Abstract
Purpose: To evaluate exothermic permanganate redox chemistry for utility in tumour ablation.
Materials and methods: Sodium permanganate (1–3 mL, 1 or 2 M) and glycerol (1 mL, 1 M) were injected in triplicate into a beaker using three injection orders: (1) simultaneous, (2) glycerol injection then permanganate injection, (3) permanganate injection then glycerol injection. Selected experiments were repeated with glucose, sucrose, dextrin, maltodextrin, different polysaccharides, and polyvinyl alcohol as substrates. Simultaneous injections of permanganate (0.5 and 1 mL, 2 M) and glucose (1 mL, 1 M) into explanted porcine muscle were also performed. Temperatures were recorded with a thermocouple probe or an infrared camera.
Results: With optimal conditions of 2 M permanganate and 1 M glycerol at 1 mL each, an average maximum temperature of 97.4°C was obtained for all three injection orders. When glucose and sucrose were used as the substrates, similar temperatures were achieved but at different rates. Dextrin and maltodextrin (180 g/L) led to maximum temperatures of 42.5° and 51.1°C respectively when simultaneously injected with permanganate (1 mL, 2 M). Polysaccharides and polyvinyl alcohols under the same conditions resulted in a minimal temperature increase. Intramuscular injections of glucose (0.5 mL, 1 M) and permanganate (0.5 mL, 2 M) reached an average temperature of 76.5°C at the lesion site.
Conclusions: The permanganate redox reaction is a powerful system with potential to reach tumouricidal temperatures. The reagent volumes, concentrations, injection order, and substrate choice allow a measure of control over the reaction.
Introduction
Hepatocellular carcinoma (HCC) has emerged as one of the most prevalent cancers worldwide and has continued to affect more and more people in the USA. It is estimated that more than half a million people are affected globally and the incidence has doubled from 2.6 to 5.2 per 100,000 head of population over the past two decades in the USA Citation[1], Citation[2]. It is now recognised as one of the cancers with the highest increase in both incidence and death rate over the last 10 years in the USA Citation[3]. Currently, there are several management options for HCC. Transplantation and surgical resection give the best outcomes in terms of survival rates, but are limited due to the scarcity of donor organs and selection criteria Citation[4], Citation[5]. Very few HCC patients qualify for these procedures and the inherent morbidity and risk make these procedures undesirable as treatment options. Several thermal ablation techniques have emerged as less invasive and more widely available alternatives. However, they each have limitations. Laser-induced thermal therapy, microwave, and high-intensity focused ultrasound are expensive and not readily available. Radiofrequency (RF) ablation is much more common but still costly and all thermal methods also suffer from heat-sink issues. Chemical ablation methods using ethyl alcohol or acetic acid have been supplanted in many instances by RF ablation. Chemical ablation is arguably as effective as RF and is quite inexpensive, but is subject to issues such as tracking along paths of least resistance. It is not, however, subject to heat sinks in the mechanism of action. In attempts to find a more localised, less expensive, and safer alternative to these methods, several exothermic reactions, such as acid/base neutralisation Citation[6] and alkali metals/water Citation[7–9], have recently been studied as potential thermochemical ablation reagents. Acid/base neutralisation produces sufficient heat at higher concentrations; alkali metals are difficult to handle, difficult to deliver, and dangerous throughout the entire process.
In our quest for suitable reactions, one general category we wished to explore was oxidation/reduction chemistry. Within this broad topic, one particular candidate with practical appeal is the permanganate redox system. Permanganate is a strong oxidant with established chemistry. It is widely used for industrial purposes, including (1) printed circuit board production, (2) pharmaceutical and chemical synthesis, (3) soil and groundwater remediation, (4) metal cleaning formulations, (5) acid mine drainage, (6) hydrogen sulphide odour control, and (7) as a topical disinfectant. Further, it is well known in wilderness survival folklore as a means of starting a fire from commonly available components. For example, ethylene glycol from the antifreeze solution obtained from a stranded vehicle and potassium permanganate topical disinfectant solution from a first aid kit have been used as an ignition source due to the amount of heat energy released over a short period of time Citation[10]. In the current study this system was evaluated for exothermic potential with a view towards constraints dictated by the possible end use in human tumour ablation.
