm by combining two indirect methods. To print the original structure, they created silicone rubber molds using a photopolymer resin model. The intravascular lumen was then created by casting water-soluble wax as a sacrificial structure over which silicone resin was applied and the wax dissolved. Furthermore, by varying the silicone layer applied to the wax, they were able to vary the aneurysmal vessel wall accuracy. Overall, the 3D printed model has advantages in providing some degree of hands-on training and skill development but has some limitations in simulating hemodynamics and more comprehensive anatomical structures. Wurm et al. [Citation26] utilized PolyJet 3D printing to fabricate aneurysm models. These models were employed as versatile components in various clamping exercises during microsurgical simulations, owing to their inherent flexibility. Neurosurgery residents and trainees can benefit from the model by refining their clamping techniques, as well as enhancing their abilities in clip placement and selection. However, it is important to note that the model lacks the capability for hemodynamic simulations within the aneurysm and does not provide information regarding the surrounding structures and the entry path, including details like the direction of the bridging veins or the lateral fissure. The mechanical response under load was determined using the brain tissue model created by Forte et al. [Citation27] using PVA and vegetable glue (PHY) composite hydrogel (CH), closely matching the strong dynamic and nonlinear responses. The outcomes demonstrated that it was suitable for simulating brain displacement phenomena as well as the response of brain tissue to depression and palpation. It also simulated the mechanical behavior of organic tissues in compression, relaxation, hysteresis, and shear. This model lacks the simulation of neuroanatomical details and does not include major blood vessels or nerves. Gelatin and modified polyvinyl alcohol composite hydrogel (MCH) was used to simulate the mechanical behavior of brain tissue during surgical needle manipulation by Leibinger et al. [Citation28] They compared the properties of friction and viscosity in detail and found that the insertion forces of the two materials were very similar, but that the modified composite hydrogel had lower viscoelasticity. Even though none of the materials under study perfectly mimicked the behavior of biological soft tissues, each type had advantageous properties (stiffness, viscosity, transparency, etc.). 3D printed models have advantages in simulating the mechanical behavior in brain tissue surgery, using different hydrogel materials that can be tuned to specific mechanical properties. However, the selection of appropriate materials to accurately simulate biological tissue behavior remains a challenge. To produce training models and results of higher quality, it is, therefore, necessary to combine multiple materials and choose the appropriate material for the precise design of the corresponding part on the tissue properties and manufacturing characteristics.
Cardiothoracic surgery
Recent advancements in 3D printing technology have significantly broadened the scope of elastomeric materials that can be utilized. Notably, the development of 3D printable elastomeric materials has revolutionized the field by offering materials with lower Young’s modulus and excellent elasticity, surpassing the capabilities of earlier 3D printed materials [Citation29]. These rubber-like materials exhibit enhanced flexibility and resilience, opening up new possibilities for a wide range of applications. The elasticity and flexibility of these materials are due to the reconfiguration and covalent cross-linking of the long polymer chains. Compared with rigid plastic materials, 3D-printed organ models made from such materials provide a haptic sensation closer to that of real organs. Therefore, they allow the surgeon to perform different precession operations on them, such as shearing, pressing and suturing.
Yang et al. [Citation30] employed the PolyJet process to print heart models using the Tango family of photopolymers, incorporating various colors to differentiate different parts of the model. The study suggests that the printed model can be utilized for training purposes such as expanded septal myotomy and surgical disassembly, providing improved visualization of the model’s geometry. However, it is important to note that the article lacks direct visual evidence, such as images or video data, to support these claims. Further exploration and verification may be necessary to validate the model’s effectiveness in these specific training scenarios. Using the PolyJet method, Bustamante et al. [Citation31] created a 3D-printed photosensitive resin tracheobronchial tree model. The models were examined using a flexible fiberoptic bronchoscope, and it was discovered that the images produced by the models matched the view of the organ as they would appear during lung isolation. These models are anticipated to increase medical professionals’ familiarity with endoscopic bronchial anatomy and assist them in overcoming lung isolation challenges. However, the production cost is high, there are some flaws in structural details, and pre-imaging resolution still needs to be improved. Costello et al. () [Citation32] successfully printed a combination material model with good texture by combining transparent resin material and silicone rubber material, which preserves the texture and structural integrity of the heart model while adapting to scalpel blade cutting and standard suture stitches around Ventricular septal defects (VSD) lesions suitable for intracardiac repair. VSD can be observed using atriotomy, ventriculotomy and pulmonary arteriotomy, allowing students to learn about each defect using all three techniques. However, one significant limitation is that when using 3D printing technology for cardiac dissection, it is currently difficult to accurately depict heart valve details. Overall, according to the passage, 3D printed models offer advantages in cardiac anatomy simulation and surgical teaching, with the ability to render cardiac structures and operational details. However, there are certain limitations, especially in depicting the details of the heart valves that remain challenging. By using 3D printing to create muscle and vascular core molds, and by building a model of the vascular arteries in the inguinal region through the silicone casting method, O’Reilly et al. [Citation33] taught anatomy to medical students and intubation methods to interventional radiologists, making the simulation more physiological by introducing pulsatile blood flow under physiological pressure. However, there is no objective evidence that practicing with 3D femoral models improves operative skills. Furthermore, despite efforts to control flow and pressure, the femoral artery waveform did not show a significant rise, and the flow velocity distribution between arteries and veins was significantly different. Mashiko et al. [Citation34] used 3D printing to create a model of a vessel and aneurysm and then impregnated it with liquid silicone using ABS as a prototype model material. The ABS material was removed after the silicone hardened by dissolving it with xylene, leaving the outer layer as an elastic silicone vascular and aneurysm model. In terms of clamping orientation, clip selection and aneurysm shape during the actual surgery, the model can help create a training environment that is nearly identical to hands-on experience. However, the model fails to simulate the adhesion of rigid vessel walls and vessels, making accurate replication of the thickness and elasticity of artery and aneurysm walls difficult. Abdel-Sayed et al. [Citation35] used a FAB@Home 3D printer to rapidly fabricate a compliant real-size silicone human aortic root anatomy model, which is very fast and relatively simple to achieve in just one step compared with casting methods and then improved its mechanical properties for training in transapical valve stent delivery by dispersive silicone impregnation. A suitable silicone model of the artificial heart was also printed using the FAB@Home 3D printer, and it was treated in the same way to improve its mechanical properties, thereby replacing the use of animals for surgical training in transapical AVR procedures [Citation36]. These models, however, have limitations in terms of production details and precision, and they do not use specific ink materials to precisely mimic the properties of the tissue. But it’s a huge leap from animal cadavers to 3D-printed silicone models for surgical training. Saba et al. [Citation37] created highly realistic, non-biohazardous tissue models that could be dissected, cauterized and sutured using 3D printed molds and PVA hydrogel injection molding. The models were used to perform left anterior descending bypass grafting with a proximal aortic anastomosis after being filled with PVA hydrogel and processed to replicate the mechanical properties of porcine myocardial and aortic tissue. The model, however, lacks objective performance comparisons and insufficient data to support the performance conditions it achieves. Atlan et al. [Citation38] used PVA hydrogel and silicone to create rat carotid artery and abdominal aortic vessel models, and randomized groups were trained in the microvascular anastomosis, patency testing, the intrinsic quality of the anastomosis, posterior wall suturing, anastomotic leakage, and intraluminal endocardial flaps. Both students and trainers agreed that the PVA vascular model is more like the rat aorta than the silicone vascular model, that it better reflects rat vasculature, and that it is a good material to replace silicone tubing. and help to shorten the learning curve of microsurgery by improving the training effect based on objective assessment. Hinton et al. [Citation39] used suspension printing (Fresh printing) to create a hydrogel embryonic heart, brain and blood vessels, among other things. They demonstrated Fresh Printing’s unique ability to print hydrogels with complex internal and external structures, as well as accurate tissue data. Mirdamadi et al. [Citation40] used alginate hydrogels as a substrate to construct a full-size adult heart model and demonstrated that alginate hydrogels can Fresh print smaller but complex organ structures with high fidelity and mechanically characterize them to determine the material components and printing parameters that most closely resemble heart tissue to assess its mechanical properties and construct anatomically accurate tissue models that offer potential applications. Although fresh printing has shown great promise, it is still not used in the production of training models for use, and the time andcost of printing are prohibitively expensive.
