Abstract
Purpose: The purpose of this study was to evaluate different methods of magnetic resonance thermometry (MRTh) for the monitoring of intradiscal laser ablation therapy in an open 1.0 Tesla magnetic resonance (MR) scanner.
Material and methods: MRTh methods based on the two endogenous MR temperature indicators of spin-lattice relaxation time T1 and water proton resonance frequency (PRF) shift were optimised and compared in vitro. For the latter, we measured the effective spin-spin relaxation times T2* in intervertebral discs of volunteers. Then we compared four gradient echo-based imaging techniques to monitor laser ablations in human disc specimens. Criteria of assessment were outline of anatomic detail, immunity against needle artefacts, signal-to-noise ratio (SNR) and accuracy of the calculated temperature.
Results: T2* decreased in an inverse and almost linear manner with the patients’ age (r = 0.9) from 70 to 30 ms (mean of 49 ms). The optimum image quality (anatomic details, needle artefacts, SNR) and temperature accuracy (±1.09°C for T1-based and ±1.11°C for PRF-based MRTh) was achieved with a non-spoiled gradient-echo sequence with an echo time of TE = 10 ms.
Conclusion: Combination of anatomic and thermometric non-invasive monitoring of laser ablations in the lumbar spine is feasible. The temperature accuracy of the investigated T1- and PRF-based MRTh methods in vitro is high enough and promises to be reliable in vivo as well.
Introduction
High-temperature ablation therapies can be an alternative to traditional surgery Citation[1], Citation[2]. One of these clinical applications, percutaneous laser disc decompression (PLDD), is described for the treatment of symptomatic herniated intervertebral discs. Here, pain reduction is achieved by thermally reducing intradiscal pressure and thus the discs’ volume Citation[3]. The therapeutic success of PLDD, whether under CT/fluoroscopic imaging Citation[4] or MR-guidance Citation[5], is clinically defined by precise needle placement and predefined energy deposition Citation[3], Citation[6]. Moreover, thermally magnetic resonance (MR)-controlled therapy permits localised monitoring of the increasing temperature inside the disc, as well as adverse temperature spreading to vulnerable adjacent anatomic structures.
MR thermometry (MRTh) has been proven to be a reliable method for therapy control Citation[7], Citation[8]. Non-invasive temperature monitoring has been established in the thermal treatment of specific soft tissue organs (brain Citation[9], liver Citation[10], prostate Citation[11]). Clinical feasibility studies of MR-monitored ablation therapies of the skeletal system exist for bone tumours Citation[12] and osteoid osteoma Citation[13]. Thermometric research as well as clinical routine are performed at field strengths ranging from 0.5 T Citation[5] up to 7 T Citation[14] in open Citation[13], Citation[15] and closed bore Citation[10], Citation[11] scanner systems. An overview of the diversity of MR-based temperature calculations is given by Rieke et al. Citation[16]. However, typically used thermometric methods are based on the endogenous temperature indicators, such as spin-lattice relaxation time (T1) Citation[17] and the phase shift of water proton resonance frequency (PRF) Citation[18]. In 1998, Steiner et al. first presented T1-MRTh during PLDD in an open system at 0.5 T Citation[19], but provided no information on temperature accuracy. To our knowledge there are no clinical applications of thermal monitoring during PLDD within an open 1.0 T system. MRTh based on PRF, although proven to be very temperature-sensitive Citation[20], has not yet been investigated for the monitoring of thermal therapies in the spine.
The aim of our study is to systematically compare two MRTh methods (T1, PRF) in phantom experiments with respect to further investigations on real-time feedback techniques. We started with relaxation time studies of the intervertebral disc (T1 and effective spin-spin relaxation time (T2*)). We then compared four gradient echo-based imaging techniques normally used for MR thermometry and examined image quality as well as temperature accuracy for the two described thermometry methods. Further, we parameterised a gradient-recalled sequence in dependence to echo time (TE) and repetition time (TR). Finally, we present colour-coded temperature maps of in vitro ablation.
Methods
Experimental set-up
All experiments were performed in an open 1.0 T MR-scanner with vertical field configuration (Panorama, Philips Healthcare, Best, NL) and a flexible multi-purpose surface coil (). Measurements were performed in accordance to the clinical PLDD set-up in vitro in human disc specimens as well as in vivo in volunteers. All volunteers were asked to take a lateral recumbent position at the scanner's iso-centre in analogy to the patients’ position during a PLDD.