Materials and methods
In vitro study
All reagents were obtained from Sigma-Aldrich Chemical Co. (St. Louis, MO) unless otherwise noted. Sodium permanganate solution of predetermined molarity was prepared by dissolving sodium permanganate monohydrate crystals in distilled water. Sodium permanganate solution and glycerol were then injected in triplicate into a clean 10 mL beaker using one of three different injection orders: (1) simultaneous injection using a coaxial injection device, (2) glycerol injection first, followed by sodium permanganate injection using a separate syringe (hereafter referred to as ‘glycerol-first’ injections), (3) sodium permanganate injection first, followed by glycerol injection using a separate syringe (hereafter referred to as ‘permanganate-first’ injections). Reaction temperature was measured by a thermocouple probe (type T MT-29/1; Physitemp Instruments, Clifton, NJ) placed at the centre of the beaker, with the tip submerged underneath the surface of the mixed solutions. Temperature recordings were made by a T-type thermocouple thermometer (Digi-Sense; Cole-Parmer, Vernon Hills, IL) at time intervals of 1 to 3 s from the onset of injections until the temperature dropped below 40°C. A series of different permanganate volumes (1, 2, and 3 mL) and concentration (1 and 2 M) were tested in order to study the effects of volume and concentration on temperature. Selected experiments were repeated with glucose, sucrose, maltodextrin (4–7 glucose unit average), and dextrin as substrates. The remaining substrates were different polysaccharides (starch, cellulose and glycogen) and polyvinyl alcohol. They were all used as 180 g/L (carbohydrates based on glucose) solutions and/or suspensions rather than expressing concentration in molarity due to their polymeric nature.
Ex vivo study
As a proof of concept, ex vivo experiments were done by performing simultaneous injections of 1 M glycerol and 2 M permanganate at 0.5 or 1 mL each into porcine muscle tissue. The temperature was recorded using a thermocouple probe placed as closely as possible to the needle tip. After the completion of injections the tissues were sectioned and lesions were examined and imaged. An infrared camera (IRI4010; IRISYS Northampton, UK) was also used as an alternative way to assess the temperature and the zone of thermal excursion at the lesion site by sectioning the tissue after completion of an injection.
Results
Glycerol versus permanganate
Using 1 M glycerol (1 mL) and 1 M permanganate (1 mL), the average maximum temperatures recorded for simultaneous injections, glycerol-first injections, and permanganate-first injections were 63.9°, 71.1°, and 59.3°C respectively. When the volume of the permanganate solution was raised to 2 mL, the average maximum temperatures for simultaneous, glycerol-first, and permanganate-first injections rose to 89.1°, 86.7°, and 70.3°C respectively. Using 3 mL of permanganate solution, the average maximum temperatures for simultaneous, glycerol-first, and permanganate-first injections were 90.6°, 90.3°, and 77.9°C respectively. Further increasing the volume of permanganate solution led to a decline in maximum temperatures (results not shown). When the volume of glycerol was raised to 2 mL, a lower average maximum temperature was obtained for all three injection orders (53.1°, 56.7°, 54.1°C). When the concentration of the permanganate solution was raised to 2 M, the average maximum temperatures for simultaneous, glycerol-first, and permanganate-first injections were 97.4°, 99.1°, and 97°C respectively. Using 3 M permanganate solution (1 mL), the reaction mixture erupted and thus temperature recording was deemed unreliable under the circumstances. Averaged recordings for simultaneous injections of permanganate and glycerol under various conditions are depicted in .
Figure 1. (A) Temperature profile of simultaneous injection of glycerol and permanganate under various conditions. (B) Temperature profile of simultaneous injection of glucose and permanganate under various conditions. (C) Temperature profile of simultaneous injection of sucrose and permanganate under various conditions. Note blunting of rise and reductions in peak temperatures compared to 1A and 1B.