(A) Segmentation of anatomic structure/region of interest. (B) Creation of stereolithography file and computer-aided design model. (C & D) Three-dimensional model created by 3D printer.
Reproduced with permission from [Citation32] © SAGE publications (2014).
![Figure 1. 3D printing in cardiothoracic surgery. (A) Segmentation of anatomic structure/region of interest. (B) Creation of stereolithography file and computer-aided design model. (C & D) Three-dimensional model created by 3D printer.Reproduced with permission from [Citation32] © SAGE publications (2014).](/cms/asset/1ad60d22-ab74-4f52-9d1c-52cfd2fd8c5c/idpm_a_12339936_f0001.jpg)
Urology
Urology is a relatively new specialty with a long history that focuses on the kidney, bladder, and urethra, as well as other related organs. 3D urology models are still in their early stages, but kidney-related models have been well-represented in various surgical simulations and have advanced significantly.
Kusaka et al. () [Citation41] used the PolyJet printing process to create 3D printed kidney transplant and pelvic models, primarily using different colors of Tango series photopolymers for renal arteries, veins, urinary tract structures and so on. The models have been used successfully for pre-operative kidney transplant planning and accurate simulation of the surgical procedure. However, due to CT imaging errors, the model has certain accuracy deficiencies and is costly to produce. Bruyère et al. [Citation42] used silicone material to rapidly print a kidney model and an abdominal cavity then inserted a balloon between the kidney and the abdomen, connected the balloon to a ventilator to simulate kidney movement during respiration, punctured the lower caliber under fluoroscopic guidance, and inserted a guide wire to allow for dilation and placement of the access sheath. Finally, the stone was grasped and extracted via a nephroscope. The model’s disadvantage is that it does not accurately reproduce the expansion of the renal cavity and does not adequately simulate the thickness of the abdomen. These shortcomings may have some impact on the realism and usefulness of simulated surgery. Improving the design and material selection of the model may help to improve its simulation capability and reliability. Adams et al. [Citation43] on the other hand, used a combination of 3D printing and casting techniques to create a kidney model that was complete, smooth and non-destructive. They printed the kidney model using a common resin for the outer mold and wax for the inner mold, creating a complete mold that was then filled with silicone for molding. However, the entire mold fabrication and dissolution process takes time, which reduces productivity. Better results were obtained using a combination of direct printing and indirect printing. However, the disadvantage is that the mold making, and dissolution process takes longer time, which may affect the efficiency of production. Improving the process and procedures to increase the speed and efficiency of manufacturing may be the key to solving this problem. Golab and Monda et al. [Citation44,Citation45] used 3D-printed molds to create silicone kidney tumor models for laparoscopic tumor resection and suture surgery training before the actual surgery, and they validated the models’ appearance and internal structure. Many nuances of robot-assisted laparoscopic partial nephrectomy (RALPN), however, were not addressed. Marker identification, kidney visualization, lung hilar clearance, urinary leakage, bleeding management, and pelvic reconstruction, for example, are all critical aspects of RALPN that have not been demonstrated in the model.
The 3D printed donor organ kidney replicas.
Reproduced with permission from [Citation41] © Elsevier (2015).
![Figure 2. 3D printing in urology.The 3D printed donor organ kidney replicas.Reproduced with permission from [Citation41] © Elsevier (2015).](/cms/asset/e8950ece-928a-46e4-a1a6-042da45e6bd7/idpm_a_12339936_f0002.jpg)
Turney et al. [Citation46] utilized a novel approach in which they 3D printed models using soluble resin, followed by immersing them in silicone fluid until the silicone cured. Subsequently, the internal model was dissolved, leaving behind a hollow silicone collection system. This system was then filled with contrast material, sealed, and covered with a dense foam layer to mimic tissue surrounding the kidney. The resulting silicone percutaneous nephrolithotomy (PCNL) training model accurately replicates the anatomy and positioning of the human kidney collection system. It serves as a safe and effective training tool for practicing precise fluoroscopically guided PCNL procedures, providing a realistic simulation environment for trainees. However, the current model composition is not well suited for ultrasound imaging, and while the model can tolerate multiple punctures, the contrast agent leaks out of the model and cannot be reused once the Gastrointestinal (GI) tract is dilated. Cheung et al. [Citation47] created and validated a pediatric laparoscopic pyeloplasty model with the kidney, a replaceable dilated renal pelvis, a ureter obstruction at the pelvic-ureteral junction, and a covered peritoneum using 3D printing and silicone casting. To provide a more realistic and comprehensive training experience, the peritoneum and vascular circulation system are used. However, uneven or inaccurate replication of organ wall thickness and the feature size is a problem. In a different study, Von Rundstedt et al. [Citation48] used 3D printing to create patient-specific silicone kidney models for preoperative planning and surgical rehearsal before RALPN. The models provided accurate information on the removal time and volume of removed tissue and achieved good agreement with actual surgical outcomes, demonstrating the efficacy of model training. According to the claims, the inks mimic normal kidney and tumor tissue characteristics. No characterization outcomes were offered, though, to bolster this assertion. The simulation of the model also failed to be as accurate as needed because this operation was carried out under thermal ischemic conditions with the renal artery clamped, so the simulation does not very accurately reflect the resection time. Melnyk et al. [Citation49] created kidney models for RAPN simulation and training using a combination of 3D printing and PVA hydrogel casting. To create a high-fidelity, standardized, anatomically accurate kidney model, the functional effects of bleeding and suturing were also compared between fresh porcine kidneys and kidneys with different formulations of PVA models. The Young’s modulus of the material was also quantified and compared using multiple mechanical testing methods. The drawback is that only low strains were tested, and higher strains were not tested for changes in properties. To enable preoperative and robotic training surgical simulations, Maddox et al. [Citation50] used agar gel materials to create 3D physical models of kidney structures with properties that are similar to those of kidney tissue. They successfully carried out surgical operations like partial nephrectomy and kidney suturing. The renal parenchyma of these models lacks the fine arterial and venous branches that may be found during RAPN, as well as the perirenal fat and active blood supply, which presents a challenge in the identification and dissection of the vascular system. It is also difficult to quantify the accuracy of tissue processing. Li et al.‘s decision [Citation51] to use PVA during fabrication was based on a comparison of the mechanical characteristics of the tissue organ with that of the material subject to stress and deformation (hydrogel for the prostate and bladder, silicone for the rectum, medial obturator muscle, anal levator muscle, etc.). To accurately mimic the human body and stimulate the living environment of the human body, the combination produced a pelvic organ group model that was used for preoperative experimental simulation of prostate brachytherapy. From this simulation, it was possible to gain some useful insertion experience and greatly increase surgical accuracy. However, there is still a lack of accurate simulation because there is a lack of detailed control data on the properties of the simulated material in terms of fatigue and creep, tribology, damage and adhesion.
