Abstract
Background: The aim of this study is preclinical evaluation of our newly developed regional hyperthermia system providing 3-D SAR control: the AMC-8 phased array consisting of two rings, each with four 70 MHz waveguides. It was designed to achieve higher tumour temperatures and improve the clinical effectiveness of locoregional hyperthermia.
Methods: The performance of the AMC-8 system was evaluated with simulations and measurements aiming at heating a centrally located target region in rectangular (30 × 30 × 110 cm) and elliptical (36 × 24 × 80 cm) homogeneous tissue equivalent phantoms. Three properties were evaluated and compared to its predecessor, the 2-D AMC-4 single ring four waveguide array: (1) spatial control and (2) size of the SAR focus, (3) the ratio between maximum SAR outside the target region and SAR in the focus. Distance and phase difference between the two rings were varied.
Results: (1) Phase steering provides 3-D SAR control for the AMC-8 system. (2) The SAR focus is more elongated compared to the AMC-4 system, yielding a lower SAR level in the focus when using the same total power. This is counter-balanced by (3) a superficial SAR deposition which is half of that in the AMC-4 system, yielding a more favourable ratio between normal tissue and target SAR and allowing higher total power and up to 30% more SAR in the focus for 3 cm ring distance.
Conclusion: The AMC-8 system is capable of 3-D SAR control and its SAR distribution is more favourable than for the 2-D AMC-4 system. This result promises improvement in clinical tumour temperatures.
Introduction
Hyperthermia, raising the tumour temperature to 40–43 °C, leads to radiosensitisation and chemosensitisation in tumour cells Citation[1–9]. Excellent clinical results have been demonstrated in randomised trials comparing radiotherapy and hyperthermia with radiotherapy alone for head and neck cancer, melanoma, rectal cancer, breast and cervical carcinoma Citation[10–15]. Radiosensitisation increases strongly with temperature Citation[16] and clinical data show a strong correlation between clinical results and thermal dose, represented by the minimum tumour temperature T90 or by the equivalent number of minutes at 43 °C Citation[17–30]. Higher tumour temperatures are therefore expected to have a major impact on treatment outcome.
Locoregional hyperthermia of deep-seated tumours is generally performed with phased array systems operating at 70–140 MHz. Good clinical results are achieved but the prescribed thermal dose is not always reached Citation[11], Citation[12], Citation[14], Citation[21], Citation[47–49]. This is often due to the incidence of treatment-limiting hot spots in normal tissue. These are difficult to avoid due to the limited power control abilities of 2-D controlled hyperthermia systems consisting of a ring of antennas around the patient. To overcome this problem new systems have been developed with 3-D power control, capable of maximising the power deposition in the tumour while reducing the temperature rise at potential hot spot locations. This can be achieved by increasing the number of antenna rings, the number of antennas per ring and the operating frequency. Examples of 3-D system designs with multiple rings and a large number of antennas are the RHOCS system (18 antennas) Citation[36] and the 3-D BSD-2000 Sigma-Eye (24 antennas) Citation[37], Citation[38]. Increasing the frequency up to 150–200 MHz yields higher resolution 3-D control, but at the cost of a smaller penetration depth, and thus lower SAR in the tumour.
For many years our department has treated pelvic and oesophageal tumours with the AMC-4 regional hyperthermia system consisting of a matched phased array of four 70 MHz waveguides positioned in a ring around the patient Citation[39], Citation[40]. The heating focus created in the tumour by constructive interference between the waveguides was validated using a LED matrix phantom Citation[41], Citation[42]: the heating pattern is very similar to other regional hyperthermia devices such as the BSD-2000 and the coaxial TEM applicator Citation[43], Citation[44].
The 2-D controlled AMC-4 system proved to be an effective device for regional hyperthermia, albeit that the prescribed thermal dose was not always reached. To increase the thermal dose a new regional hyperthermia device was designed, the AMC-8 system, consisting of two rings of four waveguides per ring to provide 3-D power control, again using a 70 MHz operating frequency. The AMC-8 system is thus a 3-D extension of a well proven, easy-to-control heating device, and expected to provide even better spatial power and temperature control. Moreover, the incidence of treatment limiting superficial hot spots will be intrinsically reduced as system power is distributed over a much larger body surface than for the AMC-4 system. In this paper this ability to reduce hot spots and the 3-D power control of the new AMC-8 system are evaluated and compared to its predecessor.
Materials and methods
AMC-8 system
The AMC-8 system ( and ) consists of two rings of four ¼λ 70 MHz waveguides with an aperture of 34 × 21 cm; total dimension is 38 × 25 cm including a 2 cm flange. The length is 12 cm (¼λ), compared to 48 cm (λ) long waveguides in the AMC-4 system. These waveguides are connected to a DDS-based phase- and amplitude-controlled RF generator (SSB Electronic, Iserlohn, Germany). The final, analogous amplifiers each provide 500 W maximum output power (Henry Radio, Los Angeles, CA). This phase and amplitude locked loop system has a phase accuracy of ±3° and a power accuracy of ±10 W Citation[45]. The waveguides operate in the TE10 mode with the dominant E-field component Ez along the longitudinal patient axis. Provided the E-field of each waveguide is at equal phase at the tumour the Ez(i) contributions of each waveguide i add up, creating a focus in the tumour, where the specific absorption rate (SAR) expressed in W/kg is proportional to the square of the total E-field:
A demi water-filled bolus is positioned between each waveguide and the patient skin or phantom to improve electric coupling without absorbing power. The water circulating in the bolus is kept at 12 °C to provide skin cooling. Each ring contains a left, top and right waveguide, and can be moved over two parallel rails. The distance Dring between the two rings can be set at values starting at 2 cm up to 50 cm. The two bottom waveguides are integrated in the patient table with a mutual distance between 2 and 9 cm, measured between the flanges (). Optional use of just one ring of four waveguides, yielding a system similar to the AMC-4 system, was incorporated to facilitate treatment of tumours in the thoracic region, e.g. oesophageal tumours, where the patients’ arms preclude the use of the full set of eight waveguides.