Figure 1. Experimental setup for temperature measurements during laser ablation in human disc specimen. (A) The static main field in the open MRI is vertical (white arrows), the open configuration allows good access from all sides to the scanners' iso-centre. (B) The bare laser fiber (1) and the fiber optic sensor (2) are inserted into the in vitro phantoms. A flexible multi-purpose coil (3) was used for MR-imaging. During heating experiments the dynamically acquired images could be viewed on an in-room monitor (4) next to the scanner. (C) The bare laser fiber and the fiber optic probe are placed orthogonally to one another with a distance of about 4mm inside the disc tissue.
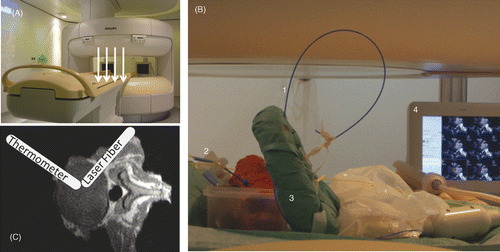
For our in vitro experiments we used freshly excised human intervertebral disc specimens. The discs’ collagen tissue is fat free, contains no vessels and consists of two parts: a viscous centre (nucleus) and a strong, fibrous ligament surrounding the nucleus (annulus).
18G MR compatible pencil tip needles (Somatex, Teltow, Germany) were positioned at an angle of approximately 45° with respect to the main field B0. Spatial and therapeutic considerations of spinal interventions in an open MR-scanner necessitate a puncture angle of (45°) Citation[21] to ensure regular needle artifacts Citation[22] and procedural reliability. A 400 µm end-firing, bare laser fibre connected to a 1064 nm Nd:YAG laser (Medilas Fibertom 5100, Dornier Med Tech, Wessling, Germany) was inserted through the cannula into the centre of the disc. The needle was retracted approximately 5 mm Citation[6], Citation[13] before the laser procedure with single 1-second pulses and intervals was performed. Laser power was 10 W, due to energy loss along the 12-m laser fibre, resulting in 1800–2200 J effectively in the disc material after 10 minutes lasing. In a clinical setting, <1500 J Citation[3] are delivered to the disc. In this experimental setting, these limits were exceeded to ensure a sufficient temperature increase from room temperature up to ≥60°C in immediate proximity of the temperature probe. Direct temperature measurements were performed using a fibre-optic temperature sensor (reFlex, Neoptix, Quebec, Canada) with a tip diameter of 1.15 mm, a manufacturer guaranteed accuracy of ±0.8°C within the temperature range of −80° to 250°C and a response time of 250 ms. The sensor was positioned approximately 4 mm from the end of the laser fibre. Three heating experiments were performed per specimen, while MR magnitude and phase data were dynamically recorded.
Relaxation time studies
To optimise the MRTh sequences for the intradiscal ablation therapy the knowledge of relaxation times T1 and T2* of the anatomic region is of interest. In our measurements we obtained relaxation times of discs in lumbar (L) and sacral (S) spine (L5/S1-L2/L3) from 20 volunteers. T1 was acquired according to Look and Locker Citation[23] by an inversion prepared segmented echo planar imaging technique. Hereby an inversion pulse is followed by a train of equally spaced interrogation pulses (25 ms) with small flip angles (TR/TE/FA = 18 ms/6.2 ms/8°). The spacing in between adjacent inversion pulses was 5 s.
We analysed T2* with a gradient-recalled echo protocol and multiple echoes (TR/FA = 21 ms/25°) with six signal readouts (first TE/echo spacing = 2.1 ms/3.2 ms). The relaxation times were fitted to the corresponding exponential curves on a pixel-by-pixel basis using a non-linear fitting algorithm of Levenberg-Marquardt Citation[24].
Sequence studies
We compared four different gradient echo-based sequences for MRTh in our in vitro experiments: an echo-planar imaging sequence (EPI) with rapid echo-shifting (principles of echo shifting using a train of observations, PRESTO), an EPI without echo shifting, a T1-weighted spoiled gradient echo sequence (T1W-GRE) and an unspoiled gradient echo sequence (GRE). To ensure comparability, field of view (230 * 290 mm2), voxel size (2.8 * 2.8 * 4 mm3) and acquisition time (TA) (8 s) were the same in all four sequences. The sequence protocols were optimised by imaging experiments on volunteers. Herein, the signal was increased by varying the bandwidth (BW) in readout direction, FA, TR and TE as follows:
PRESTO (TR/TE/FA/BW/EPI-factor/slices = 18 ms/23 ms/7°/2061 Hz/5/15),
EPI (TR/TE/FA/BW/EPI-factor/slices = 21 ms/10 ms/20°/894 Hz/11/15),
T1W-GRE (TR/TE/FA/BW/slices = 15 ms/10 ms/20°/2129 Hz/3) and
GRE (TR/TE/FA/BW/slices = 15 ms/10 ms/25°/2129 Hz/3).