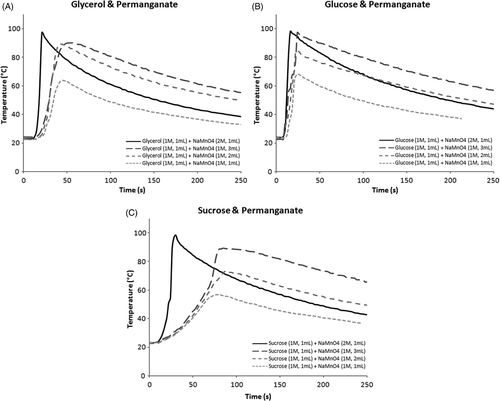
Glucose versus permanganate
Using 1 mL of glucose (1 M), the average maximum temperatures for simultaneous, glucose-first, and permanganate-first injections were 68.1°, 82.9°, and 66.3°C respectively. When the volume of the permanganate solution (1 M) was raised to 2 mL, the average maximum temperatures for simultaneous, glucose-first, and permanganate-first injections were 85.8°, 90.7°, and 88.4°C respectively. Using 3 mL of permanganate solution (1 M), the average maximum temperatures recorded for simultaneous, glucose-first, and permanganate-first injections were 97.9°, 98.3°, and 93.7°C respectively. Increasing the volume of glucose solution to 2 mL led to a decline in average maximum temperatures for all three injection orders (53.9°, 62.5°, and 52.5°C). When the concentration of the permanganate solution was raised to 2 M, the average maximum temperatures for simultaneous, glucose-first, and permanganate-first injections were 100°, 99.6°, and 94°C respectively. Averaged recordings for simultaneous injections of permanganate and glucose under various conditions are depicted in .
Sucrose versus permanganate
Using 1 mL of sucrose (1 M), the average maximum temperatures for simultaneous, sucrose-first, and permanganate-first injections were 56.8°, 58.9°, and 46.4°C respectively. Raising the volume of permanganate solution (1 M) to 2 mL, the average maximum temperatures for simultaneous, sucrose-first, and permanganate-first injections were 73.6°, 82.1°, and 64.9°C respectively. Using 3 mL of permanganate solution (1 M), the average maximum temperatures for simultaneous, sucrose-first, and permanganate-first injections were 90.1°, 85.1°, and 74.6°C respectively. Increasing the volume of sucrose solution to 2 mL also led to a decline in average temperature increase for all three injection orders (45.9°, 51°, 44.6°C). When the concentration of the sucrose solution (1 mL) was raised to 2 M, the average maximum temperatures for simultaneous, sucrose-first, and permanganate-first injections were 100.2°, 99.8°, 100°C respectively. Averaged recordings for simultaneous injections of permanganate and sucrose under various conditions are depicted in .
A summary of the results for glycerol, glucose, and sucrose, including the average temperature increases (maximum temperature−basal temperature) under various conditions, is depicted in .
Table I. Temperature increases and peak temperatures with different stoichiometries using glycerol, glucose, and sucrose as substrates.
Oligosaccharides
Simultaneous injections of dextrin (180 g/L) and permanganate (1 M, 1 mL) led to an average maximum temperature of 51.1°C. Under the same condition except with maltodextrin as the substrate instead, an average maximum temperature of 42.5°C was observed. The peak of the temperature profile was also reached at a much slower rate for both dextrin and maltodextrin when compared to that of glycerol, glucose and sucrose.
Polysaccharides and polyvinyl alcohol versus permanganate
Multiple conditions for these substrates with permanganate were tested based on the best outcomes using glycerol, but none of them resulted in an increase of more than 8°C from room temperature.
Ex vivo injections
Simultaneous intramuscular injections of glucose (1 M, 0.5 mL) and permanganate (2 M, 0.5 mL) led to an average maximum temperature of 76.5°C. shows the temperature recordings for three separate intramuscular injections. Tissue staining by permanganate (purple) and the presence of manganese dioxide (dark brown) obscured evaluation of lesions (). In order to appreciate the temperature gradient at the lesion site, an infrared image () of the lesion site was taken after simultaneous injections of permanganate (2 M, 1 mL) and glucose (1 M, 1 mL) and sectioning the tissues after completion of the injection. A warm dime (1.79 cm diameter) was used as a size reference in the same focal plane. A maximum temperature of 58.2°C in this ex vivo sample was recorded at the centre of the lesion (saturated region). However, the actual maximum temperature might be greater if not for the time delay and heat dissipation upon sectioning the lesion site wide open.