Figure 2. Transversal cross-section of the AMC-4 system/single ring set up of AMC-8 system (A) combined with the R-phantom, the sagittal midplane is indicated with a dotted line. Sagittal cross sections of the AMC-4 system (B) and the AMC-8 system (C, D) with the transversal midplane indicated with a dotted line. The 24 × 24 cm section where E-field measurements were performed shown in is indicated in the sagittal midplane of the R-phantom in (D). For simplicity, all images show AMC-8 ¼λ waveguides (34 × 21 × 12 cm).
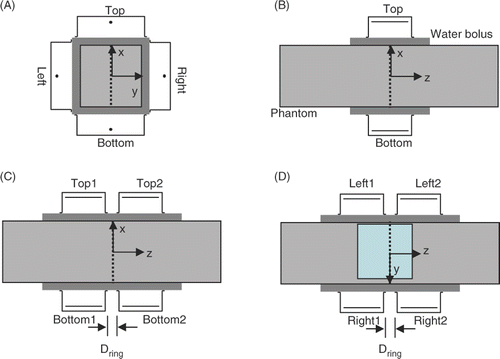
The clinical phase optimisation procedure involves obtaining maximised tumour SAR by phase optimisation of seven pairs of antennas with an E-field probe at the tumour at the start of treatment, similar to the procedure used for the AMC-4 system. This procedure is fast and simple, even for eight waveguides.
The control computer is in the treatment room to facilitate interaction with the patient, at an adequate distance to the system to avoid hazards associated with E-field exposure for the staff. The generator system is located in an adjacent room. Fibre optical measurement and control connections are used to prevent disturbance by the 70 MHz EM field.
Performance tests
The performance of the AMC-8 system has been evaluated in a number of standard configurations. The AMC-4 system, the 2-D controlled predecessor, served as reference.
System properties
To facilitate evaluation the heating patterns were characterised by three properties listed in : focus size, SAR ratio and 3-D control.
Table I. List of relevant system properties with the performance parameters and phantoms used for evaluating the properties.
Size of the SAR focus was quantified by the radial and axial full width half maximum (FWHM) of the SAR focus, the width where SAR is 50% of maximum SAR in the focus. The definition of radial FWHM was adapted for the AMC-4 where 50% SAR was not achieved along the radial axis (). In this case the maximum gradient was extrapolated to obtain an estimate of the radial FWHM.
Figure 3. Simulations showing the impact of ring distance on the normalised SAR distribution in the sagittal midplane of the R-phantom. Left: AMC-4 system (configuration 1). Right: AMC-8 system (configuration 2) with Dring = 5 (top) and Dring = 11 cm (bottom). SAR normalised to maximum SAR in the focus in each panel, note that the SAR level in the focus is lower for 8 than for 4 waveguides (see text). 50% iso SAR shown in bold.
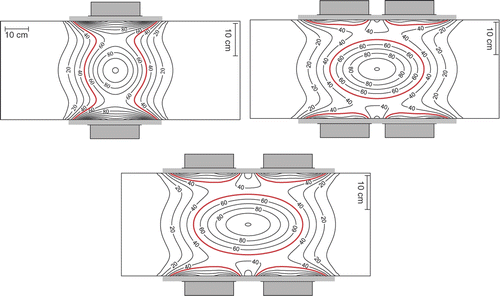
Ratio between maximum SAR in normal tissue and the SAR in the focus was defined as Sratio = SARmaximum in normal tissue/SARtarget. Sratio was introduced to quantify the severity of SAR hot spots and is a measure of the ability to heat the tumour selectively Citation[46]. The change in Sratio from AMC-4 to AMC-8 was evaluated using the relative Sratio:
3-D spatial control was evaluated by studying the position of the SAR focus for different phase settings of the AMC-8 system. We concentrated on focus displacement in the axial direction Δz as this feature of the AMC-8 system is new compared to the AMC-4.
Test configurations
FWHM, Sratio and focus shift Δz were used to evaluate the performance of the AMC-4 and AMC-8 system with simulations and measurements of E-field and SAR distributions in a rectangular homogeneous tissue equivalent R-phantom (30 × 30 × 110 cm3) for three sets of standard configurations summarised in . A standard configuration of the AMC-4 system provides reference values for FWHM and Sratio:
Central SAR focus at (x, y, z) = (0, 0, 0).
These are compared with FWHM and Sratio for a set of AMC-8 configurations with variable ring distances Dring:
Central SAR focus at (x, y, z) = (0, 0, 0), ring distance Dring varied between 2 and 13 cm resulting in different focus sizes. No phase difference between ring 1 and ring 2;
and Δz is investigated for a set of AMC-8 configurations with different phase differences φring between ring 1 and ring 2:
Shifting SAR focus to (x, y, z) = (0, 0, zfocus). phase difference φring = −30°, 0° and 30° resulting in an axial focus shift Δz. Dring = 3 cm.
Advantage of a square cross-section of the R-phantom is that the central focus at z = 0 (located at the aperture midplane for the single ring AMC-4 system and in the midplane between the two waveguide rings for the double ring AMC-8 system) for configurations 1 and 2 is created by equal phase and amplitude for all waveguides for both the AMC-4 and AMC-8 system. The simulated range for Dring is larger than allowed by the table (2−9 cm).