The assessment criterion was the signal to noise ratio (SNR) in the imaged lumbar spines of the aforementioned volunteers. All heating experiments were performed in phantoms. In these in vitro experiments, needle artefacts were measured by plotting intensity profiles. The artefact was defined to be the region deviating ± 30% from the median signal intensity around the needle Citation[22]. Temperature sensitivity (T1, PRF) was determined by means of linear regression analysis.
Further, TE and TR were varied in the unspoiled GRE sequence (TR = TE + 3 ms; TE = 2, 7, 10, 12, 15 ms). In this part of the study, heating experiments were performed and temperature sensitivity was assessed (T1, PRF). A linear regression analysis, as well as Bland-Altman analysis was performed.
Temperature calculation
MRTh was calculated from magnitude and phase data using an image processing software (MATLAB, MathWorks, Natick, USA). Calibration constants and dynamic temperature series were derived applying pixel-by-pixel calculation.
We processed T1-MRTh data by calculating the relative temperature increase from magnitude image differences according to the two-parameter model ΔT = a + b · SN, Citation[25]. Constant a is the temperature offset measured by the optical fibre probe before starting the laser procedure. The slope b is defined by the specific tissue type, as well as by the specific sequence parameters, which are flip angle (FA) and repetition time (TR). SN is the normalised signal strength SN = (S0 − S)/S0 Citation[26]. Here, S0 is the signal of the first magnitude image and S is the signal of the current image. SN is averaged over four pixels, taken at three different reference points near the optical fibre from two different heating experiments.
During PRF-MRTh the temperature-induced shift of hydrogen-PRF is extracted from phase image data, as first described by Ishihara et al. Citation[18]. We calculated the relative temperature changes ΔT from phase image differences according to ΔT = Δφ/α · γ · B0 · TE. Here, Δφ is the phase difference between the current image and the reference image acquired before heating. α is the temperature-dependent chemical shift (0.01 ppm/K), γ is the gyromagnetic ratio of hydrogen protons (γ/2π = 42.576 MHz/T), B0 is the static magnetic field (1.0 T) and TE is the echo time used in the sequence. The magnetic field drift was monitored in the surrounding muscle tissue. Drift corrections Citation[27] were averaged over 25 pixels and the dynamic phase image differences were averaged over 4 pixels as described above (T1-MRTh) as is illustrated in .
Figure 2. Representative data of the thermal history show the direct temperature measured with the fiber optic probe and the dynamically acquired MRTh data of the unspoiled GRE (TE = 7ms) during the heating experiment. PRF-MRTh with a postprocessing algorithm (•), that subtracts the magnetic field drift (⋄) from the phase-image differences (*), avoids an underestimation of the temperature.
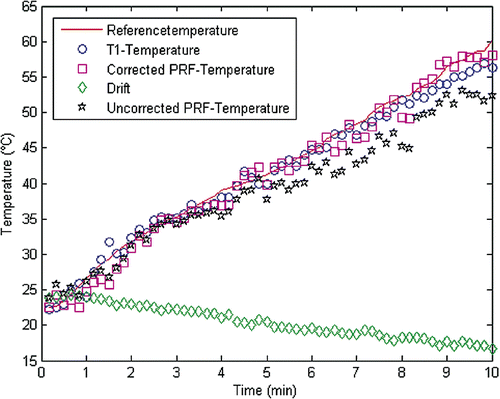
Results
Relaxation times studies
Relaxation parameters in the nucleus averaged 690 ± 70 ms for the T1 and 49 ± 13 ms for T2*. The highest T2* value (72.3 ms) was measured in the disc of a 27-year-old female, while the lowest T2* value was measured in the disc of a 69-year-old female (27.9 ms). A high age-dependency for discal T2* values () was observed (coefficient of correlation r : 0.912). T1 values showed no age-dependency.
Sequence studies
In volunteers, the unspoiled GRE showed the least susceptibility artefacts out of the investigated pulse sequences. The EPI-based sequences showed substantial susceptibility artefacts at the phase margins between the different tissues in the volunteers’ lumbar region (disc, bone, cerebrospinal fluid). The T1W-GRE and the GRE were superior with respect to anatomical detail, such as nerve roots, facet joints and the spinal canal. SNR values for vertebral discs as well as for vertebral endplates were highest in the GRE sequence ().
