Abstract
Purpose: The aim of this study is to investigate the effects of an arterial bifurcation on the temperature distribution during microwave ablation in a muscle-equivalent phantom.
Methods: Two experiments with water flow rates of 42.39 mL/min and 70.79 mL/min in the typical range of blood flows in the hepatic artery of the human body were implemented. Temperature measurements inside the phantom were performed in the plane of the arterial bifurcation, in each experiment the microwave antenna was placed at three different positions at 10, 15 and 20 mm from the vessel.
Results: The heating pattern was not symmetrical around the antenna with large temperature gradients near the blood vessel when the antenna was near to the vessel (10 mm): The higher the blood velocity, the smaller the heated area. The heating pattern was more circular and symmetrical, and the temperature contours with the two given flow rates nearly coincide when the antenna was far away from the blood vessel (20 mm). The temperatures near the recirculation zone in the daughter arteries immediately after the bifurcation hardly vary with blood velocity.
Conclusion: These results indicate that the flow rate in the vessel and the distance between the vessel and the antenna can significantly affect the heating pattern during thermal ablation. The effect of blood flow on ablation is negligible if the distance between blood vessel and antenna exceeds 20 mm, and vessel occlusion can be avoided. The present results can be helpful in clinical microwave ablation surgical planning.
Introduction
Hepatocellular carcinoma (HCC) remains a challenging medical problem since it is responsible for more than one million deaths per year worldwide Citation[1]. Surgical resection is the first choice for HCC, but it is not a viable option for all patients due to poor liver function induced by chronic liver disease Citation[2]. Transcatheter arterial embolisation and ultrasound-guided percutaneous ethanol injection therapy are ineffective in some aspects Citation[3–5]. However, microwave ablation, which aims to destroy the tumour by increasing the temperature of malignant tissues to above 54°C by microwave energy Citation[6], is effective because it can create a deeper ablation lesion.
Anatomically, there are usually large blood vessels crossing through or surrounding a tumour. Theoretical, experimental and clinical studies have demonstrated that large blood vessels can produce localised steep cooling in heated tissues during microwave ablation therapy because of the convective effect of blood flow Citation[7–10]. Thus, steep temperature decrease may occur around the vessels, which can significantly affect the ablation lesion when the target tissue is heated.
The bio-heat transfer between tissue and blood flow is important in the temperature distribution of thermal ablation. The research on blood flow effects will be helpful for decision making or surgical planning in microwave ablation therapy. Developments in this direction are two-fold, comprising the continuum model and the discrete vessel model and are reflected in the contributions from Pennes classical bio-heat equation on the resting human forearm, such as Chen and Holmes 50–300 µm diameter thermally significant counter-current artery vein model Citation[11]. Important milestones also include Chato's analysis of individual blood vessels Citation[12] and the three-layered microcirculatory model by Weinbaum and Jiji Citation[13].
Some works aim to demonstrate the effects of large blood vessels on temperature distribution Citation[14–16]. Unfortunately, little attention has so far been paid to arterial bifurcation, which is the representative feature of blood vessel networks Citation[14–18]. The flow at a bifurcation is significantly disturbed, which may result in complicated flow patterns that can affect the vicinal temperature distribution in thermal ablation Citation[19].
Some papers solved this problem with theoretical models where the given conditions seemed idealistic Citation[11–14]. As an alternative, a numerical simulation was performed to demonstrate a more complex heat transfer situation Citation[19]. However, the experimental method should be used to investigate the effects of arterial bifurcation on the temperature distribution of thermal ablation.
In the present experimental study, a typical arterial bifurcation was selected to address the question of how a thermally significant arterial bifurcation may affect the temperature field of microwave ablation. Attention was paid to the variation of the ablation shape with the microwave antenna position and the flow rate in the arterial bifurcation.
Materials and methods
Experimental system
The experimental system is shown schematically in Citation[20], Citation[21], which consists of a microwave generator, thermocouples and peristaltic pump.