Efficiency
In addition, the system efficiency EFF was determined, defined as the ratio between measured and simulated SAR values for a nominal output power of 800 W and standard phase and amplitude settings. The efficiency of the AMC-4 system EFF(AMC-4) was compared with EFF(AMC-8)1 ring of a single ring of the AMC-8 system, and EFF(AMC-8)1 ring was compared to EFF(AMC-8)2 rings of the double ring configuration of the AMC-8 system using an elliptical tissue equivalent phantom with standard quality assurance dimensions of 36 × 24 × 80 cm3 Citation[43], Citation[44]. The former comparison was performed as the original 48 cm long waveguides in the AMC-4 system were replaced by 12 cm long ¼λ waveguide in the AMC-8 system. These waveguides are equivalent, but there may be differences in efficiency. To this end the SAR distribution in the transversal midplane of the E-phantom was computed and measured for 5 different phase and amplitude settings for each system configuration listed in . Settings 2, 3 and 5 with φring = 0° and phases 30° and 45° for the left and right waveguides yield a central focus in the origin (0,0,0) in the transversal midplane z = 0 in both AMC-4 and AMC-8 system. Settings 1 and 7 with phase 0° for all waveguides yield a blurred focus. Settings 4 and 6 with φring = −30° yield an axially shifted focus for the AMC-8 system. Dring = 3 cm.
Table II. Configurations used for determining characterisation parameters FWHM, RSratio and Δz for the single ring or two rings set up of the AMC-8 system by using E-field distribution measurements and simulations in the R-phantom. FWHM indicates the size of the focus. RSratio is the normalised ratio between maximum normal tissue SAR and SAR in the focus. The SAR focus shift Δz is a measure for 3-D spatial control. Power settings of every ring are listed as the amplitude ratio between the top, bottom, left, right waveguide (t:b:l:r), respectively, with a total power of 800 W. Phase settings are listed for the top, bottom, left, right waveguide (t,b,l,r), respectively. Phase and amplitude settings of ring 2 of the AMC-8 (two rings) are identical to settings of ring 1, with the phase settings offset with φring and a ring to ring distance Dring. †Simulations only.
Table III. Phase and amplitude settings used for determining system efficiency EFF using SAR distribution measurements and simulations in the E-phantom. Five phase and amplitude settings were used for each of the three systems: AMC-4, AMC-8 (single ring) and AMC-8 (two rings). Power settings of every ring are listed as the amplitude ratio between the top, bottom, left, right waveguide (t:b:l:r), respectively, with a total power of 800 W. Phase settings are listed for the top, bottom, left, right waveguide (t,b,l,r), respectively. Phase and amplitude settings of ring 2 of the AMC-8 (two rings) are identical to the settings of ring 1, with the phase settings offset with φring and a ring to ring distance Dring = 3 cm.
Simulations
Computer simulations were performed with our hyperthermia treatment planning (HTP) system based on the finite difference time domain (FDTD) method Citation[47–49]. The AMC-4 and AMC-8 system (), consisting of four or eight ¼λ waveguides (each 34 × 21 × 12 cm3, excluding a modelled 1-cm flange) and four or eight water boluses (40 × 5 × 40 cm3), respectively, were modelled in a discrete space of 151 × 151 × 151 cm3 and at a resolution of 1 × 1 × 1 cm3. The longer λ waveguide (34 × 21 × 48 cm3) was not modelled to save space. The water boluses of pairs of aligned waveguides in the AMC-8 system were modelled as a single continuous bolus. The sources of the emanating E-field were located at the edges of the waveguide chokes and were excited by means of a triple-cosine waveform operating at 70 MHz in the temporal domain. Simulations of configuration sets 1, 2 and 3 () were performed for the AMC-4 and for the AMC-8 system.
Simulations for the efficiency evaluation were performed for the AMC-4 and for the AMC-8 system for the settings listed in .
Measurements
E-field and SAR distributions were measured in tissue equivalent phantoms using either one ring of the AMC-8 system (representing the AMC-4 system), or both rings of the AMC-8 system as indicated in configuration sets 1 and 3, respectively. When one ring was used the second ring was moved away and the second bottom waveguide was removed from the patient table. Configuration set 2 was dropped from the measurements as it was impossible to maintain a continuous bolus for large ring distances Dring.
The E-field distribution was measured in the R-phantom, a liquid rectangular tissue-equivalent phantom (30 × 30 × 110 cm3) filled with a saline solution of 0.3% NaCl. This solution has a relative dielectric permittivity of 77 and a conductivity of 0.51 Sm−1 at room temperature at 70 MHz, properties representative of the tissue in the human torso at 70 MHz Citation[50]. Amplitude and phase of the E-field distribution in the R-phantom are mapped in the transversal and sagittal midplane of the R-phantom with a direction sensitive probe developed at our department in collaboration with TNO-FEL Citation[44], Citation[51]. Measurements were limited to the dominant axial component Ez of the E-field. In each plane horizontal and vertical scans were made with 4 cm spacing between scan lines and 4 E-field measurements per mm. The E-field distributions were then reconstructed in sections of 24 × 24 cm (example shown in ).