Figure 2. (A) Temperature data from simultaneous ex vivo injections of glucose and permanganate into muscle tissue using only 500 microlitres each. (B) Gross specimen bisected after simultaneous injection of glucose and permanganate into muscle tissue under conditions listed for . Staining from permanganate products is apparent. (C) Infrared pseudo color image from sample in , simultaneous injection of glucose and permanganate with warmed dime for a reference. Temperature range 19.8–58.2°C. Note relatively large area still off scale despite delay for tissue sectioning prior to imaging.
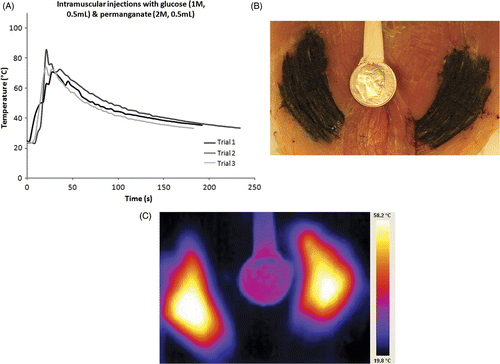
Discussion
Redox chemistry has tremendous potential for energy release, hence its use in propulsion systems, ballistics, and explosives. One example, briefly considered and discarded, is the thermite reaction. Such chemistry usually requires a very high initiation temperature and once started, goes to completion in a self-sustaining manner. These and other factors made us look elsewhere.
Permanganate, particularly in solution, is relatively safe to handle compared to alkali metals as it is stable to air and moisture. This stands in marked contrast to elemental sodium and potassium which are pyrophoric and can spontaneously react with the moisture in the air, producing sparks or worse if there is no inert atmosphere provided Citation[11]. Having an ignition source may pose as a threat to patients in an operative room where high concentrations of oxygen are commonly present Citation[12]. Indeed, prior to the replacement of ether by modern fluorinated inhalational anaesthetics, fire in the operating suite was a much greater danger than today. In addition, potentially fatal intravascular gas embolism may be of concern to patients if a large amount of hydrogen gas produced by alkaline metals and water is introduced into the blood Citation[13] via a relatively vascular organ such as the liver. Permanganate is more efficient than acid/base neutralisation in the sense that low-molarity permanganate solution is capable of achieving a high maximum temperature (above 80°C) produced by an equivalent volume of acid and base at a much higher molarity in vitro (). Another potential advantage of using the permanganate redox system is the flexibility to manipulate reaction kinetics by using different substrates.
Figure 3. Comparison of redox and neutralization reactions. Note that the exotherm from 1 M acid and base is near baseline and peak temperature from 15 M solutions is intermediate between 1 and 2 M redox values.