Hepatobiliary & pancreatic surgery
Liver transplantation, like kidney transplantation, is a critical procedure in certain cases. In order to optimize surgical protocols for living liver transplantation, Zein et al. [Citation52] employed Computed Tomography (CT) and Magnetic Resonance Imaging (MRI) contrast-enhanced data, along with the Polyjet method, to directly 3D print the first complete liver model (LDLT) using multiple materials. Notably, the researchers carefully selected appropriate coding colors to effectively stain various vascular structures, enhancing the visual representation of the model. It should be noted that when utilizing indirect 3D printing techniques for creating liver models, a wide range of materials can be utilized to achieve the desired outcomes. Pediatric living liver transplantation (LDLT) was the main focus of Wang et al.’s [Citation53] research, and they selected photopolymerization technology to directly print liver molds while casting abdominal models using silicone with a tissue-like feel. The risk of the large-size syndrome and graft reduction in young infants was found to be lower when physical liver models were used, according to their findings. Recently, Ishii et al. and Kuroda et al. [Citation54,Citation55] created liver models with flexible and transparent parenchyma using the polyurethane casting method. The former model was created as a transparent, hard shell for intraoperative liver transplantation navigation using a photopolymerization printing technique. The second model, built upon previous research [Citation56], incorporated a plaster liver parenchyma model created through 3D printing for the purpose of planning and simulating LDLT. One key aspect of 3D-printed liver models is the inclusion of vascular structures. Accurately identifying the critical locations of blood vessels during complex liver surgeries facilitates the process of vascular reconstruction, ultimately enhancing surgical outcomes.3D printed models have potential for use in liver surgery. Transparent hard-shell models and plaster liver parenchyma model casts offer the benefits of visual navigation and surgical planning, respectively. For complex liver surgery, accurate vascular location is important for a successful procedure, and 3D printed liver models can help achieve this goal. To print other structures, such as the gallbladder, Huber et al. [Citation57] used ABS filaments and material injectors to create thin-walled liver tissue. This liver model aided in the application of complex vascular reconstruction during surgical planning. By combining FDM printing of ABS molds with silicone casting, Casa-Murillo et al. [Citation58] was able to create a low-cost, high-quality teaching anatomical model of the biliary tree for training in laparoscopic cholecystectomy, but the model did not depict more challenging scenarios, such as rare bile duct variants or a lack of fibrous tissue material to simulate the inflammatory platform of fluid collection. By changing the parameters of the hydrogel manufacturing process, PVA’s mechanical elasticity was made to be very similar to that of the human abdominal aortic aneurysm wall, intraluminal thrombus ILT, and abdominal fat. Overall, these studies demonstrate the application of 3D printing in creating structures such as the gallbladder, liver tissue, and anatomical models for training purposes. While there are notable advancements in replicating certain characteristics, limitations still exist, such as the lack of representation of rare variations or specific tissue properties. Further research and development are necessary to address these limitations and improve the fidelity and functionality of 3D printed models in various medical applications. He et al. [Citation59] developed an advanced human model (AMM) that accurately replicated the primary mechanical, anatomical, and pathological characteristics of abdominal aortic aneurysms (AAA) and their surrounding tissues. Notably, a specialized sealing system was devised to maintain intraluminal and abdominal pressure while enclosing adjacent tissues such as the spine and abdominal fat. Through comprehensive experiments investigating the biomechanical limitations during endovascular aneurysm repair (EVAR), the significance of accounting for the surrounding tissues in AAA deformation during EVAR was emphasized. This model provides a valuable in vitro environment for medical training. However, it should be noted that removing the inner mold may cause minimal damage to the model and result in hydrogel tearing under pressure. Overall, He et al.‘s study demonstrates the development of an advanced abdominal aortic aneurysm model that faithfully represents relevant mechanical and anatomical characteristics. The inclusion of surrounding tissues and the evaluation of their impact on EVAR contribute to improved training and understanding in the field of abdominal aortic aneurysm management. Kwon et al. [Citation60] used 3D printing and silicone casting to create a specialized endoscopic retrograde cholangiopancreatography (ERCP) silicone training model. The model was reasonably priced, simple to build, and enabled the user to perform a variety of specialized ERCP techniques as well as repetitive training. However, no model was created with all parts of the esophagus, stomach, and duodenum in the same order as humans, and the material’s surface tension was higher than that of biological tissues and too transparent. Jong et al. [Citation61] used PVA hydrogel as a liver-mimicking material to create a liver model, adjusted the material concentration and process method to adjust the model’s tissue properties, and investigated the PVA mold imaging properties and needle-tissue force properties through data comparison to design high-quality, high-simulation kidney models. However, there are no design comparisons in terms of viscoelasticity and realistic environmental manipulation. Witowski J et al. () [Citation62] used 3D printed liver models to assist with surgical planning for significant and complex resections; they can also be used as a quality control device for tumor resections, to discuss the approach or feasibility of the surgery, etc. This is thought to be a useful area for students, surgeons, and patients; however, there are still challenges with the method, and it is a relatively expensive one. In the future, there may be more advancements in the material.
Development of 3D printed liver models. (A) Raw CT axial image. (B) CT image with segmentation masks overlay (green, tumor; purple, portal vein; blue, inferior vena cava). (C) Surface rendering of segmented meshes. (D) 3D printed liver model.
Reproduced with permission from [Citation62] © SpringerLink (2020).
![Figure 3. 3D printing in hepatobiliary and pancreatic surgery.Development of 3D printed liver models. (A) Raw CT axial image. (B) CT image with segmentation masks overlay (green, tumor; purple, portal vein; blue, inferior vena cava). (C) Surface rendering of segmented meshes. (D) 3D printed liver model.Reproduced with permission from [Citation62] © SpringerLink (2020).](/cms/asset/0430afd2-e4b2-4348-bf55-503e43ce120b/idpm_a_12339936_f0003.jpg)
Production process of gel models for surgical applications
To achieve high fidelity and efficiency in simulation training in surgical training, the model’s realistic physical and chemical properties must approximate human tissues [Citation43]. As a result, Stereolithography (STL) format files must be extracted from CT for 3D printing in various gel materials, including direct 3D printing () and indirect 3D printing. (). Hydrogels are gels made up of cross-linked hydrophilic polymers. They can bind large amounts of water, similar to soft tissues in the human body, depending on the density of the cross-links and the nature of the repeating units. For example, Duan et al. [Citation63] used an alginate/gelatin hydrogel for 3D bioprinting of heterogeneous aortic valve catheters; Markstedt et al. [Citation64] used a bioink composed of cellulose nanoprimary fibers and alginate for 3D bioprinting of human chondrocytes, and Fei Gao et al. [Citation65] designed a high-strength biohybrid scaffold. The hydrogel-based human tissue and organ model has significant advantages in the construction of a highly simulated surgical training system by realistically simulating the physiological environment and interfacial material exchange of tissues and organs, and during the fabrication process, researchers explored its acoustics and mechanical strength to achieve realistic effects.
(A) The general process of making an organ model. (B) General steps for indirect printing.
STL: Stereolithography.
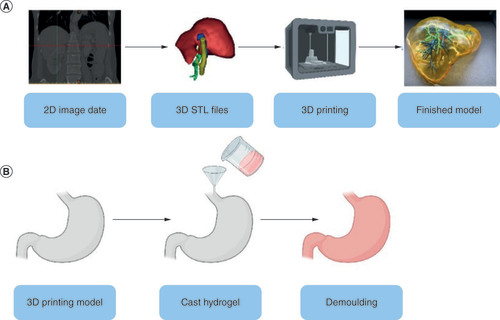
In their research, Gao et al. [Citation66] developed a specialized 3D printing ink using gelatin methacrylate and nano clay. By extrusion 3D printing with the application of shear force, this ink successfully generated stable hydrogel filaments. Under the precise control of a computer system, the team was able to fabricate organ structures such as a bionic ear and branching blood vessels. The incorporation of nanoclay in the ink formulation significantly enhanced the mechanical properties, shape accuracy, and biocompatibility of the printed models. It should be noted, however, that further advancements toward individualized treatment still require additional time and research.