To determine efficiency EFF the SAR distribution was measured in the transversal midplane of the E-phantom, a solid phantom with an elliptical cross-section of 36 × 24 cm2 and a length of 80 cm filled with a wall paper paste-like muscle equivalent material (NaCl 3.0 g/L, methylcellulose 26.79 g/L), for the seven different phase and amplitude settings listed in . The SAR values were obtained from ΔT measurements, using the temperature rise ΔT after a power on period of duration Δt:with ct the heat capacity of the E-phantom material. A prepulse technique (prepulse 6 s power on, 5 s power off, main pulse Δt = 60 s 800 W power on) was used to neutralise effects of self heating, if present, similar to the multi pulse technique described by de Leeuw et al. Citation[52]. The temperature rise was measured in 126 points covering the transversal midplane of the E-phantom at z = 0 with 16 multisensor thermocouple probes inserted in polyethylene catheters. The thermocouple probes used have seven or fourteen measurement points with 0.5, 1 or 2 cm spacing (Ella CS, Czech republic) and are monitored using a 196-channel thermocouple thermometry system with an accuracy better than 0.01°C. The efficiency determination was performed for all 126 points in the transversal midplane and for a subset with points within a 10-cm radius from (0, 0, 0) representing the target area.
Results
Size of the central SAR focus
Simulation results in show the SAR distribution in the sagittal midplane of an R-phantom heated by the AMC-4 system or the AMC-8 system, with two different ring distances and normalised at the centre. Phase and amplitude are equal for all waveguides, yielding a central SAR focus (configuration sets 1 and 2). Note the elongated SAR focus in axial direction for the AMC-8 system, particularly for the largest ring distance Dring between the two waveguide rings. The radial and axial FWHM are plotted as a function of ring distance Dring in . The radial FWHM is slightly smaller for the AMC-8 system compared to the AMC-4 system. The axial FWHM is 50% longer than for the AMC-4 system for the smallest ring distance, and 100% longer for the largest ring distance Dring. At the same total power the SAR level in the focus is lower for eight than for four waveguides, this difference can be attributed to the difference in target location. The target is located in front of each waveguide in the single ring system, whereas it is ≥13 cm off centre for each waveguide in the double ring system. The average waveguide target distance thus increases from 20 to 24 cm, effectively resulting in lower target SAR.
Ratio between SAR in focus and in normal tissue
Maximum normal tissue SAR is found at the surface of the tissue equivalent phantom in , and is significantly higher for the AMC-4 system than for the AMC-8 system relative to the SAR in the target. The impact of this difference in superficial SAR on RSratio () shows that using two rings of four waveguides improves Sratio with 30% for small distances Dring compared to a single ring of four (the AMC-4 system). This gain decreases to 15% for larger ring distances Dring, which is still better than for the AMC-4 system.
3-D control of the SAR focus
Measured and simulated Ez distributions in the sagittal midplane of the R-phantom for three different ring to ring phase settings φring = −30°, 0° and 30° for the AMC-8 system (configuration set 3) are shown in . Axial SAR control is excellent: the phase difference φring causes an axial focus shift Δz. The maximum possible range of this shift is thus from the centre of ring 1 to the centre of ring 2, i.e. 25 cm + Dring. In the maximum shift positions the simulated axial FWHM of the focus becomes smaller and similar to the FWHM in the single ring system. The shift of the SAR focus can be quantified using the Ez-profile along the central z-axis for φring = −30°, 0° and 30° in . Fitting the resulting axial Ez-field profiles with a Gaussian function:yielded zfocus equal to 6.7, 1.6 and −2.4 cm for φring = −30°, 0° and 30°, respectively. Hence, a 60° phase difference corresponds to a 9.1 cm focus displacement Δz. The effective phase range for correcting positioning errors <5 cm is thus −30° < φring < 30°.
Figure 6. Ez field distributions in the 24 × 24 cm section of the sagittal midplane of the R-phantom in the AMC-8 system shown in for configuration 3. Top row: measured Ez field distributions for φring = 30° (left hand panel), φring = 0° (central panel) and φring = −30° (right hand panel) reconstructed from data on horizontal and vertical tracks shown. The measured focus position zfocus = 6.7 cm for φring = −30°, zfocus = 1.6 cm for φring = 0°, and zfocus = −2.4 cm for φring = 30°. Bottom row: simulated Ez field distributions matching distributions of the top row in arbitrary units, therefore only position and extension of simulated and measured contours may be compared.
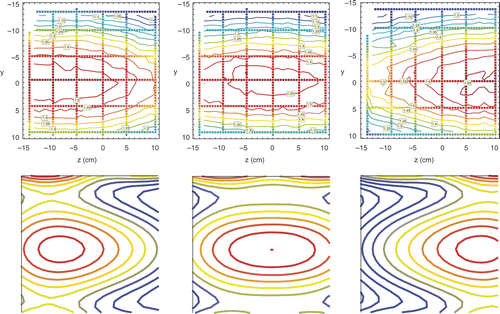
Efficiency
Efficiency was derived from the ratio between measured and simulated SAR in the solid E-phantom. In an example is shown of a measured and a simulated SAR distribution in the E-phantom, both after a 60-s 800-W power pulse by a single ring of the AMC-8 system with phase and amplitude setting 3 from . A simulated efficiency of 64% resulted in an average SAR equal to the measured SAR in the central area (r < 10). Efficiency measurements yielded no significant difference between the different phase and amplitude settings listed in and resulted in a mean efficiency EFF(AMC-4) of 55 ± 9% for the AMC-4 system, versus EFF(AMC-8)1 ring = 68 ± 9% for a single ring in the AMC-8 system for the power deposition in the central target area (r < 10). The difference between EFF(AMC-8)1 ring = 68 ± 9% and EFF(AMC-8)2 rings = 62 ± 9% is not significant.