Figure 4. Comparison of four gradient-echo based sequences for thermal mapping. (A) SNR in two different tissues types acquired during volunteer scans, (B) needle susceptibility artifacts in cadaveric lumbar disc tissue.
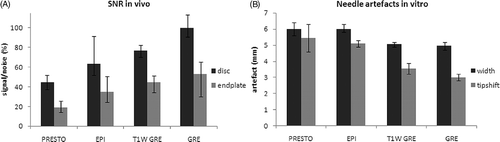
In the phantom studies, the GRE showed the smallest needle artefact (PRESTO/EPI/T1W-GRE/GRE: 6.0/6.0/5.1/4.9 mm) and the smallest tip-shift error (PRESTO/EPI/T1W-GRE/GRE: 5.4/5.1/3.6/3.0 mm) (). The highest correlation between temperatures, directly measured and calculated from PRF-MRTh, was shown for the GRE sequence (PRESTO/EPI/T1W-GRE/GRE: 0.688/0.981/0.899/0.984). The smallest temperature error of PRF-thermometry was shown for the GRE sequence as well (PRESTO/EPI/T1W-GRE/GRE: 4.3 ± 3.6/3.8 ± 2.9/2.3 ± 1.0/1.5 ± 1.5°C).
Comparing the four sequences with respect to temperature sensitivity calculated with T1-MRTh, the GRE-calculated temperatures showed the highest correlation to the measured temperatures (PRESTO/EPI/T1W-GRE/GRE: 0.935/0.986/0.983/0.988) and the smallest temperature error (PRESTO/EPI/T1W-GRE/GRE: 5.3 ± 3.7/1.2 ± 0.9/1.4 ± 0.8/1.1 ± 0.8°C).
Results of T1- and PRF-based MRTh applying GRE with different TEs are shown in . The accuracy of MRTh-derived temperature increases with increasing TE for the PRF-MRTh, which is illustrated by the increasing coefficient of determination and the decreasing temperature error. For the T1-based MRTh, the accuracy of temperature increases in shortening TE. The minimum (0.61°C) and maximum temperature errors (2.46°C) were reached with PRF-MRTh during heating experiments.
Table I. Results of linear regression analysis of the phantom heating experiments for GRE with T1- and PRF-based MRTh. The analysis was based on a two-parameter model ΔTMR=Tref_min+b · TMR, the number of data points was n = 60 and the temperature range from 20 to 60°C.
Agreement of directly measured and MRTh-calculated temperature values for the T1- and PRF-based MRTh is shown for the shortest (2.4 ms) and the longest (15 ms) echo-time (). The Bland-Altman analysis presenting mean of differences ±95% limits of agreement (1.96·standard deviation of the differences T(probe)-T(MR)) were 1.6 ± 5.3 for the PRF-MRTh (TE = 2.4 ms), −0.1 ± 1.5 for the PRF-MRTh (TE = 15 ms), 0.5 ± 2.0 for the T1-MRTh (TE = 2.4 ms) and −1.5 ± 3.5 for the T1-MRTh (TE = 15 ms), respectively. No temperature dependent error was found for both MRTh methods inside the measured area from 20°C up to 60°C, whereas both MRTh methods show statistically distributed errors for short TE.
Figure 5. Mean-difference plots (Bland-Altman Plots) show the comparison between measured temperature with the optical temperature probe and calculated absolute MR-temperature with a two-parameter model ΔTMR =Tref_min+b · TMR. Results with lowest and highest echo-times for both thermometric methods (PRF, T1) are shown, the means and ±95% limits of agreement are indicated: (A) PRF-thermometry, TE = 2,4ms; (B) T1-thermometry, TE = 2,4ms; (C) PRF-thermometry, TE = 15ms; (D) T1-thermometry, TE = 15ms.
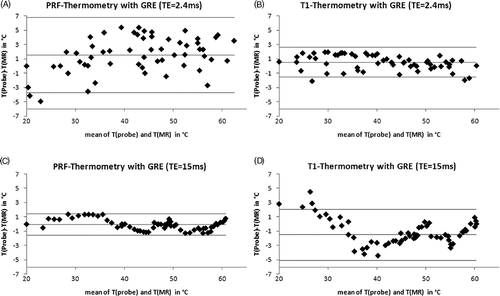
MRTh colour-coded plots were mapped over magnitude image data () taken from a heating experiment, which was dynamically monitored with the GRE (TE = 10 ms). The areas of heating were consistent with the procedure and nearly identical for both MRTh calculation methods. No motion artefacts were observed during image acquisition (7.8 s).