Microwave generator
A model MTC-3 microwave generator (designed by the Institute of Microwave Electronics, Nanjing Qinghai, China) was used in the present microwave ablation study. It works at 2450 ± 50 MHz with a power output of 0–100 W. The system was equipped with a low-loss cable and a water-cooled microwave antenna (1.9 mm in diameter) with a surface coating to prevent tissue adhesion. The structure of the antenna and the water circulation is shown in .
Thermocouples
Copper-constantan thermocouples (TCs), which have low microwave pickup, fast time rise, and good temperature sensitivity and reliability Citation[22], were used to measure the temperature near the branching blood vessel. The TCs, which connect with the data acquisition/switch unit (Agilent HP34970A, Santa Clara, CA, USA), were calibrated in a mixture of ice and water, and an accuracy of ±0.1°C was obtained.
Peristaltic pump
The flow rates of water in the tube-simulated blood vessel and the cooling water in the microwave antenna can be continuously modulated by two separate peristaltic pumps.
Experimental materials
For the convenience of the temperature measuring with TCs, the experiment was performed in a muscle-equivalent microwave phantom instead of liver tissue Citation[23]. shows the properties of the phantom and liver tissue which demonstrates that the thermal properties of the phantom are close to those of biological liver tissue.
Table I. Properties of phantom and liver.
The blood vessel was simulated by a copper tube with an internal diameter of 3 mm and a wall thickness of 0.5 mm. The thermal conductivity of copper is so high that we assume the ‘blood’ in the tube to be connected with the ‘tissue’ directly.
Experimental method
In the experiment, the arterial bifurcation and TCs were fixed on a specially designed bracket, which was placed into a cylindrical organic glass box as a phantom container. The phantom was left in the lab room for 12 h for de-aeration and to cool completely to a relatively uniform temperature before the phantom was set into the box. TCs were placed at the sites of interest in the same plane as the bifurcation axes. The microwave antenna was perpendicular to this plane, and the generator was just on the plane. A 60 W power supply was set for 5 min continuous heating. The water flow rates in the vessel were steady at 42.39 mL/min and 70.79 mL/min corresponding to the hepatic artery flow rates of 127.17 mL/min and 211.95 mL/min with the Reynolds numbers of about 300 and 500 respectively Citation[10]. TCs started to measure temperature at the instant when the microwave power was turned on. The temperature was recorded every three seconds at each site. After 5 min heating, the power of the microwave was turned off and the phantom was cooled to room temperature. The procedure was repeated three times under the same conditions, and the final temperature data were the averages of the three measured values.
The microwave antenna was inserted into the phantom beside the bifurcation at three different positions as illustrated in , where the dots denote different positions of the antenna with the distance between two neighbouring positions of 5 mm. D denotes the distance between the antenna and the central line of the blood vessel. At the first position, D = 10 mm.
shows the sites of TCs with three different antenna positions. With the increase of D, more TCs were arranged in the experiment to get accurate temperature profiles. The TCs were positioned on the right hand of the blood vessel because the temperature on the left hand of the blood vessel was found not to reach 54°C in primary experiments.
Experimental data processing
The thermal field was represented by isotemperature contours in this study, and the isotemperature contours were obtained from the TCs measured temperature by interpolation and extrapolation using the software TableCurves (Sigma-Aldrich, St Louis, MO). Thus, the isotemperature contours in the area without thermometry might not be exactly accurate, especially in very close vicinity to the vessels.
Experimental results
Temperature curves in the vicinity of blood vessel
Some temperature curves in the vicinity of the blood vessel with different blood velocities are depicted in when the antenna was at the first position (D = 10 mm). The temperatures with 42.39 mL/min blood velocity were higher than those with 70.79 mL/min blood velocity at the same TC site, because the blood flow with higher flow rate took more heat away. When the TCs approached the antenna, such as TC No.8, TC No.15 and TC No.21 in , the temperature increased very quickly. Contrarily, when the TCs were far away from the antenna, such as TC No.1 and TC No.27, the temperature increased slowly.
Figure 5. Experimental temperature curves for distance between antenna and vessel D = 10 mm (A) TC No.1; (B) TC No.8; (C) TC No.15; (D) TC No.21; (E) TC No.27.