Figure 7. Heating efficiency determined by comparing measured and simulated SAR distributions in the transversal midplane of the E-phantom. Example shown for a single ring of the AMC-8 system for phase and amplitude setting 3 listed in (amplitude 3 : 3 : 2 : 2, phase 0°,0°,45°,45° for top:bottom:left:right). Left: measured SAR distribution in W/kg. Right: simulated SAR distribution in W/kg, an efficiency of 64% was assumed to match the average simulated SAR to the measured SAR data in the central area (r < 10).
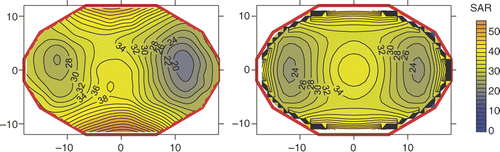
Discussion
The 3-D controlled AMC-8 system was developed to improve treatment delivery. This paper established the properties of the AMC-8 system in comparison to the 2-D controlled AMC-4 system. Three properties relevant for achieving an adequate temperature rise in the target were evaluated:
The 3-D control of the AMC-8 system will result in more accurate targeting and thus in a higher tumour temperature than for the AMC-4 system.
The size of the SAR focus in axial direction is larger for the AMC-8 system than for the AMC-4 system. Thus the SAR in the target is lower than for the AMC-4 when using the same total power.
The heated surface is twice as large compared to the AMC-4 system, thus the superficial power deposition is twice as low when using the same total power compared to the AMC-4 system, expected to lead to less superficial hot spots. This enables the use of more power, presumably resulting in higher tumour temperatures than using the AMC-4 system.
The tumour SAR gain due to 3-D control is not easy to quantify as this depends on the average localisation error in the axial direction when using the 2-D AMC-4 system. This requires a study with realistic patient conditions, in the present phantom set up such errors are likely to be small.
Property 2 is favourable for the AMC-4 system, property 3 for the AMC-8 system. The balance between 2 and 3 is favourable for the AMC-8 system as shown by the 30% improvement in Sratio, equivalent to a 30% rise in maximum achievable tumour SAR and leading to a rise in tumour temperature. The amount of power needed to achieve this gain will be significantly higher than the amount of power generally applied using the AMC-4 system, due to property 2. The distance between the two rings must be kept small for a maximum gain in Sratio and tumour temperature.
There was a small difference in efficiency between the AMC-4 and AMC-8 system, which may be attributed to a small phase deviation for the bottom waveguide in the AMC-4 system. Phase settings 2, 3 and 4 therefore yielded a focus shift, explaining the lower efficiency for r < 10 compared to the entire plane for the AMC-4 system: the energy was absorbed in the plane but with the focus not exactly at the centre. For the AMC-8 system such differences did not occur. Taking this phase deviation into account the difference in efficiency reduces to 7% at most.
Thus the AMC-8 system is capable of 3-D control and improves the heating of centrally located targets in homogeneous phantoms. The next step is to demonstrate the clinical benefit of the AMC-8 over the AMC-4 system regarding improvement in hot spot suppression and SAR control in heterogeneous patient anatomies.
For the homogeneous phantoms used in this study the ‘normal tissue hot spots’ occur near the outer surface, with a maximum SAR lower than the SAR in the focus. Likely hot-spot locations in realistic anatomies are at superficial fat-muscle interfaces, probably reaching higher SAR levels than in homogeneous phantoms. The resulting temperature rise can be suppressed using skin cooling using the water bolus so that these are not treatment limiting and acceptable tumour temperatures achievable. Hot spots may also occur at muscle-fat interfaces at greater depth and closer to the tumour. Hot spots at greater depth are insensitive for skin cooling but can be neutralised by SAR steering. Hot spots near the tumour are difficult to suppress as even SAR steering may not be able to maintain high tumour temperatures and suppress nearby hot spots at the same time Citation[53]. Thus the Sratio is expected to be less favourable in realistic anatomies than in the homogeneous tissue used in this study, but the advantage of the AMC-8 system over the AMC-4 system is likely to hold.
Simulation studies of the performance of other regional hyperthermia devices showed significant improvement with 3-D control in realistic anatomies. Upgrading the BSD sigma 60 applicator from one ring of eight antennas to three rings with 24 antennas was found to yield a potential temperature gain of 1.7–2°C for two examples of patients with rectal cancer in a study by Wust et al. Citation[54], a gain equivalent to quadrupling thermal dose. Seebass et al. Citation[55] extended this study and found a BSD system with three rings, six to eight channels per ring operating at ∼150 MHz yields the best results in a varied group of five tumour locations. Paulsen et al. Citation[56] studied the potential temperature gain from the introduction of 3-D power control for a hypothetical system comparing heatability of ten superficial and three deep target locations while varying three parameters: number of rings (1, 2 and 4), number of antennas per ring (2–4, 6, 8, 12 and 24), and operating frequency (100, 150 or 200 MHz). Results showed all three parameters influenced temperature gain. Frequencies > 100 MHz were advantageous, especially for more superficial target locations. Both an increase in the circumferential antenna density and in the number of rings improved the gain. Four rings yielded the best suppression of hot spots outside the target volume. The impact of operating frequency and number and configuration of antennas was studied by Kroeze et al. Citation[36] for the design of an open water bolus cavity slot applicator (RHOCS) for three different patient examples. A system operating at 150 MHz with three rings and six antennas per ring yielded the best gain in power deposition in the tumour.