Figure 6. MRTh is calculated from image data during a laser ablation in human cadaveric disc. For imaging the GRE was used (TE = 10ms). Color-coded thermographic plots were mapped over the corresponding MR magnitude images. Calculations based on T1-MRTh (left) and on PRF-MRTh (right) show the heat spread inside the nucleus. The image data were taken at different points during the experiment: (A) after 2 min, (B) after 6min and (C) after 10 min of lasing with a NdYAG laser (1064 nm).
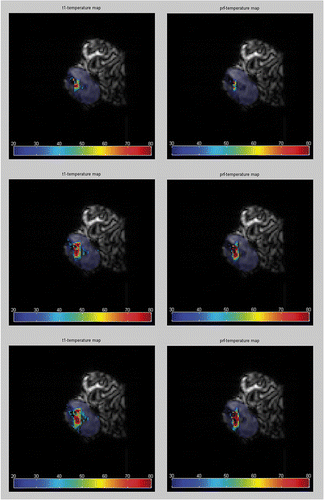
Discussion
To improve the effectiveness and safety of laser ablation therapy in the lumbar spine, both anatomical and thermometric MR monitoring is desirable. In contrast to the bore-like MR scanners, those with open configuration design permit easy access to the patient and more flexible interventional manipulation. In comparison with lower magnetic fields associated with the most open MR scanners, the relatively high field strength of 1 T is expected to improve image quality and increase temperature accuracy. In the last years great progress has been made in research and clinical application of MRTh Citation[7], Citation[16], Citation[28–30]. However, so far, no MRTh application is commercially available. Due to the clinical protocols and applications, every user has to optimise their specific MRTh approach. This depends on the anatomical region and the target tissue, field strength of the MR scanner and configuration, as well as the heat source used. As a consequence, various pulse sequences and calculation algorithms have been proposed. Therefore, the published documentation of several research groups can only provide slight orientation.
To enable comparability, we systematically investigated fast pulse sequences with different gradient-echo readouts, suitable for real-time feedback and capable of MRTh. We optimised each sequence with regard to image quality (anatomical details, needle artefacts, SNR) both in vitro and in vivo. The evaluation of temperature accuracy was performed in vitro. The investigated MRTh methods were based on the two endogenous MR temperature indicators: T1 and PRF. The optimal compromise between acquisition time, image quality and temperature accuracy was achieved with a non-spoiled gradient-echo sequence at the echo time of TE = 10 ms. Although only partially acquired in vivo, these results are promising for applications in vivo.
Relaxation times studies
The theoretical requirement for PRF-MRTh is TE = T2*. De Zwart et al. Citation[32] have shown that with TE = T2*, the temperature-sensitive signal to noise ratio (SNRT = TE · exp(−TE/T2*)) of PRF-MRTh reaches its maximum, while the variance of the calculated temperature reaches its minimum. In consequence, T2* relaxation data of disc tissue at 1.0 T were needed. Bottomley et al. Citation[32], listed T1 and the spin-spin relaxation time (T2) for several tissue types at different field strengths. T1 and T2 relaxation times have been measured in 355 discs in a 1.5 T closed-configuration MR scanner Citation[33]. Unfortunately, no relaxation time data for a field strength of 1.0 T and disc tissue is available. As previously shown by Boos et al. Citation[34], age and the extent of herniation have a significant influence on the relaxation times. Our measurements were performed on healthy volunteers. Although the sample size in this study was small, we were able to show that older individuals have longer T2* relaxation times. Age-related dehydration, as well as the summation of biochemical effects, which are associated with disc degeneration, contribute to increased proton relaxation times Citation[34]. We measured the shortest effective spin-spin relaxation times (about 30 ms) in the discs of healthy young volunteers (20–30 years). Thus, for degenerated discs T2* values at 1.0 T are expected to be even higher.
It was not possible to fulfil the theoretical requirements for high temperature precision (TE = T2*) for PRF-MRTh. Optimisation of the acquisition parameter TE was limited by the increasing needle artefact with increasing TE Citation[22], that occurs within heat induced lesion. In fact, we used half of the predicted optimal TE. The PRF-MRTh provided temperature accuracies of ±2°C and better, which has proposed as the maximum tolerable error for high-temperature MRTh Citation[29].