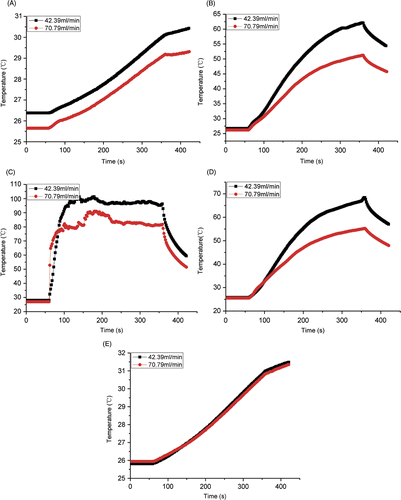
The temperature of TC No.15 rose more rapidly than other TCs because TC No.15 was near the antenna where microwave energy played an important role. There was a temperature fluctuation when the TC was near the copper tube and the antenna (), while no fluctuation was found at other sites for large distance (). This phenomenon may be due to the use of copper tube as a blood vessel, which has microwave interaction.
The temperature of TC No.27 was higher than the temperature of TC No.1 although the distance between TC No.1 and the antenna was the same as that between TC No.27 and the antenna. The reason for this is that TC No.27 was near the daughter artery, where the blood velocity was lower than the mother artery, and hence the convection effect was smaller.
The temperature curves of TC No.27 almost overlapped. It seems that the different blood flow doesn’t affect the temperature at this site. Usually, there is a recirculation region in the daughter artery immediately after the bifurcation. The convective effect of the recirculation zone on the temperature field is very weak Citation[19]. TC No.27 was near the recirculation zone of arterial bifurcation where the velocity was low and thus kept the same temperature even though the flow rate changed in the mother artery.
The maximum tissue temperatures for the three distances plotted in show that the maximum temperature does not continue increasing with the distance between antenna and the bifurcation for a distance of 25 mm. This was due to the effect of the recirculation on the temperature distribution.
Influence of the position of the antenna on heating pattern
The 54°C temperature contours under the condition of blood flow rate 42.39 mL/min at different times after heating with D = 10 mm, 15 mm and 20 mm are shown in respectively. The region above 54°C is defined as the ablation lesion, i.e. the therapeutic region. It was found that a large temperature gradient existed near the blood vessel.
Figure 7. Experimental temperature contours (54°C) after 60 s, 120 s, 180 s, 240 s and 300 s of heating for different distances D between the antenna and the blood vessel with a blood flow of 42.39 mL/min. (A) D = 10 mm; (B) D = 15 mm; (C) D = 20 mm.
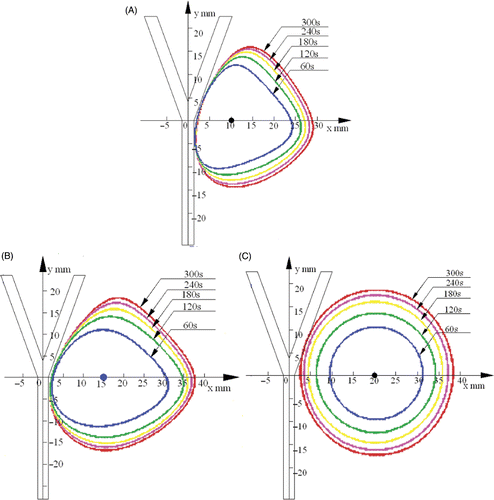
The heating patterns were different at different antenna positions. In these series, the antenna was placed at three positions. When the antenna was at the first position (D = 10 mm) as shown in , the heating pattern was not evidently symmetrical. This phenomenon can be explained as follows: the blood flow can take away the heat, restrain the diffusion of heat to the left side, and make the heating pattern a right excursion. When the antenna was further away (D = 15 mm) as shown in , the heating pattern was more circular and symmetrical because the influence of the convection of the blood flow was smaller compared with the first position (D = 10 mm). When the antenna was at the third position (D = 20 mm) as shown in , the heating pattern was almost a circle and thus the blood flow had the least convective effect on the temperature field.