Pepley et al. [Citation67] polyvinyl chloride (PVC), softeners, and mineral oil were thoroughly mixed in a pan under a fume hood, then slowly heated to a specific temperature, and stirred to ensure uniform heating and homogeneous mixing. When the material thickened and changed from milky to translucent, it was removed from the heat source and poured into the 3D printing mold of choice. The molds were left at room temperature to cool naturally. When making multiple layers of modified PVC, they wait until the first layer has naturally cooled and hardened, but is still warm, before pouring another layer. One cannot wait for complete cooling and hardening; residual heat improves layer adhesion, and hardening prevents the top and bottom layers from mixing. The multi-layer structure allows for the differentiation of different soft tissues as well as the differentiation of different printed parts and details of the organ model. In general, this method provides a way to prepare modified PVC material suitable for 3D printing, allowing for the creation of complex organ models with differentiated soft tissues and intricate details. The authors’ approach demonstrates attention to optimizing layer adhesion and preserving the integrity of each layer during the printing process.
Aoyagi et al. [Citation68] mixed sodium alginate, ethanol (for better sodium alginate dissolution), and glycerol (to increase scattering and improve ultrasound fidelity) and stirred until the sodium alginate was uniformly dispersed in the solution. Following that, in vessel 2, calcium sulfate dihydrate (to provide calcium ions), trisodium phosphate dodecahydrate (retarder), and water were mixed and stirred until calcium sulfate dihydrate was uniformly dispersed in the solution. The solution in container 2 is then poured into container 1 and stirred until it becomes viscous but retains some fluidity. Finally, the solution is poured into a mold and aged in a constant temperature incubator at 37°C for 24 h to allow calcium alginate to gel and harden into the shape of the mold (which can be designed in various shapes that require training). The single sodium alginate, on the other hand, is not mechanically strong enough and does not respond well to electrosurgical instruments. It is worth noting that the use of single sodium alginate alone does not provide sufficient mechanical strength, and it may not respond well to electrosurgical instruments. Hence, the addition of other materials and the specific preparation method described by Aoyagi et al. significantly improved the mechanical properties and suitability of the gelatinous material for training purposes. This method offers a practical approach to creating gelatinous materials for 3D printing, providing improved mechanical strength and compatibility with electrosurgical instruments. The described process allows for the production of customized training models with desired shapes for various training needs.
Kumar et al. [Citation69] synthesized polyacrylamide hydrogels in deionized water at room temperature (22°C) by first placing an aqueous solution of acrylamide in a mold, using N, N-methylenebisacrylamide (MBAA) as a cross-linking agent, then adding fine particles of titanium dioxide (TiO2) to control the echogenicity of the gel, then degassing the solution for 15–30 min, adding After degassing the solution for 15–30 min, ammonium persulfate (APS) was added as the gel initiator and tetramethylethylenediamine (TEMED) as the accelerator, and the solution was poured into a container and placed in an ice water bath for about 10 min. The elasticity of the model can be altered by adjusting the acrylamide concentration to cover a wide range of normal to pathological tissue elasticity, which has a very high accuracy under ultrasonography and can be considered one of the available diagnostic tools to improve the diagnostic accuracy of breast and thyroid tumors. However, they are vulnerable to dehydration and biological attack, necessitating frequent material updates and adding to training costs, as well as inaccuracy and uncertainty in their effectiveness. The study highlights the synthesis of polyacrylamide hydrogel models with tunable elasticity for diagnostic purposes. While they offer advantages in terms of ultrasound visibility and elasticity adjustment, challenges related to material durability and effectiveness should be addressed for their widespread adoption in training and diagnostic settings.
He et al. [Citation70] first dissolved Poly(vinyl acetate) (PVA-C) powder in deionized water before completely dissolving it in 100°C water to obtain a 10% mass fraction PVA solution, and they discovered that the 10% mass fraction had a lower viscosity than the 15% mass fraction PVA solution, allowing for the release of air bubbles during the molding step, as well as better mechanical properties. It is injected into the mold after cooling and cryogenically treated in a temperature-controlled refrigerator. The cryogenic treatment was designed in the following manner: each cycle was frozen to -20°C at a rate of 0.333°C/min, then allowed to stand for two h, then thawed to 10°C at a rate of 0.08°C/min and stopped for 2 h. They discovered that repeated thermal cycling of PVA-C increased the hydrogel’s crystallinity, thereby increasing its elastic modulus. To determine the optimal number of freeze-thaw cycles for each application PVA-C models with the same low-temperature treatment but different numbers of freeze-thaw cycles were created to model the vein wall, neonatal brain, and abdominal fat, among others. This material allows for a wide range of tissue models, but it is susceptible to biological invasion and loses stability over time.
Elvira et al. [Citation71] froze PVA solutions at -20°C for 5 h before thawing them at 5°C. After the first layer has been cured by one freeze-thaw cycle, a second solution layer with different additives can be placed on top of the cured layer for another freeze-thaw cycle to ensure that the second layer gels and bonds to the first layer. In this manner, the third layer can be bonded to simulate various soft tissues. Single PVA 3D printed hydrogel organ models suffer from mechanical property limitations, biocompatibility issues, lack of diversity and tissue specificity. These limitations need to be considered in practical applications and solutions need to be found to improve the accuracy, stability and feasibility of the models.
Mechanical properties of the gel model
A series of studies have been conducted to enable gel models to achieve the mechanical properties of real tissues with the same characteristics as the tissues being studied.
Adams et al. [Citation43] compared previously reported 3D printed kidney models in the Urology Teaching and Training System to their new 3D printing method, which allowed them to use a wider range of materials, including those with similar elastic modulus to kidney tissue. The human kidney’s anatomical structure and mechanical properties are more precisely replicated, allowing mechanical properties to realistically mimic those of human tissues and organs. In future experiments, these models can be used to simulate percutaneous diagnostic and therapeutic methods such as biopsy and percutaneous nephrostomy. It also helps to develop and test imaging techniques like ultrasound, MRI and CT.
De Jong et al. [Citation72] conducted experiments to evaluate the forces exerted on Polyvinyl Alcohol (PVA) samples with different mass percentages and freeze-thaw (FT) cycles. These forces were compared with those observed in isolated human liver tissue. By varying the PVA-to-water mass percentages (4 and 7%) and the number of freeze-thaw cycles (1, 2 and 3 cycles), the researchers simulated the conditions and performed needle insertions into the PVA samples. Specifically, they used an 18-gauge needle inserted 13-times at a speed of 5 mm/s. Similar experiments were conducted in isolated livers to measure the axial forces on the needle during insertion and retraction. Based on their findings, the 4% PVA gel model with two freeze-thaw cycles exhibited comparable characteristics to the human liver. The mechanical properties of PVA hydrogels can be adjusted by varying the PVA concentration and the number of freeze-thaw cycles, allowing for the simulation of liver tissue characteristics. Overall, the study highlights the potential of PVA hydrogels as a material for simulating liver tissue characteristics in experimental settings. The control over PVA concentration and freeze-thaw cycles offers a means to tailor the mechanical properties of the hydrogels, enhancing their suitability for specific applications requiring liver tissue simulation.