Sequence studies
The comparison of four gradient-recalled sequence types (PRESTO, EPI, T1W-GRE, GRE), typically used for thermometric monitoring, was carried out with regard to image quality and temperature sensitivity. A spatial resolution of 2.8 * 2.8 * 4 mm3 was found to show sufficient SNR of the nucleus in magnitude images. In using echo-planar imaging techniques (PRESTO, EPI) we were not able visualise smaller anatomic structures or heat-induced lesions round the cannula. Both sequences were insufficient to monitor the spinal region with its high susceptibility gradients caused by resonance offset artefacts, which lead to image destruction at susceptibility boundaries. Using the EPI technique, the image distortion in phase encoding direction is stronger than the distortion in frequency-encoding direction Citation[34]. Hence, we used multi-shot EPI techniques to reduce artefacts in phase encoding direction. Susceptibility artefacts at tissue/bone interfaces and the needle artefact size could not be reduced by the variation of readouts after excitation (EPI-factor), which affect image distortion as well as temperature sensitivity Citation[28], or the variation of BW in frequency-encoding direction. Magnitude images of the GRE and T1W-GRE showed the best anatomical detail, the highest SNR and the smallest needle artefact.
We made compromises in temporal resolution by using the longest acquisition time of the GRE for all sequences to ensure comparability. Acquiring three slices is sufficient to survey the ablation volume, as well as the heat spread to neighbouring tissues. This finally leads to a temporal solution of 8 s. Although the EPI exhibited good temperature sensitivity in both methods, we are aware that the EPIs’ temporal resolution can be improved.
The slight systematic offset between MRTh and direct temperature measurements expressed by the ordinate point of intersection in are caused by the high spatial temperature and susceptibility gradients around the needle. We eliminated systematic errors by using a fibre-optic probe with a tip size of half a voxel and a response time that is much shorter than a single-scan duration. We ensured comparability with an invariable experimental set-up, regular geometry () and constant temperature ranges (). The slightly changing composition of the cadaveric specimens might be the reason for the differences in the slope of correlation (). During our in vitro heating experiments, no motion artefacts occurred. Other well known sources of error during MRTh such as changes of the thermal coefficient depending on the tissue type and the heating method Citation[35] as well as the temperature dependent susceptibility, which was examined by dePoorter Citation[36] in fat and muscle tissue, were precluded in this study with respect to spatial and temporal MRTh resolution.
Good agreement between MRTh and direct temperature measurements could be shown with varying TEs for the GRE. Temperature errors were small although we reduced the TE down to 10 ms for the PRF-MRTh (±1.11°C) and increased the TE up to 15 ms for the T1-MRTh (±1.78°C). Moreover, comparable heat patterns could be shown in in vitro MRTh with a GRE (TE = 10 ms).
Gradient echo-based sequences have been reported to be promising for MRTh during high-temperature interventions. The requirements for monitoring of high temperature gradients in small anatomical regions are high temporal and spatial resolution. Since both requirements cannot both be met at one time, compromise solutions have to be found. Accelerated gradient echo techniques such as PRESTO have been used for MRTh control at 4.7 T during ablative interventions in liver and thigh Citation[31], Citation[37]. Other groups have evaluated EPI with varying echo train lengths at 1.5 T during radio-frequency ablation of liver tissue Citation[28]. Further research groups used T1W-GRE in an open 0.5 T system during PLDD Citation[15] and have investigated unspoiled GRE at 1.5 T to monitor high focused ultrasound hyperthermia Citation[26]. In an open system at 1.0 T, we decided to use the GRE due to its high SNR in disc and neighbouring tissues and the induction of discrete needle artefacts.
Moreover, we were able to achieve good temperature accuracy using the GRE in the full range of tested TEs. When using T1-MRTh, the sequence should first be calibrated for the specific tissue, since spin-lattice-relaxation and its temperature-induced signal changes are strongly tissue-dependent. T1-MRTh is considered to be linear up to a coagulation temperature of 60°C Citation[38] and is proven to be more sensitive at relatively low field strengths Citation[7].
The advantage of PRF-MRTh of discal laser therapies is that the nucleus and the annulus are fat free. It is well known that errors related to the presence of lipids lead to temperature errors of PRF-MRTh Citation[7], Citation[16], Citation[20], Citation[29]. Subsequently, when monitoring disc tissue with PRF-MRTh, these errors are irrelevant. There is no doubt, that MRTh is an attractive option to optimise therapeutic efficacy and safety of thermal interventions. MR monitoring of high-temperature thermal therapies offers not only anatomical information on the treated regions but also allows flash temperature mapping. PLDD, especially under MR fluoroscopy, is a therapy that has become increasingly popular over the last 20 years. Thermometric monitoring can be helpful to survey the vulnerable structures surrounding the disc, i.e. endplates, the spinal canal and nerve roots, which have to be protected from excessive heat.