In order to verify the accuracy of the experimental results, the ablation pattern and size was compared between numerical and experimental results. The simulations were performed by ANSYS 9.0 in this study. shows the heating pattern in the simulation, and the contours were also set to 54°C. The numerical values agree well with the experimental pattern and the ablation length, as can be seen in .
Figure 8. Simulated temperature contours (54°C) for different distances, D, between the antenna and the blood vessel with a blood flow of 42.39 mL/min. (A) D = 10 mm; (B) D = 15 mm; (C) D = 20 mm.
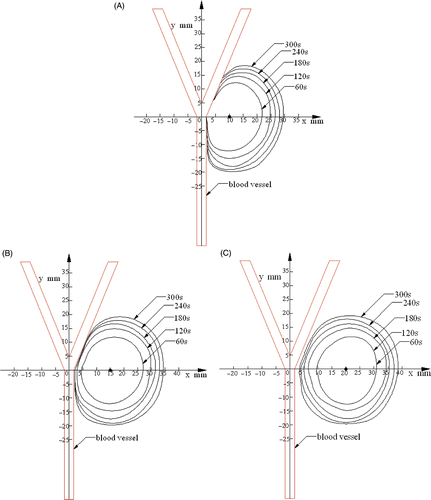
Table II. Comparison of the coagulation region.
The experimental temperature profiles along the vessel (D = 10, 15, 20 mm) are displayed in . The temperatures reduced with the distance between the antenna and the blood vessel increased.
Figure 9. Experimental temperature profiles along the blood vessel for different distances D between the antenna and the blood vessel.
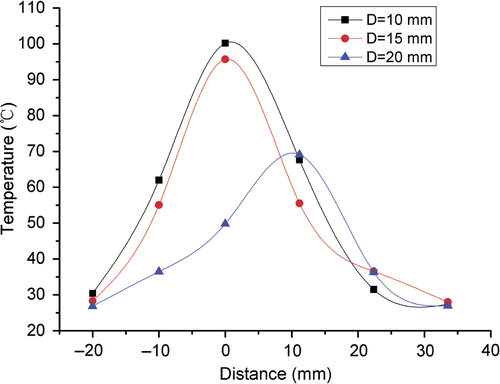
The experimental and the numerical temperature profiles along the vessel are compared in . The experimental profiles were plotted by TC No.1, 8, 15, 21, 27, 32 where the blood flow rate was 42.39 mL/min. The profiles agreed well, and the statistical analysis showed that there was no significant change in temperature between numerical and experimental results.
Figure 10. Experimental and simulated temperature profiles along the blood vessel with a blood flow of 42.39 mL/min for D = 10 mm.
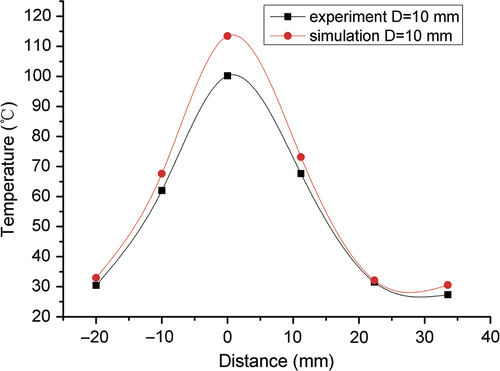
Results of the heating pattern when the antenna was placed at three different positions at the time of 300 s with a water flow rate of 42.39 mL/min are shown in .
Influence of flow rate on heating pattern
shows the 54°C temperature contours under the conditions of blood flow rates 42.39 mL/min and 70.79 mL/min at the time of 300 s after heating with the antenna located at two different positions respectively. In , the water flow had a significant effect on the heat transfer in the phantom, and the heating pattern with blood flow rate of 42.39 mL/min was larger than that of with 70.79 mL/min. The two heating patterns overlapped on the right, and they were slightly different just near the blood vessel. In , the influence of the flow rate can be ignored because the temperature contours in both cases almost overlapped. However, the blood vessel still affected the temperature distribution since the left area of the heating pattern was slightly attenuated by the cooling effect of blood flow.