In comparison to these systems the active hot spot suppression of the AMC-8 system is expected to be less effective due to its comparatively low frequency and low number of antennas. This is compensated by the lower superficial SAR deposition, good penetration depth and the robust optimisation procedure of the AMC-8 system. The theoretical tumour temperature gain is excellent for the three systems but obtained using a simulated anatomy. Clinically correct optimal settings are not easily obtained for systems with high numbers of independent antennas, and are also likely to become less accurate for increasing numbers of antennas. The optimisation tools clinically used Citation[57] are reliable but the inevitable small errors in input data reduce the reliability of pre-treatment computed optimised settings. More accurate settings can be obtained by optimisation during treatment; this requires fast on-line optimisation for which methods are currently being developed Citation[58–61]. The robust clinical optimisation procedure used for the AMC-8 system is feasible for eight waveguides, but time-consuming and presently rarely applied in 18 or 24 antenna systems. The clinical gain obtained with the AMC-8 system is presently evaluated in a study supported by the Dutch Cancer Society.
Conclusion
The AMC-8 system is capable of 3-D SAR control and its SAR distribution is more favourable than the SAR distribution of the 2-D controlled AMC-4 system. Clinically this difference should lead to fewer hot spots and higher tumour temperatures compared to the AMC-4 system.
Declaration of interest: The authors report no conflicts of interest. The authors alone are responsible for the content and writing of the article.
References
- Field SB. In vivo aspects of hyperthermic oncology. An introduction to the practical aspects of clinical hyperthermia, SB Field, J Hand. Taylor and Francis, London 1990; 55–68
- Raaphorst G. Fundamental aspects of hyperthermic biology. An introduction to the practical aspects of clinical hyperthermia, SB Field, J Hand. Taylor and Francis, London 1990; 10–54
- Overgaard J, Radacic MM, Grau C. Interaction of hyperthermia and cis-diamminedichloroplatinum(II) alone or combined with radiation in a C3H mammary carcinoma in vivo. Cancer Res 1991; 51: 707–711
- Sminia P, Los G, Hendriks JJ, van Vugt MJ, Haveman J, Gonzalez GD. The influence of hyperthermia on the uptake of cisplatin in the rat cervical spinal cord. Eur J Cancer 1992; 28A: 1139–1143
- Dahl O. Interaction of heat and drugs in vitro and in vivo 1995; 103–121
- Hettinga JV, Konings AW, Kampinga HH. Reduction of cellular cisplatin resistance by hyperthermia–A review. Int J Hyperthermia 1997; 13: 439–457
- Rietbroek RC, van de Vaart PJ, Haveman J, Blommaert FA, Geerdink A, Bakker PJ, Veenhof CH. Hyperthermia enhances the cytotoxicity and platinum-DNA adduct formation of lobaplatin and oxaliplatin in cultured SW 1573 cells. J Cancer Res Clin Oncol 1997; 123: 6–12
- Westermann AM, Jones EL, Schem B-C, van der Steen-Banasik EM, Koper P, Mella O, Uitterhoeve AL, de Wit R, van der Velden J, Burger C, et al. First results of triple-modality treatment combining radiotherapy, chemotherapy, and hyperthermia for the treatment of patients with stage IIB, III, and IVA cervical carcinoma. Cancer 2005; 104: 763–770
- Romano M, Maluta S, Cordiano C, Delaini GG, Genna M, Oliani C, Capelli P, Tomezzolli A, Manfrini C, Porcaro AB, et al. Pre-operative radio-chemotherapy plus regional hyperthermia in the treatment of advanced rectal cancer: Experience with radiation dose escalation. Int J Radiat Oncol Biol Phys 2003; 57: S386–S387
- Datta NR, Bose AK, Kapoor HK, Gupta S. Head and neck cancers: Results of thermoradiotherapy versus radiotherapy. Int J Hyperthermia 1990; 6: 479–486
- Overgaard J, Gonzalez GD, Hulshof MC, Arcangeli G, Dahl O, Mella O, Bentzen SM. European Society for Hyperthermic Oncology. Randomised trial of hyperthermia as adjuvant to radiotherapy for recurrent or metastatic malignant melanoma. Lancet 1995; 345: 540–543
- Vernon CC, Hand JW, Field SB, Machin D, Whaley JB, van der Zee J, van Putten WL, van Rhoon GC, van Dijk JD, González GD, et al. International Collaborative Hyperthermia Group. Radiotherapy with or without hyperthermia in the treatment of superficial localized breast cancer: Results from five randomized controlled trials. Int J Radiat Oncol Biol Phys 1996; 35: 731–744
- Rau B, Wust P, Hohenberger P, Loffel J, Hunerbein M, Below C, Gellermann J, Speidel A, Vogl T, Riess H, et al. Preoperative hyperthermia combined with radiochemotherapy in locally advanced rectal cancer: A phase II clinical trial. Ann Surg 1998; 227: 380–389
- van der Zee J, Gonzalez GD, van Rhoon GC, van Dijk JD, van Putten WL, Hart AA. Dutch Deep Hyperthermia Group. Comparison of radiotherapy alone with radiotherapy plus hyperthermia in locally advanced pelvic tumours: A prospective, randomised, multicentre trial. Lancet 2000; 355: 1119–1125