Conclusion
In this in vitro feasibility study in the 1.0 T open MR scanner, we were able to show sufficient temperature accuracy (better than ±2°C) for non-spoiled gradient-recalled echo sequences. Further, we were able to increase temperature accuracy by varying calculation techniques (T1-MRTh, PRF-MRTh) with respect to sequence parameters (TE, TR). Whether T1-MRTh or PRF-MRTh should be regarded as the method of choice has to be investigated further in clinical studies.
Acknowledgements
The project is financed by a public grant (TSB Technologiestiftung Berlin–Zukunftsfonds Berlin). Co-financed by the European Union–European fund for regional development (project number: 10132816/10134231).
Declaration of interest: The authors report no conflicts of interest. The authors alone are responsible for the content and writing of the paper.
References
- Diederich CJ. Thermal ablation and high-temperature thermal therapy: Overview of technology and clinical implementation. Int J Hyperthermia 2005; 21: 745–753
- Stauffer PR, Goldberg SN. Introduction: Thermal ablation therapy. Int J Hyperthermia 2004; 20: 671–677
- Choy DS. Percutaneous laser disc decompression: A 17-year experience. Photomed Laser Surg 2004; 22: 407–410
- Gronemeyer DH, Buschkamp H, Braun M, Schirp S, Weinsheimer PA, Gevargez A. Image-guided percutaneous laser disk decompression for herniated lumbar disks: A 4-year follow-up in 200 patients. J Clin Laser Med Surg 2003; 21: 131–138
- Steiner P, Schoenenberger AW, Erhart P, Penner E, von Schulthess GK, Debatin JF. Imaging temperature changes in an interventional 0.5 T magnet: In-vitro results. Lasers Surg Med 1997; 21: 464–473
- Ishiwata Y, Takada H, Gondo G, Osano S, Hashimoto T, Yamamoto I. Magnetic resonance-guided percutaneous laser disk decompression for lumbar disk herniation–relationship between clinical results and location of needle tip. Surg Neurol 2007; 68: 159–163
- Quesson B, de Zwart JA, Moonen CT. Magnetic resonance temperature imaging for guidance of thermotherapy. J Magn Reson Imaging 2000; 12: 525–533
- Hynynen K, McDannold N. MRI guided and monitored focused ultrasound thermal ablation methods: A review of progress. Int J Hyperthermia 2004; 20: 725–737
- Hynynen K, Clement G. Clinical applications of focused ultrasound-the brain. Int J Hyperthermia 2007; 23: 193–202
- Vogl TJ, Straub R, Zangos S, Mack MG, Eichler K. MR-guided laser-induced thermotherapy (LITT) of liver tumours: Experimental and clinical data. Int J Hyperthermia 2004; 20: 713–724
- Rieke V, Kinsey AM, Ross AB, Nau WH, Diederich CJ, Sommer G, Pauly KB. Referenceless MR thermometry for monitoring thermal ablation in the prostate. IEEE Trans Med Imaging 2007; 26: 813–821
- Grenier N, Quesson B, de Senneville BD, Trillaud H, Couillaud F, Moonen C. Molecular MR imaging and MR-guided ultrasound therapies in cancer. JBR-BTR 2009; 92: 8–12
- Streitparth F, Gebauer B, Melcher I, Schaser K, Philipp C, Rump J, Hamm B, Teichgraber U. MR-guided laser ablation of osteoid osteoma in an open high-field system (1.0 T). Cardiovasc Intervent Radiol 2009; 32: 320–325
- Jakob PM, Hendrich C, Breitling T, Schafer A, Berden A, Haase A. Real time monitoring of laser-induced thermal changes in cartilage in vitro by using snapshot FLASH. Magn Reson Med 1997; 37: 805–808
- Schoenenberger AW, Steiner P, Debatin JF, Zweifel K, Erhart P, von Schulthess GK, Hodler J. Real-time monitoring of laser diskectomies with a superconducting, open-configuration MR system. Am J Roentgenol 1997; 169: 863–867
- Rieke V, Pauly Butts K. MR thermometry. J Magn Reson Imaging 2008; 27: 376–390
- Dickinson RJ, Hall AS, Hind AJ, Young IR. Measurement of changes in tissue temperature using MR imaging. J Comput Assist Tomogr 1986; 10: 468–472
- Ishihara Y, Calderon A, Watanabe H, Okamoto K, Suzuki Y, Kuroda K, Suzuki Y. A precise and fast temperature mapping using water proton chemical shift. Magn Reson Med 1995; 34: 814–823