No-flow and blocked-daughter measurements
One may suspect that the copper tube was a cooling effect. Therefore, a measurement with no-flow of cooling water in the copper tube was carried out in order to check whether the copper tube is an appropriate material to replace the blood vessel. The temperatures in TC No.15 and TC No.16 () which were located at the same distance to the antenna with TC No.15 closer to the copper tube are shown in . The two similar measurements indicate that the copper tube is probably an acceptable material. In addition, a measurement with the blocked daughter of the left branch was performed to demonstrate the influence of the bifurcation of the blood vessel. shows the temperatures in TC No.1 and TC No.27. The two measurements were almost the same, which confirmed that the present experimental model could reflect the influence of bifurcation on ablation temperature without considering the effect of blood vessel curvature.
Discussion and conclusions
In this paper, an experimental study on the effects of arterial bifurcation on the temperature distribution of microwave ablation was performed. The present experiments demonstrated qualitatively the influence of water flow in arterial bifurcation on the phantom ablation. It was found that blood flow played an important role in the temperature field when the antenna was near the arterial bifurcation. For the arterial bifurcation, the higher the flow rate in the tube, the more heat will be taken away, and thus the tissue near the blood vessel cannot reach the therapeutic temperature (54°C). The experiment also indicated that the effect of blood on the temperature field can be ignored if the distance between blood vessel and antenna exceeds 20 mm. Moreover, there is a recirculation zone in the daughter artery immediately after the bifurcation which actually acts as a thermal insulating layer between the tissue and the blood. The temperature near the recirculation zone doesn’t vary with the blood velocity. Our previous computational simulation has predicted the presence of this zone Citation[19] and the simulation results were also confirmed by the experiments in this study.
This research may provide experimental reference for the processing method of arterial bifurcation in microwave ablation therapy in clinical application. In clinical therapy doctors can determine whether to block the blood vessel or not according to the position of the tumour, the velocity of blood flow and the distance between antenna and blood vessel to get good therapeutic results.
However, the present study is only a preliminary investigation on the cooling effect of arterial bifurcation in microwave ablation, and still has some limitations. Firstly, the number of TCs was not enough and therefore some temperatures were obtained by numerical interpolation which might not be accurate. Secondly, the blood vessel was simulated by copper tube which can enhance the heat convection of blood flow since it has higher thermal conductivity than blood vessel. Therefore, the thermal field in the present study may be smaller than the real one. As we know, the flow has a much bigger cooling effect. As a comparison, other materials would probably be better for mimicking the blood vessel in further studies. Thirdly, in order to keep the same Reynolds number as human blood flow, the flow rate of cooling water was reduced which could reduce the convective heat transfer too. The better way may be to keep the same Nusselt number to maintain consistent convective heat transfer. Finally, the TCs were placed in the plane of the blood vessel, and a 2D temperature field was investigated. In a future study, the TCs can be placed on other planes, and then a 3D temperature field can be investigated. This phantom is a non-perfused phantom, which is acceptable for the present study where ablation was studied. A phantom with perfused ‘tissue’ should be used for the lower temperature long duration hyperthermia range (<45°C) as this will certainly affect the patterns shown. Nevertheless, efforts made in this paper can help promote further investigation in this promising direction.
Declaration of interest: This research is supported by the National Science Foundation of China (10872013, 10772010 and 30470450), Beijing Natural Science Foundation (3092004, 3092005 and 3062003).