- van der Zee J, Gonzalez GD. The Dutch Deep Hyperthermia Trial: Results in cervical cancer. Int J Hyperthermia 2002; 18: 1–12
- Field SB, Morris CC. The relationship between heating time and temperature: Its relevance to clinical hyperthermia. Radiother Oncol 1983; 1: 179–186
- Dewhirst MW, Sim DA, Sapareto S, Connor WG. Importance of minimum tumor temperature in determining early and long-term responses of spontaneous canine and feline tumors to heat and radiation. Cancer Res 1984; 44: 43–50
- Shimm DS, Kittelson JM, Oleson JR, Aristizabal SA, Barlow LC, Cetas TC. Interstitial thermoradiotherapy: Thermal dosimetry and clinical results. Int J Radiat Oncol Biol Phys 1990; 18: 383–387
- Cox RS, Kapp DS. Correlation of thermal parameters with outcome in combined radiation therapy-hyperthermia trials. Int J Hyperthermia 1992; 8: 719–732
- Sneed PK, Gutin PH, Stauffer PR, Phillips TL, Prados MD, Weaver KA, Suen S, Lamb SA, Ham B, Ahn DK, et al. Thermoradiotherapy of recurrent malignant brain tumors. Int J Radiat Oncol Biol Phys 1992; 23: 853–861
- Oleson JR, Samulski TV, Leopold KA, Clegg ST, Dewhirst MW, Dodge RK, George SL. Sensitivity of hyperthermia trial outcomes to temperature and time: Implications for thermal goals of treatment. Int J Radiat Oncol Biol Phys 1993; 25: 289–297
- Kapp DS, Cox RS. Thermal treatment parameters are most predictive of outcome in patients with single tumor nodules per treatment field in recurrent adenocarcinoma of the breast. Int J Radiat Oncol Biol Phys 1995; 33: 887–899
- Sherar M, Liu FF, Pintilie M, Levin W, Hunt J, Hill R, Hand J, Vernon C, van Rhoon G, van der Zee J, et al. Relationship between thermal dose and outcome in thermoradiotherapy treatments for superficial recurrences of breast cancer: Data from a phase III trial. Int J Radiat Oncol Biol Phys 1997; 39: 371–380
- Wust P, Rau B, Gellerman J, Pegios W, Loffel J, Riess H, et al. Radiochemotherapy and hyperthermia in the treatment of rectal cancer. Recent Results Cancer Res 1998; 146: 175–191
- Dewhirst M. Thermal dosimetry. Thermo-radiotherapy and thermochemotherapy, MH Seegenschmiedt, P Fessenden, C Vernon. Springer, Berlin 1995; 123–136
- Leopold KA, Dewhirst MW, Samulski TV, Dodge RK, George SL, Blivin JL, Prosnitz LR, Oleson JR. Cumulative minutes with T90 greater than Tempindex is predictive of response of superficial malignancies to hyperthermia and radiation. Int J Radiat Oncol Biol Phys 1993; 25: 841–847
- Overgaard J, Gonzalez GD, Hulshof MC, Arcangeli G, Dahl O, Mella O, Bentzen SM. Hyperthermia as an adjuvant to radiation therapy of recurrent or metastatic malignant melanoma. A multicentre randomized trial by the European Society for Hyperthermic Oncology. Int J Hyperthermia 1996; 12: 3–20
- Myerson RJ, Perez CA, Emami B, Straube W, Kuske RR, Leybovich L, Von Gerichten D. Tumor control in long-term survivors following superficial hyperthermia. Int J Radiat Oncol Biol Phys 1990; 18: 1123–1129
- Thrall DE, LaRue SM, Yu D, Samulski T, Sanders L, Case B, Rosner G, Azuma C, Poulson J, Pruitt AF, et al. Thermal dose is related to duration of local control in canine sarcomas treated with thermoradiotherapy. Clin Cancer Res 2005; 11: 5206–5214
- Maguire PD, Samulski TV, Prosnitz LR, Jones EL, Rosner GL, Powers B, Layfield LW, Brizel DM, Scully SP, Harrelson JM, et al. A phase II trial testing the thermal dose parameter CEM43° T90 as a predictor of response in soft tissue sarcomas treated with pre-operative thermoradiotherapy. Int J Hyperthermia 2001; 17: 283–290
- Hand JW, Machin D, Vernon CC, Whaley JB. Analysis of thermal parameters obtained during phase III trials of hyperthermia as an adjunct to radiotherapy in the treatment of breast carcinoma. Int J Hyperthermia 1997; 13: 343–364
- Seegenschmiedt MH, Martus P, Fietkau R, Iro H, Brady LW, Sauer R. Multivariate analysis of prognostic parameters using interstitial thermoradiotherapy (IHT-IRT): Tumor and treatment variables predict outcome. Int J Radiat Oncol Biol Phys 1994; 29: 1049–1063
- Prionas SD, Kapp DS, Goffinet DR, Ben-Yosef R, Fessenden P, Bagshaw MA. Thermometry of interstitial hyperthermia given as an adjuvant to brachytherapy for the treatment of carcinoma of the prostate. Int J Radiat Oncol Biol Phys 1994; 28: 151–162
- Wust P, Stahl H, Loffel J, Seebass M, Riess H, Felix R. Clinical, physiological and anatomical determinants for radiofrequency hyperthermia. Int J Hyperthermia 1995; 11: 151–167
- Ryan TP, Trembly BS, Roberts DW, Strohbehn JW, Coughlin CT, Hoopes PJ. Brain hyperthermia: I. Interstitial microwave antenna array techniques–The Dartmouth experience. Int J Radiat Oncol Biol Phys 1994; 29: 1065–1078
- Kroeze H, van de Kamer JB, de Leeuw AA, Lagendijk JJ. Regional hyperthermia applicator design using FDTD modelling. Phys Med Biol 2001; 46: 1919–1935