- Steiner P, Zweifel K, Botnar R, Schoenenberger AW, Debatin JF, von Schulthess GK, Hodler J. MR guidance of laser disc decompression: Preliminary in vivo experience. Eur Radiol 1998; 8: 592–597
- McDannold N. Quantitative MRI-based temperature mapping based on the proton resonant frequency shift: Review of validation studies. Int J Hyperthermia 2005; 21: 533–546
- Streitparth F, Walter T, Wonneberger U, Chopra S, Wichlas F, Wagner M, Hermann KG, Hamm B, Teichgraber U. Image-guided spinal injection procedures in open high-field MRI with vertical field orientation: Feasibility and technical features. Eur Radiol 2009, DOI: 10.1007/s00330-009-1567-3
- Wonneberger U, Schnackenburg B, Streitparth F, Walter T, Rump J, Teichgraber UK. Evaluation of magnetic resonance imaging-compatible needles and interactive sequences for musculoskeletal interventions using an open high-field magnetic resonance imaging scanner. Cardiovasc Intervent Radiol 2009, DOI: 10.1007/s00270-009-9676-6
- Look DC, Locker DR. Time saving in measurement of NMR and EPR relaxation times. Rev Sci Instruments 1970; 41: 250–251
- Wlodarczyk W, Hentschel M, Wust P, Noeske R, Hosten N, Rinneberg H, Felix R. Comparison of four magnetic resonance methods for mapping small temperature changes. Phys Med Biol 1999; 44: 607–624
- Germain D, Vahala E, Ehnholm GJ, Vaara T, Ylihautala M, Savart M, Laurent A, Tanttu J, Saint-Jalmes H. MR temperature measurement in liver tissue at 0.23 T with a steady-state free precession sequence. Magn Reson Med 2002; 47: 940–947
- Cline HE, Hynynen K, Schneider E, Hardy CJ, Maier SE, Watkins RD, Jolesz FA. Simultaneous magnetic resonance phase and magnitude temperature maps in muscle. Magn Reson Med 1996; 35: 309–315
- De Poorter J, De Wagter C, De Deene Y, Thomsen C, Stahlberg F, Achten E. Noninvasive MRI thermometry with the proton resonance frequency (PRF) method: In vivo results in human muscle. Magn Reson Med 1995; 33: 74–81
- Cernicanu A, Lepetit-Coiffe M, Roland J, Becker CD, Terraz S. Validation of fast MR thermometry at 1.5 T with gradient-echo echo planar imaging sequences: Phantom and clinical feasibility studies. NMR Biomed 2008; 21: 849–858
- de Senneville BD, Mougenot C, Quesson B, Dragonu I, Grenier N, Moonen CT. MR thermometry for monitoring tumor ablation. Eur Radiol 2007; 17: 2401–2410
- Denis de Senneville B, Quesson B, Moonen CT. Magnetic resonance temperature imaging. Int J Hyperthermia 2005; 21: 515–531
- de Zwart JA, van Gelderen P, Kelly DJ, Moonen CT. Fast magnetic-resonance temperature imaging. J Magn Reson B 1996; 112: 86–90
- Bottomley PA, Hardy CJ, Argersinger RE, Allen-Moore G. A review of 1H nuclear magnetic resonance relaxation in pathology: Are T1 and T2 diagnostic?. Med Phys 1987; 14: 1–37
- Boos N, Dreier D, Hilfiker E, Schade V, Kreis R, Hora J, Aebi M, Boesch C. Tissue characterization of symptomatic and asymptomatic disc herniations by quantitative magnetic resonance imaging. J Orthop Res 1997; 15: 141–149
- Dragonu I, de Senneville BD, Quesson B, Moonen C, Ries M. Real-time geometric distortion correction for interventional imaging with echo-planar imaging (EPI). Magn Reson Med 2009; 61: 994–1000
- Peters RD, Hinks RS, Henkelman RM. Ex vivo tissue-type independence in proton-resonance frequency shift MR thermometry. Magn Reson Med 1998; 40: 454–459
- De Poorter J. Noninvasive MRI thermometry with the proton resonance frequency method: Study of susceptibility effects. Magn Reson Med 1995; 34: 359–367
- de Zwart JA, Vimeux FC, Delalande C, Canioni P, Moonen CT. Fast lipid-suppressed MR temperature mapping with echo-shifted gradient-echo imaging and spectral-spatial excitation. Magn Reson Med 1999; 42: 53–59
- Graham SJ, Stanisz GJ, Kecojevic A, Bronskill MJ, Henkelman RM. Analysis of changes in MR properties of tissues after heat treatment. Magn Reson Med 1999; 42: 1061–1071