Leibinger et al. [Citation73] performed fracture tests on gelatin, CH, and MCH to characterize the behavior of all model and real tissues at failure, and then measured the insertion force required for the brain, MCH, and gelatin; due to the lack of transparency of biological soft tissues, they examined the internal displacement and strain close to the needle using laser-based imaging for the model, allowing for a comprehensive measurement of the interaction between the inserted needle and the substrate. And, after comparing their measurements to those of real soft tissue porcine brain, they concluded that gelatin, CH, and MCH are all suitable as realistic substitutes for real soft tissue, To achieve a more realistic effect, the concentration of gelatin and composite hydrogel must be adjusted to match the properties of soft tissue.
Bottlenecks in the gel organ model
Significant progress has been made in 3D-printed organ models and their corresponding surgical applications in recent years. However, there is still a lot of room for improvement in the various gel organ models, with a lack of electrosurgical instrument responsiveness, insufficient ultrasound mimicry, and a variety of issues with subsequent gel preservation. Many investigations and efforts have been made by researchers to address this series of bottlenecks.
Responsiveness of electrosurgical instruments to gel models
Electrosurgery is now used in over 90% of surgical procedures. It is also the preferred method for preventing bleeding during laparoscopy [Citation74]. Electrosurgical devices have been utilized in surgical procedures for over a century to perform precise cutting and control bleeding through the process of coagulation.
Amiri et al. [Citation75] used fat, water, and agar/gelatin to create a conductive tissue mimetic material (TMM). Anatomically relevant heterogeneous breast models representing the optical properties of different layers of the human breast were created using this developed formulation and 3D printing molds. The TMM they created is similar to human tissue in that it has enough ions or conductivity. During electrosurgery, the tissue temperature can rapidly increase to levels above 100°C, leading to local tissue cutting and coagulation [Citation76]. However, it is important to note that exposure to high temperatures can also cause excessive melting of certain compounds. Their TMM breast model does not melt heavily at high temperatures during electrosurgery, but instead cuts (burns) cleanly, similar to human tissue. TMMs are better suited for demonstrating realistic tissue effects, burning, and debris formation during electrosurgery. Because most polymers are not electrically conductive, they require more additives, are more difficult to fabricate, change shape over time, are susceptible to biological invasion, and are more expensive. The TMM’s conductivity, clean cutting behavior, ease of fabrication, and cost-effectiveness make it a valuable tool in the field of surgical simulation and training.
Application of gel models in ultrasound
Acoustic properties similar to those of real tissues are required for gel tissue substitutes used in ultrasound imaging; the most critical acoustic properties are sound velocity, characteristic acoustic impedance, attenuation, backscattering coefficient and nonlinear parameters. The strength and elasticity of real tissue are affected by several factors, including age, health and the individual’s current state [Citation77]. Real tissue uncertainty, on the other hand, reflects the importance of models, which can be used as a training modality for sonographers and as a test for ultrasound systems but are not available in human, cadaveric or animal models.
Aoyagi [Citation78] used cheaper sodium alginate to create a sodium alginate gel model by die-casting through a 3D printed mold and added different proportions of ethanol to its pre-polymerization solution to modify its ultrasound properties and control the scatter pattern with a texture similar to that of soft parts of the human body, which is suitable for basic ultrasound training and medical student education, but the mechanical properties are poor.
Earle et al. [Citation79] added a small amount of suspended wheat flour to 5% mass fraction agar as a model precursor material and created an agar model that was ultrasound examined and found to produce ultrasound images very similar to real tissues and was easy to make, did not require refrigeration and had several distinct advantages over other hydrogel models for ultrasound training under various conditions. Both studies demonstrate the development of models for ultrasound training with distinct characteristics and benefits. While Aoyagi’s sodium alginate gel model offers a texture similar to soft human tissues, it may have limitations in mechanical properties. On the other hand, Earle’s agar model provides realistic ultrasound images and advantageous properties such as ease of production and storage.
Alves et al. [Citation80] employed the traditional casting technique in plastics to create a gel model using a mixture of bulletproof gel, mineral oil and cellulose. The addition of mineral oil acted as a dispersant, while cellulose provided a scattering medium for subsequent ultrasound imaging. The researchers conducted quantitative measurements of sound velocity and attenuation in comparison to reported data for human biological tissue types. They discovered that the sound velocity and attenuation of the tested gels were similar to the values reported for cardiac tissue. Furthermore, the researchers validated the moldability of these gels by using patient CT imaging data. It developed a cost-effective, reproducible method for designing and assembling a novel, fully molded gel heart model that can be used to simulate ultrasound examinations of the heart. The study highlights the development of a gel model that closely resembles cardiac tissue in terms of sound velocity and attenuation. The cost-effectiveness and reproducibility of the method make it a practical solution for designing fully molded gel models for ultrasound simulation.
Pepley et al. [Citation67] used a molded construction method to fabricate a modified PVC mixture into an ultrasound training model, creating realistic multilayer models by varying the proportions of different fillers between layers, which can be customized to represent different patient types, such as obese or muscular patients. A variety of patient scenarios can be provided to customize the patient’s condition in ways that other model-making materials cannot.
Storage of gel models
Different 3D printing materials currently available have their own characteristics (), and some of them are difficult to store. Water-based gels, such as polyvinyl alcohol, agar, and gelatin hydrogels, have several storage disadvantages, including poor time stability [Citation72], the need for careful storage and maintenance, susceptibility to biological invasion and harboring fungi and bacteria, which makes subsequent training in applied surgery manufacturing difficult. Because of the limitations of the heat deformation properties of 3D printing materials, autoclaving and radiation sterilization are less commonly used.
Table 1. Comparison of different 3D printing materials.
To overcome the limitations of hydrogels, Li et al. [Citation81] optimized the model’s durability by creating gelatin-based hydrogels with 1% chlorhexidine digluconate (which was used to prevent bacterial and fungal invasion due to its antibacterial properties).
Elvira et al. [Citation71] kept PVA hydrogels that had gone through the freeze-thaw cycle in a sealed box at 5°C and added 0.05 wt% chlorhexidine (a disinfectant) to the aqueous solution. As a result, there is a moist environment that prevents deformation or bacterial fungal invasion caused by drying and shrinkage of the PVA, which could interfere with subsequent training use.
Based on the numerous gel materials proposed for training, Cabrelli et al. [Citation82] proposed copolymer-oil gels as an inert and stable material. The glycerol in their oil-based gel was used as a dispersion, changing the ultrasonic as well as mechanical properties of the oil-based copolymer model, using two mineral oils with different viscosities, to characterize the samples in terms of sound velocity, attenuation, and backscatter and density, Young’s modulus in a range of different soft tissue values for applied surgical training, the oil-based gel addresses both the poor temporal stability of water-based gels, which can reduce biological invasion and the need for special environment preservation [Citation83]. Collectively, these studies offer innovative approaches to enhance the durability, stability and usability of gel-based training models. By incorporating antibacterial agents, optimizing storage conditions and exploring alternative gel materials, researchers are continuously advancing the development of gel models for various training applications in the medical field.
Chao et al. [Citation84] tightly applied a hydrocolloid dressing to a gelatin-based hydrogel, a medical bandage used to dry and protect certain types of wounds. It can adhere tightly to the surface of the gelatin matrix and be manipulated on the model without damaging the surface. Although it is effective at preventing biological invasion, it raises the cost, the conditions for applying the dressing are harsh, and mass production is more difficult.