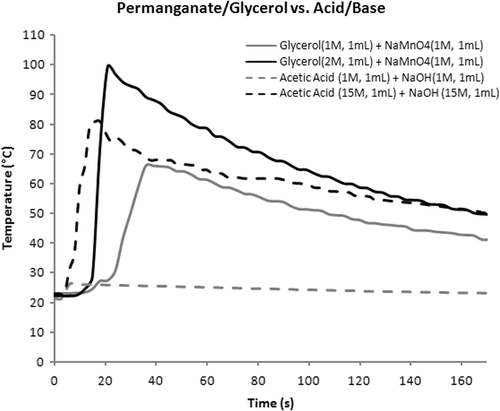
Choice of reagents
The choice of reagents for the current study reflects several considerations. First and foremost, systemic exposure to any of the reagents or their subsequent reaction products must be considered if a reaction were ever to see clinical application. In this regard, the ethylene glycol/potassium permanganate system is far from ideal. The renal toxicity of oxalic acid, the metabolite of ethylene glycol Citation[14] is well known as a consequence of antifreeze poisoning, and so we examined other substrates. One compound, glycerol, immediately came to attention for several reasons. It is a naturally occurring substance which forms the molecular backbone of triglycerides and phosphoglycerides which are essential components of cell membranes and also sphingolipids. Glycerol has three hydroxyl groups available as substrates for exothermic oxidation, two of which are intrinsically more reactive primary alcohols. It is also miscible in all proportions with water, has very little toxicity, is clear, colourless, inexpensive, of adequately low viscosity, and listed by the FDA as ‘generally recognized as safe’. Toxicity nevertheless is a function of the dose and route, and in fact glycerol has been used in higher concentrations locally for neurolysis and orally as a treatment for high intraocular and intracranial pressure Citation[15], Citation[16]. This could by itself be useful in tumour ablation if any unreacted product remained due to local cytotoxic effects, but has not to our knowledge been tested. Accurate assessment of systemic exposure becomes more complicated, however. The reaction products from glycerol cover a spectrum of oxidation states and have been characterised previously only in general terms. Also to be considered are that the products vary depending on the conditions of the reaction itself. With excess oxygen present and a reaction driven to completion, oxalic acid could potentially be formed. This is the same substance responsible for renal failure from ethylene glycol discussed above. However, the conditions envisioned for tumour therapy do not favour this pathway as extreme conditions are required, insufficient oxygen is available, and alternate pathways for reaction exist. Among the alternative products are tartronic acid and glyceric acid (). These would of themselves be interesting as the carboxylic acid moieties could lend a chemical ablation component if they remained in the acid form much the way acetic acid has been used clinically for tumour ablation. Finally, if the chemistry is efficient enough, low systemic doses would not be expected to cause a metabolic acidosis.
Figure 4. Two of the possible incomplete oxidation products from glycerol oxidation. Note that in each case carboxylic acids may be formed.
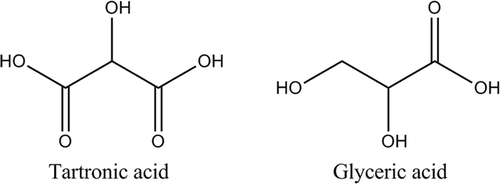
Many other compounds function as substrates for oxidation, as shown by the data obtained. These were of interest because they are also readily available and found both in the diet and in the body, particularly glucose. The ratio of primary to secondary alcohols in glucose is inverted compared to glycerol. Interestingly, under the conditions of the experiments, this inherent structural difference in reactivity does not appear to have resulted in a lower maximum temperature for glucose. This may be due in part to the fact that more hydroxyl groups are present in one molecule of glucose compared to one molecule of glycerol (i.e. not a strictly straightforward comparison) but the situation is not so clear cut. If this were the case, one might predict that sucrose would have a similar temperature increase instead of the lower increase and slower rise to peak temperature that was observed. Regardless of the underlying mechanism responsible for the different temperature profiles, it may be beneficial to have the flexibility to manipulate reaction kinetics and temperature profile by using different substrates. While fast kinetics may help alleviate the heat sink problem, slow kinetics may provide more safety by not heating the catheter and thereby the normal tissue along the injection path during the delivery. Future in vivo study will help us elucidate the benefits and disadvantages of different reaction kinetics in terms of tumouricidal effect. Perhaps, in the future, clinicians will be able to choose from a variety of substrates and tailor the ablation therapy to each patient's situation.
Structural effects clearly do have consequences for the exotherms observed, however. Temperatures obtained from the more complex polysaccharides were disappointingly low. In particular, polyvinyl alcohol, a polymer used for embolisation particles among other things, seemed relatively unreactive. This may be due to a lack of accessibility of substrate hydroxyl groups in complex 3D molecular structures but this is speculative. Surface area, which is a function of particle size, clearly would be expected to play a role for such a material.