References
- Liang P, Dong BW, Yu XL, Yu DJ, Cheng ZG, Li S, Peng JS, Nan Q, Wang HJ. Computer-aided dynamic simulation of microwave-induced thermal distribution in coagulation of liver cancer. IEEE Trans Biomed Eng 2001; 48: 821–829
- Seki T, Wakabayashi M, Nakagawa T, Imamura M, Tamai T, Nishimura A, Yamashiki N, Okamura A, Inoue K. Percutaneous microwave coagulation therapy for patients with small hepatocellular carcinoma. Cancer 1999; 85: 1694–1702
- Seki T, Nonaka T, Kubota Y, Mizuno T, Sameshima Y. Ultrasonically guided percutaneous ethanol injection therapy for hepatocellular carcinoma. Am J Gastorenterol 1989; 84: 1400–1407
- Ebara M, Ohto M, Sugiura N, Kita K, Yoshikawa M, Okuda K, Kondo F, Kondo Y. Percutaneous ethanol injection for the treatment of small hepatocellular carcinoma study of 95 patients. J Gastroenterol Hepatol 1990; 5: 616–626
- Yu ZC, Liu WC, Fan L, Shao J, Huang Y, Si XM. The efficacy and safety of percutaneous microwave coagulation by a new microwave delivery system in large hepatocellular carcinomas: Four case studies. Int J Hyperthermia 2009; 25: 392–398
- Dong BW, Liang P, Yu XL, Zeng XQ, Wang PJ, Su L, Wang XD, Xin H, Li S. Sonographically guided microwave coagulation treatment of liver cancer: An experiment and clinical study. Am J Roentgenol 1998; 171: 449–454
- Michael R Horsman. Angiogenesis and vascular targeting: Relevance for hyperthermia. Int J Hyperthermia 2008; 24: 57–65
- Kolios MC, Sherar MD, Hunt JW. Large blood vessel cooling in heated tissues: A numerical study. Phys Med Biol 1995; 40: 477–494
- Haemmerich D, Wright AW, Mahvi DM, Webster JG, Lee FT, Jr. Hepatic bipolar radiofrequency ablation creates coagulation zones close to blood vessel: A finite element study. Med Biol Eng Comput 2003; 41: 317–323
- Liang P, Dong BW. Ultrasound-guided microwave coagulation therapy for liver cancer. People's Military Medical Press, Beijing 2003; 71–84
- Chen MM, Holmes KR. Microvascular contributions in tissue heat transfer. Ann NY Acad Sci 1980; 335: 137–150
- Chato JC. Heat transfer to blood vessels. ASME J Biomech Eng 1980; 102: 110–118
- Weinbaum S, Jiji LM. A new simplified bioheat equation for the effect of blood flow on local average tissue temperature. ASME J Biomech Eng 1985; 107: 131–139
- Baish JW, Ayyaswamy PS, Foster KR. Heat transport mechanisms in vascular tissue: A model comparison. J Biomech Eng 1986; 108: 324–331
- Chen ZP, Roemer RB. The effects of large blood vessels on temperature distribution during simulated hyperthermia. J Biomech Eng 1992; 114: 473–481
- Tungjitkusolmun S, Staelin ST, Tsai JZ, Webster JG, Lee FT, Jr, Mahvi DM, Vorperian VR. Three-dimensional finite-element analysis for radio-frequency hepatic tumor ablation. IEEE Trans Biomed Eng 2002; 49: 3–9
- Lubashevsky, Gafiychuk VV, Datsko BY. Anomalous properties of heat diffusion in living tissue caused by branching artery network. Qualitative description. Retrieved from: http://arxiv.org/abs/cond-mat/0201057.
- Sheu TW, Chou CW, Tsai SF, Liang PC. Three-dimensional analysis for radio-frequency ablation of liver tumor with blood perfusion effect. Comp Meth Biomech Biomed Eng 2005; 8: 229–240
- Liu YJ, Qiao AK, Nan Q, Yang XY. Thermal characteristics of microwave ablation in the vicinity of an arterial bifurcation. Int J Hyperthermia 2006; 22: 491–506
- Liu YJ, Yang XY, Nan Q, Xiao JY, Li L. Phantom experimental study on microwave ablation with water-cooled antenna. Int J Hyperthermia 2007; 23: 381–386
- Lu YL, Nan Q, Li L, Liu YJ. Numerical study on thermal field of microwave ablation with water-cooled antenna. Int J Hyperthermia 2009; 25: 108–115
- Szwarnowski S, Sheppard RJ, Grant EH, Bleehen NM. A thermocouple for measuring temperature biological material heated by microwaves at 2.45 GHz. Br J Radiol 1980; 53: 711–715
- Jiang HB, Hao J, Li AH. Microwave phantom muscle tissue. Chinese J Biomed Eng 1992; 11: 199–203