Conclusion & future perspective
To meet the challenge of today’s widespread use of electrosurgical devices, medical students and novice surgery resident must go through standardized training, which ranges from 5 to 10 years for surgical qualification, depending on specialties and regions [Citation85,Citation86], 3D printed surgical models, which replace human cadavers and animal organs, are a logical alternative to training. Therefore, investigating the use of 3D printed models in surgery is a key area that could improve surgical outcomes, increase surgeon experience [Citation87], and reduce surgical errors, benefiting both surgeons and patients. Despite significant progress in this area, we have found that the current bottlenecks in 3D printed hydrogels are mainly in the responsiveness to electrosurgical instruments and the storage of the models, which have not yet been thoroughly investigated. The real responsiveness to human-like tissues of electrosurgical instruments, such as electrocoagulation, which can carbonize the material and thus coagulate blood to stop bleeding, ultrasonic knife to cut substantial organs, coagulate blood vessels, and so on, as well as the longevity and storage aspects of the material, if continued to be explored to find an optimal solution, will undoubtedly impact the medical training and education field in the future and become a boon to doctors and patients. The quality of the model in the method of direct hydrogel precursor ink printing is dependent on the continuous improvement of the printing hardware, and there is still less direct printing. In the future, We believe that the development of stimulus-responsive inks and precursors for the design of more complex and fine 3D printing brakes has a bright future in biomedical reception. In the organ model, the indirect printing method must address the fineness issue as well as the solvent selection of sacrificial materials. Due to the structural complexity of each organization, a single material and manufacturing method may not truly reflect the true details of the actual structural model, which will require a multi-material, multi-method combination process for manufacturing, and a suitable solution in terms of model longevity and convenient storage. The integration of advanced imaging techniques, such as computed CT and MRI [Citation88], can provide detailed anatomical information, allowing for the creation of highly realistic and patient-specific organ models. Furthermore, the utilization of advanced materials, biofabrication techniques and additive manufacturing processes can contribute to the development of organ models that closely mimic the physiological and mechanical properties of real organs. By fostering collaboration between the medical and industrial sectors and facilitating interdisciplinary communication, the field of organ modeling can reach unprecedented levels of advancement in the future. Notably, parameters such as Young’s modulus, tensile and compressive properties, water content, coefficient of friction, rheological properties, electrical and thermal conductivity, as well as ultrasonic elasticity, can be closely replicated in individual organ models, mirroring the characteristics of real organs. These advancements hold significant potential in benefiting medical professionals and patients alike. This collaborative approach allows for the seamless integration of medical knowledge, technological advancements and industrial expertise, leading to the development of more sophisticated and advanced organ models. Ultimately, these advancements will contribute to improved patient outcomes, enhanced surgical techniques, and better healthcare practices.
A broad range of personalized 3D printed gel organ models have already been used in surgical procedures, and they’re revolutionizing medical training, but there are still some bottlenecks.
Gel 3D printing in technology
Three 3D printing techniques, direct 3D printing, indirect 3D printing and a combination of both, are applied to gel printing.
Model material applications in various surgical fields
3D printed gel organ models have applications in neurosurgery, cardiothoracic surgery, urology, hepatobiliary and pancreatic surgery.
Mechanical properties of the gel model
Gel materials mimic a range of mechanical properties such as real tissue stretching, suture cutting, ultrasound performance, electrosurgical instrument reactivity, and more in organ models.
Summary & outlook
Advancing 3D printed gel organ models requires interdisciplinary collaboration, innovation, and addressing key challenges to benefit medical practice and patients.
Author contributions
SJ Yu, XD Xu contributed to this work equally, and J Zhang and ZF Wang defined the focus of the review. XD Xu modified the manuscript. SJ Yu drafted the manuscript. LD Cao, L Ma, JL Mao and H Chen modified it into the final edition. All authors have read and agreed to the published version of the manuscript.
Competing interests disclosure
The authors have no competing interests or relevant affiliations with any organization or entity with the subject matter or materials discussed in the manuscript. This includes employment, consultancies, stock ownership or options and expert testimony.
Writing disclosure
No writing assistance was utilized in the production of this manuscript.
Financial disclosure
This work was supported by the fund of the Subproject of the Key R&D Program of the Ministry of Science and Technology (2018YFB1107104) and the Xinjiang Uygur Autonomous Region Regional Cooperative Innovation Program (2019E0287). The authors have no other relevant affiliations or financial involvement with any organization or entity with a financial interest in or financial conflict with the subject matter or materials discussed in the manuscript apart from those disclosed.
References
- ValdiviesoRF, ZornKC. Urological laparoscopic training – practice makes perfect. Nat. Rev. Urol.11, 138–139 (2014).
- PandaN, MorseCR. Commentary: practice makes perfect in cervical esophagogastric anastomosis. J. Thorac. Cardiovasc. Surg.160, 1611–1612 (2020).
- ChevallierC, WillaertW, KawaEet al.Postmortem circulation: a new model for testing endovascular devices and training clinicians in their use: testing endovascular devices and training clinicians with postmortem circulation. Clin. Anat.27, 556–562 (2014).
- MelnykR, EzzatB, BelfastEet al.Correction to: mechanical and functional validation of a perfused, robot-assisted partial nephrectomy simulation platform using a combination of 3D printing and hydrogel casting. World J. Urol.38, 1643 (2020).
- GiannopoulosAA, MitsourasD, YooS-J, LiuPP, ChatzizisisYS, RybickiFJ. Applications of 3D printing in cardiovascular diseases. Nat. Rev. Cardiol.13, 701–718 (2016).
- CuljatMO, GoldenbergD, TewariP, SinghRS. A review of tissue substitutes for ultrasound imaging. Ultrasound Med. Biol.36, 861–873 (2010).
- WuP, WangJ, WangX. A critical review of the use of 3-D printing in the construction industry. Autom. Constr.68, 21–31 (2016).
- DongH, HuB, ZhangWet al.Robotic-assisted automated in situ bioprinting. Int.J. Bioprinting9(1), 629 (2022).
- FortunatoGM, SigismondiS, NicolettaMet al.Analysis of the robotic-based In Situ bioprinting workflow for the regeneration of damaged tissues through a case study. Bioengineering.10, 560 (2023).
- HongN, YangG-H, LeeJ, KimG. 3D bioprinting and its in vivo applications: 3D bioprinting and its in vivo applications. J Biomed Mater Res B Appl Biomater.106, 444–459 (2018).
- LiuF, LiuC, ChenQet al.Progress in organ 3D bioprinting. Int. J. Bioprinting4(1), 128 (2018).
- ZhangX-Q, WuD, HouJ-Bet al.Multiresponsive reversible deformation of patterned polyacrylamide hydrogel constructed by a computer-assisted dispenser. ACS Appl. Polym. Mater.1, 1187–1194 (2019).
- XiongZ, ZhengM-L, DongX-Zet al.Asymmetric microstructure of hydrogel: two-photon microfabrication and stimuli-responsive behavior. Soft Matter.7, 10353 (2011).
- KurodaS, KobayashiT, OhdanH. 3D printing model of the intrahepatic vessels for navigation during anatomical resection of hepatocellular carcinoma. Int. J. Surg. Case Rep.41, 219–222 (2017).
- JinZ, LiY, YuK, LiuL, FuJ, YaoXet al.3D printing of physical organ models: recent developments and challenges. Adv Sci.8, 2101394 (2021).
- RethyA, SæternesJO, HalgunsetJet al.Anthropomorphic liver phantom with flow for multimodal image-guided liver therapy research and training. Int. J. Comput. Assist. Radiol. Surg.13, 61–72 (2018).
- WitowskiJ, BudzyńskiA, GrochowskaAet al.Decision-making based on 3D printed models in laparoscopic liver resections with intraoperative ultrasound: a prospective observational study. Eur. Radiol.30, 1306–1312 (2020).
- KleinGT, LuY, WangMY. 3D printing and neurosurgery – ready for prime time?World Neurosurg.80, 233–235 (2013).