Among factors to consider for the permanganate anion itself, sodium was chosen as the cation for three reasons. First, systemic exposure to excess potassium could potentially cause cardiac rhythm, whereas a transient exposure to excess sodium would not cause this issue. Second, the solubility of the sodium salt is significantly greater than the potassium salt (40% solutions are available versus 3–4%). This would allow for a higher concentration to be employed, which in turn would imply that a smaller volume of reagent would need to be injected for the same overall amount of permanganate. Given that the atomic mass of sodium is 23 a.m.u. versus 39 a.m.u. for potassium, it is also apparent that on a mass basis the sodium salt is more efficient than the potassium salt.
Weighed against these considerations is the toxicity of the manganese-containing by-products. Generation of poorly soluble manganese dioxide is quite likely and possibly the reduction of permanganate could lead to an alkaline environment. The alkaline environment could be beneficial for ablation but as mentioned earlier the manganese exposure and burden must be considered. One of the products, manganese dioxide, is itself a mild, specific oxidising agent. The toxicity reported in the literature from acute exposure to permanganate is generally the result of accidental or deliberate oral ingestion of large quantities. It therefore takes the form of local, superficial mucositis and airway oedema Citation[17–20] as these are the tissues directly exposed. Cases of neurological symptoms have been noted as well, generally from chronic inhalational exposure in miners, and the symptoms may take years to develop. A pseudo-Parkinsonism presentation has been noted after sufficient exposure. While no data exist on the issue, it is noteworthy that in general, the neurological symptoms have taken many years longer to develop than the natural life expectancy of this patient population (cirrhotics, especially so in cirrhotics with hepatoma) regardless of treatment success or transplant. Furthermore, the acute toxicity studies involve the permanganate ion itself. Given the extraordinary reactivity of permanganate and an abundance of available substrate, it would appear likely that permanganate would be quickly consumed and therefore unable to cause such symptoms.
Due to the many possible substrates and products, it is not yet clear what chemical species exactly are involved in these cases, or what the final oxidation state of the manganese ion is. Other confounding factors also exist. Given the differences in oxidation states and routes of exposure, it is difficult to speculate on the systemic toxicity from an acute exposure to manganese such as might be seen in local tumour hyperthermia treatment. Likewise, the degree of chronic exposure would be unknown due to the fact that successfully ablated tissues would be devascularised.
Thermodynamics
The thermodynamics in these experiments are complex for a number of reasons. The data from represent in essence thermometric titrations in which no further heat is evolved beyond a 2 : 1 ratio of permanganate to glycerol. This occurred at a practical upper temperature limit of about 100°C, and beyond this a violent exotherm was observed. Energy losses therefore would occur from kinetic energy, some degree of vaporisation, and incomplete reaction. With this in mind we chose to optimise the conditions for minimising the contributed thermal mass of the reagents themselves by optimising the concentrations. As can be seen in , with the highest concentrations of permanganate and glycerol tested, the lag time essentially disappeared. It is not yet known if this energy release profile would be the most desirable with respect to tissue ablation.
The precise mechanism of the reaction, the exact products of the reaction, and their relative distribution are unknown. These issues make theoretical calculations about the potential energy challenging at the very least but some observations and approximations can be made. It would seem likely that at a minimum the primary alcohols are oxidised to aldehydes or more likely to carboxylic acids, but it is not known whether complexation of products with reactants occurs under these conditions and to what extent this may occur. Mechanistic data are sparse in this area, and tend to assume that oxidation is carried to completion and the product is CO2. This is not likely the case as discussed below. It is also not clear whether the reaction products undergo carbon-carbon bond cleavage, or whether the secondary alcohol in glycerol acts as a substrate as well. In a simple side-by-side comparison, a primary alcohol will normally be oxidised more quickly but at the same time given the structure of glycerol as three adjacent hydroxyl groups on a carbon chain, one could speculate on a possible proximity effect that would provide a kinetic advantage favouring oxidation of the secondary alcohol after one of the primary hydroxyls has undergone reaction.