- Wust P, Beck R, Berger J, Fahling H, Seebass M, Wlodarczyk W, Hoffmann W, Nadobny J. Electric field distributions in a phased-array applicator with 12 channels: Measurements and numerical simulations. Med Phys 2000; 27: 2565–2579
- Nadobny J, Fahling H, Hagmann MJ, Turner PF, Wlodarczyk W, Gellermann JM, Deuflhard P, Wust P. Experimental and numerical investigation of feed-point parameters in a 3-D hyperthermia applicator using different FDTD models of feed networks. IEEE Trans Biomed Eng 2002; 49: 1348–1359
- van Dijk J, Gonzalez DG, Blank LE. Deep local hyperthermia with a four aperture array system of large waveguide applicators. Results of simulations and clinical application. Hyperthermic Oncology. Taylor and Francis, London 1988; 573–575
- van Dijk JD, Schneider C, van Os R, Blank LE, Gonzalez DG. Results of deep body hyperthermia with large waveguide radiators. Adv Exp Med Biol 1990; 267: 315–319
- Schneider C, van Dijk JD. Visualization by a matrix of light-emitting diodes of interference effects from a radiative four-applicator hyperthermia system. Int J Hyperthermia 1991; 7: 355–366
- Schneider CJ, de Leeuw AA, van Dijk JD. Quantitative determination of SAR profiles from photographs of the light-emitting diode matrix. Int J Hyperthermia 1992; 8: 609–619
- Schneider CJ, van Dijk JD, de Leeuw AA, Wust P, Baumhoer W. Quality assurance in various radiative hyperthermia systems applying a phantom with LED matrix. Int J Hyperthermia 1994; 10: 733–747
- Schneider CJ, Kuijer JP, Colussi LC, Schepp CJ, van Dijk JD. Performance evaluation of annular arrays in practice: The measurement of phase and amplitude patterns of radio-frequency deep body applicators. Med Phys 1995; 22: 755–765
- Crezee J, Kok HP, Wiersma J, van Stam G, Sijbrands J, Bel A, van Haaren PM. Improving locoregional hyperthermia equipment using 3-D power control: From AMC-4 to AMC-8. Proceedings 22nd annual meeting of the European Society for Hyperthermic Oncology (Graz) 2005. pp 14–15
- Wiersma J. Pre-implementation simulations to improve the axial control of a waveguide based hyperthermia system. Hyperthermia treatment planning for the AMC-4 system using the WF-CGFFT method. PhD thesis. 2005. pp 111–122
- Van de Kamer JB, De Leeuw AA, Hornsleth SN, Kroeze H, Kotte AN, Lagendijk JJ. Development of a regional hyperthermia treatment planning system. Int J Hyperthermia 2001; 17: 207–220
- van de Kamer JB, van Vulpen M, de Leeuw AA, Kroeze H, Lagendijk JJ. CT-resolution regional hyperthermia treatment planning. Int J Hyperthermia 2002; 18: 104–116
- Kok HP, Van Haaren PM, Van de Kamer JB, Wiersma J, van Dijk JD, Crezee J. High resolution temperature based optimisation for hyperthermia treatment planning. Phys Med Biol 2005; 50: 3127–3141
- Hagmann MJ, Levin RL, Turner PF. A comparison of the annular phased array to helical coil applicators for limb and torso hyperthermia. IEEE Trans Biomed Eng 1985; 32: 916–926
- van Stam G, Wiersma J, van Wieringen N, van Dijk J. Performance tests of an electric field measurement system. Proceedings 18th Annual meeting of the European Society for Hyperthermic Oncology; 1999. p 80
- de Leeuw AA, Crezee J, Lagendijk JJ. Temperature and SAR measurements in deep-body hyperthermia with thermocouple thermometry. Int J Hyperthermia 1993; 9: 685–697
- Wiersma J, van Wieringen N, Crezee H, van Dijk JD. Delineation of potential hot spots for hyperthermia treatment planning optimisation. Int J Hyperthermia 2007; 23: 287–301
- Wust P, Seebass M, Nadobny J, Deuflhard P, Monich G, Felix R. Simulation studies promote technological development of radiofrequency phased array hyperthermia. Int J Hyperthermia 1996; 12: 477–494
- Seebass M, Beck R, Gellermann J, Nadobny J, Wust P. Electromagnetic phased arrays for regional hyperthermia: Optimal frequency and antenna arrangement. Int J Hyperthermia 2001; 17: 321–336
- Paulsen KD, Geimer S, Tang J, Boyse WE. Optimization of pelvic heating rate distributions with electromagnetic phased arrays. Int J Hyperthermia 1999; 15: 157–186
- Wiersma J, van Maarseveen RA, van Dijk JD. A flexible optimization tool for hyperthermia treatments with RF phased array systems. Int J Hyperthermia 2002; 18: 73–85
- Cheng KS, Stakhursky VL, Stauffer P, Dewhirst M, Das SK. Online feedback focusing algorithm for hyperthermia cancer treatment. Int J Hyperthermia 2007; 23: 539–554
- Weihrauch M, Wust P, Weiser M, Nadobny J, Eisenhardt S, Budach V, Gellermann J. Adaptation of antenna profiles for control of MR guided hyperthermia (HT) in a hybrid MR-HT system. Med Phys 2007; 34: 4717–4725
- Cheng KS, Yuan Y, Li Z, Stauffer PR, Maccarini P, Joines WT, Dewhirst MW, Das SK. The performance of a reduced-order adaptive controller when used in multi-antenna hyperthermia treatments with nonlinear temperature-dependent perfusion. Phys Med Biol 2009; 54: 1979–1995
- Stakhursky VL, Arabe O, Cheng KS, Macfall J, Maccarini P, Craciunescu O, Dewhirst M, Stauffer P, Das SK. Real-time MRI-guided hyperthermia treatment using a fast adaptive algorithm. Phys Med Biol 2009; 54: 2131–2145