- EncarnacionRamirez M, RamirezPena I, BarrientosCastillo REet al.Development of a 3D printed brain model with vasculature for neurosurgical procedure visualisation and training. Biomedicines.11, 330 (2023).
- D’UrsoPS, ThompsonRG, AtkinsonRLet al.Cerebrovascular biomodelling: a technical note. Surg. Neurol.52, 490–500 (1999).
- McMenaminPG, HusseyD, ChinDet al.The reproduction of human pathology specimens using three-dimensional (3D) printing technology for teaching purposes. Med. Teach.43, 189–197 (2021).
- KosterhonM, NeufurthM, NeulenAet al.Multicolor 3D printing of complex intracranial tumors in neurosurgery. J. Vis. Exp.155, e60471 (2020).
- MarksM, AlexanderA, MatsumotoJet al.Creating three dimensional models of Alzheimer’s disease. 3D Print. Med.3, 13 (2017).
- ScerratiA, TrovalusciF, AlbaneseAet al.A workflow to generate physical 3D models of cerebral aneurysms applying open source freeware for CAD modeling and 3D printing. Interdiscip. Neurosurg.17, 1–6 (2019).
- NagassaRG, McMenaminPG, AdamsJW, QuayleMR, RosenfeldJV. Advanced 3D printed model of middle cerebral artery aneurysms for neurosurgery simulation. 3D Print Med.5, 11 (2019).
- WurmG, LehnerM, TomancokB, KleiserR, NussbaumerK. Cerebrovascular biomodeling for aneurysm surgery: simulation-based training by means of rapid prototyping technologies. Surg. Innov.18, 294–306 (2011).
- ForteAE, GalvanS, ManieriF, RodriguezY, BaenaF, DiniD. A composite hydrogel for brain tissue phantoms. Mater. Des.112, 227–238 (2016).
- LeibingerA, ForteAE, TanZet al.Soft tissue phantoms for realistic needle insertion: a comparative study. Ann. Biomed. Eng.44, 2442–2452 (2016).
- LoadmanMJR. Analysis of Rubber and Rubber-like Polymers. Springer, Dordrecht, The Netherlands (1998).
- YangDH, KangJ-W, KimN, SongJ-K, LeeJ-W, LimT-H. Myocardial 3-dimensional printing for septal myectomy guidance in a patient with obstructive hypertrophic cardiomyopathy. Circulation132, 300–301 (2015).
- BustamanteS, BoseS, BishopP, KlatteR, NorrisF. Novel application of rapid prototyping for simulation of bronchoscopic anatomy. J. Cardiothorac. Vasc. Anesth.28, 1122–1125 (2014).
- CostelloJP, OlivieriLJ, KriegerAet al.Utilizing three-dimensional printing technology to assess the feasibility of high-fidelity synthetic ventricular septal defect models for simulation in medical education. World J. Pediatr. Congenit. Heart Surg.5, 421–426 (2014).
- O’ReillyMK, ReeseS, HerlihyTet al.Fabrication and assessment of 3D printed anatomical models of the lower limb for anatomical teaching and femoral vessel access training in medicine: subject specific 3D-printed anatomy. Anat. Sci. Educ.9, 71–79 (2016).
- MashikoT, OtaniK, KawanoRet al.Development of three-dimensional hollow elastic model for cerebral aneurysm clipping simulation enabling rapid and low cost prototyping. World Neurosurg.83, 351–361 (2015).
- Abdel-SayedP, KalejsM, Von SegesserLK. A new training set-up for trans-apical aortic valve replacement. Interact. Cardiovasc. Thorac. Surg.8, 599–601 (2009).
- KalejsM, SegesserLKV. Rapid prototyping of compliant human aortic roots for assessment of valved stents. Interact. Cardiovasc. Thorac. Surg.8, 182–186 (2009).
- SabaP, AyersB, MelnykR, GosevI, GhaziA, HicksG. Development of a high-fidelity coronary artery bypass graft training platform using 3-dimensional printing and hydrogel molding. J. Thorac. Cardiovasc. Surg.161, e291–293 (2021).
- AtlanM, LellouchAG, LegagneuxJ, ChaouatM, MasqueletA-C, LetourneurD. A new synthetic model for microvascular anastomosis training? A randomized comparative study between silicone and polyvinyl alcohol gelatin tubes. J Surg Educ.75, 182–187 (2018).
- HintonTJ, JalleratQ, PalcheskoRNet al.Three-dimensional printing of complex biological structures by freeform reversible embedding of suspended hydrogels. Sci Adv.1, e1500758 (2015).
- MirdamadiE, TashmanJW, ShiwarskiDJ, PalcheskoRN, FeinbergAW. FRESH 3D bioprinting a full-size model of the human heart. ACS Biomater. Sci. Eng.6, 6453–6459 (2020).
- KusakaM, SugimotoM, FukamiNet al.Initial experience with a tailor-made simulation and navigation program using a 3-D printer model of kidney transplantation surgery. Transplant. Proc.47, 596–599 (2015).
- BruyèreF, LerouxC, BrunereauL, LermusiauxP. Rapid prototyping model for percutaneous nephrolithotomy training. J. Endourol.22, 91–96 (2008).
- AdamsF, QiuT, MarkAet al.Soft 3D-printed phantom of the human kidney with collecting system. Ann. Biomed. Eng.45, 963–972 (2017).
- GolabA, SmektalaT, KaczmarekK, StamirowskiR, HrabM, SlojewskiM. Laparoscopic partial nephrectomy supported by training involving personalized silicone replica poured in three-dimensional printed casting mold. J. Laparoendosc Adv. Surg. Tech.27, 420–422 (2017).
- MondaSM, WeeseJR, AndersonBGet al.Development and validity of a silicone renal tumor model for robotic partial nephrectomy training. Urology114, 114–120 (2018).
- TurneyBW. A new model with an anatomically accurate human renal collecting system for training in fluoroscopy-guided percutaneous nephrolithotomy access. J. Endourol.28, 360–363 (2014).
- CheungCL, LooiT, LendvayTS, DrakeJM, FarhatWA. Use of 3-dimensional printing technology and silicone modeling in surgical simulation: development and face validation in pediatric laparoscopic pyeloplasty. J. Surg. Educ.71, 762–767 (2014).
- Von RundstedtF-C, ScovellJM, AgrawalS, ZaneveldJ, LinkRE. Utility of patient-specific silicone renal models for planning and rehearsal of complex tumour resections prior to robot-assisted laparoscopic partial nephrectomy. BJU Int.119, 598–604 (2017).
- MelnykR, EzzatB, BelfastEet al.Mechanical and functional validation of a perfused, robot-assisted partial nephrectomy simulation platform using a combination of 3D printing and hydrogel casting. World J. Urol.38, 1631–1641 (2020).
- MaddoxMM, FeibusA, LiuJ, WangJ, ThomasR, SilbersteinJL. 3D-printed soft-tissue physical models of renal malignancies for individualized surgical simulation: a feasibility study. J. Robot Surg.12, 27–33 (2018).
- LiP, JiangS, YuY, YangJ, YangZ. Biomaterial characteristics and application of silicone rubber and PVA hydrogels mimicked in organ groups for prostate brachytherapy. J. Mech. Behav. Biomed Mater.49, 220–234 (2015).
- ZeinNN, HanounehIA, BishopPDet al.Three-dimensional print of a liver for preoperative planning in living donor liver transplantation: three-dimensional printing. Liver Transpl.19, 1304–1310 (2013).
- WangP, QueW, ZhangMet al.Application of 3-dimensional printing in pediatric living donor liver transplantation: a single-center experience. Liver Transpl.25, 831–840 (2019).