The heats of combustion for glycerol, glucose, and sucrose are −1654.3 kJ mol−1, −2805 kJ mol−1, and −6014 kJ mol−1, respectively. However, as observed by comparing , there is little or no correlation between different heats of combustion and peak temperature observed during reaction. For glycerol, glucose, and sucrose, the heat of combustion is approximately 0.5 kJ mol−1 of carbon in the substrate. For reactions reaching 100°C approximately 20% of the heat expected from complete combustion is liberated during reaction. The data indicate that substrate is therefore unlikely to be completely consumed. As the amount of oxidant is increased to consume more fuel, so is the mass to be heated increase. At some point it appears these interactions may cancel each other out.
In any calculations for comparison purposes, the extent to which heat transfer occurs (i.e. is the system adiabatic?) must be considered. In general, the reactions proceed to completion on time scales short enough to result in negligible heat losses to the surrounding air relative to the total heat generated in the mixture. More specifically, assuming half the vessel's Pyrex walls are held constant at 100°C (the worst case scenario) for the ∼10 s reaction time typically observed, only ∼2.5% of the heat generated is lost to the surrounding air. However, in reactions proceeding far slower such as the maltodextrin reaction (a more complex substrate, discussed below), similar calculations show a more significant heat loss of ∼20% to the surrounding air. Nevertheless, within the time frame for the more reactive substrates this does not seem likely to play a significant role.
Due to the small volumes of solution used during reaction, the rate of conductive heat transfer from the solution to the beaker itself cannot be neglected. More specifically, assuming half the Pyrex vessel is heated to maximum solution temperature, ∼20% of the heat generated from reaction is transferred to the vessel walls. As a result, the peak temperatures reported could be reduced by the thermal capacitance of the beaker in close proximity to the solution itself. However, the relative behaviours of different mixtures and the conclusions are unaffected by the heat loss experienced from the solution to the surroundings given the standardised methods for the experiments.
The effect of injection order
It was of interest to assess the order of injection to see if there were any effects on the amount of heat released based on how the reaction was performed. As can be seen in , it is clear that, in general, the highest maximum temperatures were achieved with either substrates present or injected first. This observation, along with the fact that higher maximum temperatures were obtained with higher permanganate-to-substrate ratios suggests that permanganate served as the limiting reagent.
The effect of different substrates
To study the effect of different substrates on the reaction temperature, we deliberately selected substrates of increasing structural complexity. The progression of structural complexity of the substrates used in our experiments is depicted in . We used 180 g/L rather than molarity as the basis for the relevant calculations for starch, dextrose, and maltodextrose as it represents the molecular weight of glucose. The polymers, which were composed of glucose but of mixed amounts and solubility, were therefore compared on a mass basis. As can be seen in , using 1 M permanganate, the rank of temperature increase achieved by different substrates from the highest to the lowest is glucose, glycerol, sucrose, dextrin and maltodextrin. This observation is in line with the observation that larger, more complex substrates tend to yield lower maximum temperatures. However, at least for the smaller substrates, the differences in maximum temperature and kinetics among the substrates were less pronounced when the concentration of the permanganate solution was increased to 2 M ().
Limitations and future directions
The experiments in this initial stage study provided us with a basic understanding of the exothermic potential and relative reactivity of different candidate substrates without the added complexity of tissue heterogeneity in ex vivo study. Carefully designed ex vivo and in vivo experiments will be necessary in order to elucidate conditions required to achieve different lesion sizes and maximum temperatures in living tissues. Volume calculations, tissue distribution in appropriate model systems, and standardised probe placement will be key to such studies for consistency. Such information will help to optimise the conditions required to develop an effective thermochemical ablation therapy. Now that the thermal potential is established, histology will also be of interest to show the lesions in more detail.
Systemic exposure of permanganate and its reaction products must also be thoroughly evaluated and limited to ensure clinical safety and a satisfactory therapeutic index. Clinicians will have to utilise imaging guidance to deliver the chemical reagents into tumours. In the future it may also be worthwhile to evaluate the performance of thermochemical therapy in combination with transarterial chemoembolisation (TACE), which can eliminate the major blood supply to hepatocellular carcinoma as one mechanism of action Citation[3] or with thermal sensitisers, for example.
It will be of interest to also investigate the minimum conditions and exposure required to kill tumour cells in a cell culture of permanganate.
Conclusions
The permanganate redox reaction is a powerful system clearly capable of reaching tumouricidal temperatures. The reagent volumes, concentrations, injection order, and choice of substrate allow some flexibility with a measure of control over the maximum temperatures and the time to reach the maximum temperatures.
Acknowledgements
The authors would like to thank Rina Farah for her technical assistance, Dr Paul Iaizzo for providing the explanted porcine tissues and the use of the infrared camera, and from the Department of Chemical Engineering and Materials Sciences we thank Dr Lanny Schmidt and his student Joshua Colby and Brian Michael for assistance with evaluating the thermodynamics.
Declaration of interest: This research was supported in part by a grant from the Ernest J. Ring Faculty Development Award from the society of Interventional Radiology Foundation.
References
- Seeff LB. Introduction: The burden of hepatocellular carcinoma. Gastroenterology 2004; 127: S1–S4
- El-Serag HB. Hepatocellular carcinoma: Recent trends in the United States. Gastroenterology 2004; 127: S27–S34
- Marrero JA, Pelletier S. Hepatocellular carcinoma. Clin Liver Dis 2006; 10: 339–351
- Cormier JN, Thomas KT, Chari RS, Pinson CW. Management of hepatocellular carcinoma. J Gastrointest Surg 2006; 10: 761–780
- Schwarz RE, Smith DD. Trends in local therapy for hepatocellular carcinoma and survival outcomes in the US population. Am J Surg 2008; 195: 829–836
- Misselt AJ, Edelman TL, Choi JH, Bischof JC, Cressman ENK, A hydrophobic gel phantom for study of thermochemical ablation: Initial results using a weak acid and weak base. J Vasc Interv Radiol 2009;20:1352–1358.
- Rao W, Liu J. Tumor thermal ablation therapy using alkali metals as powerful self-heating seeds. Min Invas Ther 2008; 17: 43–49
- Rao W, Liu J. Anti-tumor effect of sodium-induced thermochemical ablation therapy. Int J Hyperthermia 2008; 24: 675–681
- Rao W, Liu J. Injectable liquid alkali alloy-based tumor thermal ablation therapy. Min Invas Ther 2009; 18: 30–35
- Shakhashiri, BZ. Chemical demonstrations, Vol. 1. Madison, WI: The University of Wisconsin Press, 1983, p. 83.
- Urben P. Bretherick's Handbook of Reactive Chemical Hazards, 7th. Elsevier, Oxford 2007; 1909
- Thomas GJ. Fire and explosion hazards with flammable anesthetics and their control. J Natl Med Assoc 1960; 52: 397–403
- Fukaya E, Hopf HW. HBO and gas embolism. Neurol Res 2007; 29: 142–145
- Jacobsen D, McMartin KE. Methanol and ethylene glycol poisoning. Mechanisms of toxicity, clinical course, diagnosis and treatment. Med Toxicol 1986; 1: 309–334
- Sindou M, Tatli M. Treatment of trigeminal neuralgia with glycerol injection at the gasserian ganglion. Neurochirurgie 2009; 55: 211–212
- Thornit DN, Sander B, la Cour M, Lund-Andersen H. The effects of peroral glycerol on plasma osmolarity in diabetic patients and healthy individuals. Basic Clin Pharmacol Toxicol 2009; 105: 289–293
- Middleton SJ, Jacyna M, McClaren D, Robinson R, Thomas HC. Haemorrhagic pancreatitis–A cause of death in severe potassium permanganate poisoning. Postgrad Med J 1990; 66: 657–658
- Young RJ, Critchley JA, Young KK, Freebairn RC, Reynolds AP, Lolin YI. Fatal acute hepatorenal failure following potassium permanganate ingestion. Hum Exp Toxicol 1996; 15: 259–261
- Ong KL, Tan TH, Cheung WL. Potassium permanganate poisoning–A rare cause of fatal self poisoning. J Accid Emerg Med 1997; 14: 43–45
- Johnson TB, Cassidy DD. Unintentional ingestion of potassium permanganate. Pediatr Emerg Care 2004; 20: 185–187