- IshiiT, FukumitsuK, OgawaE, OkamotoT, UemotoS. Living donor liver transplantation in situs inversus totalis with a patient-specific three-dimensional printed liver model. Pediatr. Transplant.24(3), e13675 (2020).
- KurodaS, KobayashiT, OhdanH. 3D printing model of the intrahepatic vessels for navigation during anatomical resection of hepatocellular carcinoma. Int. J. Surg. Case Rep.41, 219–222 (2017).
- KurodaS, KiharaT, AkitaY, KobayashiT, NikawaH, OhdanH. Simulation and navigation of living donor hepatectomy using a unique three-dimensional printed liver model with soft and transparent parenchyma. Surg. Today50, 307–313 (2020).
- HuberT, HuettlF, TripkeV, BaumgartJ, LangH. Experiences with three-dimensional printing in complex liver surgery. Ann. Surg.273, e26–27 (2021).
- Casas-MurilloC, Zuñiga-RuizA, Lopez-BarronREet al.3D-printed anatomical models of the cystic duct and its variants, a low-cost solution for an in-house built simulator for laparoscopic surgery training. Surg. Radiol. Anat.43, 537–544 (2021).
- HeZ, MongrainR, LessardS, ChayerB, CloutierG, SoulezG. Anthropomorphic and biomechanical mockup for abdominal aortic aneurysm. Med. Eng. Phys.77, 60–68 (2020).
- KwonC-I, ShinY, HongJet al.Production of ERCP training model using a 3D printing technique (with video). BMC Gastroenterol.20, 145 (2020).
- DeJong TL, PluymenLH, Van GerwenDJ, KleinrensinkG-J, DankelmanJ, VanDen Dobbelsteen JJ. PVA matches human liver in needle-tissue interaction. J. Mech. Behav. Biomed. Mater.69, 223–228 (2017).
- WitowskiJ, BudzyńskiA, GrochowskaAet al.Decision-making based on 3D printed models in laparoscopic liver resections with intraoperative ultrasound: a prospective observational study. Eur. Radiol.30, 1306–1312 (2020).
- DuanB, HockadayLA, KangKH, ButcherJT. 3D Bioprinting of heterogeneous aortic valve conduits with alginate/gelatin hydrogels. J. Biomed. Mater. Res. A.101A, 1255–1264 (2013).
- MarkstedtK, MantasA, TournierI, MartínezÁvila H, HäggD, GatenholmP. 3D bioprinting human chondrocytes with nanocellulose–alginate bioink for cartilage tissue engineering applications. Biomacromolecules16, 1489–1496 (2015).
- GaoF, XuZ, LiangQ, LiuB, LiH, WuYet al.Direct 3D printing of high strength biohybrid gradient hydrogel scaffolds for efficient repair of osteochondral defect. Adv. Funct. Mater.28, 1706644 (2018).
- GaoQ, NiuX, ShaoL, ZhouL, LinZ, SunAet al.3D printing of complex GelMA-based scaffolds with nanoclay. Biofabrication11, 035006 (2019).
- PepleyDF, SonntagCC, PrabhuRSet al.Building ultrasound phantoms with modified polyvinyl chloride: a comparison of needle insertion forces and sonographic appearance with commercial and traditional simulation materials. Simul. Healthc.13, 149–153 (2018).
- AoyagiM, HiraguriT. Ultrasound phantom using sodium alginate as a gelling agent: ultrasound phantom using sodium alginate. J. Ultrasound Med.36, 2345–2353 (2017).
- KumarK, AndrewsME, JayashankarV, MishraAK, SureshS. Measurement of viscoelastic properties of polyacrylamide-based tissue-mimicking phantoms for ultrasound elastography applications. IEEE Trans Instrum Meas.59, 1224–1232 (2010).
- HeZ, MongrainR, LessardS, ChayerB, CloutierG, SoulezG. Anthropomorphic and biomechanical mockup for abdominal aortic aneurysm. Med. Eng. Phys.77, 60–68 (2020).
- ElviraL, DuránC, HigutiRTet al.Development and characterization of medical phantoms for ultrasound imaging based on customizable and mouldable polyvinyl alcohol cryogel–based materials and 3-d printing: application to high-frequency cranial ultrasonography in infants. Ultrasound Med. Biol.45, 2226–2241 (2019).
- DeJong TL, PluymenLH, Van GerwenDJ, KleinrensinkG-J, DankelmanJ, VanDen Dobbelsteen JJ. PVA matches human liver in needle-tissue interaction. J. Mech. Behav. Biomed. Mater.69, 223–228 (2017).
- LeibingerA, ForteAE, TanZet al.Soft tissue phantoms for realistic needle insertion: a comparative study. Ann. Biomed. Eng.44, 2442–2452 (2016).
- FarjadSultan S. Simulators for training in ultrasound guided procedures. Med. Ultrason.15, 125–131 (2013).
- AmiriSA, BerckelPV, LaiM, DankelmanJ, HendriksBHW. Tissue-mimicking phantom materials with tunable optical properties suitable for assessment of diffuse reflectance spectroscopy during electrosurgery. Biomed. Opt. Express.13, 2616 (2022).
- AlkatoutI, SchollmeyerT, HawaldarNA, SharmaN, MettlerL. Principles and safety measures of electrosurgery in laparoscopy. JSLS16, 130–139 (2012).
- JohnC. The corono-apically varying ultrasonic velocity in human hard dental tissues. J. Acoust. Soc. Am.116, 545–556 (2004).
- AoyagiM. Sodium Alginate ultrasound phantom for medical education. Ultrason. Imaging43, 253–261 (2021).
- EarleM, PortuGD, DeVosE. Agar ultrasound phantoms for low-cost training without refrigeration. Afr. J. Emerg. Med.6, 18–23 (2016).
- AlvesN, KimA, TanJet al.Cardiac tissue-mimicking ballistic gel phantom for ultrasound imaging in clinical and research applications. Ultrasound Med. Biol.46, 2057–2069 (2020).
- LiJW, KarmakarMK, LiX, KwokWH, KeeWDN. Gelatin-agar lumbosacral spine phantom: a simple model for learning the basic skills required to perform real-time sonographically guided central neuraxial blocks. J. Ultrasound Med.30, 263–272 (2011).
- CabrelliLC, GrilloFW, SampaioDRT, CarneiroAAO, PavanTZ. Acoustic and elastic properties of glycerol in oil-based gel phantoms. Ultrasound Med. Biol.43, 2086–2094 (2017).
- HockingG, HebardS, MitchellCH. A review of the benefits and pitfalls of phantoms in ultrasound-guided regional anesthesia. Reg. Anesth. Pain Med.36, 162–170 (2011).
- ChaoS-L, ChenK-C, LinL-W, WangT-L, ChongC-F. Ultrasound phantoms made of gelatin covered with hydrocolloid skin dressing. J. Emerg. Med.45, 240–243 (2013).
- WhewellH, BrownC, GokaniVJet al.Variation in training requirements within general surgery: comparison of 23 countries. BJS Open4, 714–723 (2020).
- MassarwehNN, CosgriffN, SlakeyDP. Electrosurgery: history, principles, and current and future uses. J. Am. Coll. Surg.202, 520–530 (2006).
- QiuK, HaghiashtianiG, McAlpineMC. 3D printed organ models for surgical applications. Annu. Rev. Anal. Chem.11, 287–306 (2018).
- UhlJF, SufianovA, RuizC, IakimovYet al.The use of 3D printed models for surgical simulation of cranioplasty in craniosynostosis as training and education. Brain Sci.13, 894 (2023).