Abstract
Purpose: Thermosensitive liposomes provide a mechanism for triggering the local release of anticancer drugs, but this technology requires precise temperature control in targeted regions with minimal heating of surrounding tissue. The objective of this study was to evaluate the feasibility of using MRI-controlled focused ultrasound (FUS) and thermosensitive liposomes to achieve thermally mediated localised drug delivery in vivo.
Materials and methods: Results are reported from ten rabbits, where a FUS beam was scanned in a circular trajectory to heat 10–15 mm diameter regions in normal thigh to 43°C for 20–30 min. MRI thermometry was used for closed-loop feedback control to achieve temporally and spatially uniform heating. Lyso-thermosensitive liposomal doxorubicin was infused intravenously during hyperthermia. Unabsorbed liposomes were flushed from the vasculature by saline perfusion 2 h later, and tissue samples were harvested from heated and unheated thigh regions. The fluorescence intensity of the homogenised samples was used to calculate the concentration of doxorubicin in tissue.
Results: Closed-loop control of FUS heating using MRI thermometry achieved temperature distributions with mean, T90 and T10 of 42.9°C, 41.0°C and 44.8°C, respectively, over a period of 20 min. Doxorubicin concentrations were significantly higher in tissues sampled from heated than unheated regions of normal thigh muscle (8.3 versus 0.5 ng/mg, mean per-animal difference = 7.8 ng/mg, P < 0.05, Wilcoxon matched pairs signed rank test).
Conclusions: The results show the potential of MRI-controlled focused ultrasound hyperthermia for enhanced local drug delivery with temperature-sensitive drug carriers.
Introduction
Hyperthermia has been shown to enhance extravasation of drug carriers in solid tumours, potentially overcoming barriers to drug delivery presented by the tumour microenvironment Citation[1]. The use of temperature-sensitive drug carriers provides a mechanism for triggering the rapid release of high concentrations of active drug in a targeted region Citation[1–5]. This localised therapy depends on the stability and heat-triggered release of the contents of drug carriers, and also the ability to accurately maintain controlled temperature elevations in targeted regions with minimal temperature increase in surrounding tissue.
Most studies with thermosensitive drug carriers in preclinical models have either applied regional, superficial heating with water baths Citation[3], Citation[6–11] and microwave electromagnetic applicators Citation[12], Citation[13], or localised heating of deep targets with invasive catheters Citation[14–16]. Recently, pulsed high-intensity focused ultrasound has been used to potentiate drug release from thermosensitive liposomes Citation[17–19]. Temperature measurement in all of these studies has been limited to point measurements made by invasive thermocouple or optical probes.
Millimetre-sized focal regions several cm beneath the skin can be heated non-invasively with minimal energy deposition in the intervening tissue using externally focused ultrasound transducers Citation[20]. By mechanically Citation[21], Citation[22] or electronically Citation[23] scanning the ultrasound focus, larger target regions can be heated. Early mechanically scanned systems Citation[22] overcame spatially varying energy absorption and blood flow in tissue with rapid scanning of the focal point Citation[24], Citation[25] and multi-point control based on arrays of invasive thermocouple probes Citation[26], Citation[27]. Promising clinical temperature distributions were achieved in a number of anatomical sites Citation[28].
Since then it has been shown that spatial temperature distributions can be non-invasively monitored in most tissues using MRI thermometry based on the proton resonance frequency shift Citation[29], Citation[30]. Stable measurements can be made during ultrasound heating Citation[31], and have been used clinically to guide point-by-point coagulation of tumours Citation[32–35]. MRI temperature measurements can also be used to regulate temperature elevations in a feedback control loop Citation[36–39]. This form of adaptive, closed-loop therapy has been applied for tumour coagulation using intracavitary Citation[40], Citation[41] and externally focused Citation[42] ultrasound transducers, lasers Citation[43], and percutaneous radiofrequency applicators Citation[44]. Non-invasive radiofrequency hyperthermia under MRI temperature control is under development Citation[45], but poor steering and focusing abilities limit target temperature elevations that can be safely achieved. MRI-controlled hyperthermia using scanned ultrasound beams is challenging to implement due to image artefacts caused by device motion and the inability to use conventional motors in the magnet bore. Slow hydraulic scanning of a single element transducer has been shown to achieve spatially uniform heating across a 20 mm diameter region under MRI control, but with wide periodic temporal variations in temperature elevation Citation[46], Citation[47]. With a sophisticated phased-array system capable of rapid electronic displacement of the focal point, precise spatial and temporal control has been achieved Citation[48]. An MRI-compatible focused ultrasound system developed recently Citation[49] enables mechanical scanning of an ultrasound transducer at circular scan rates required to achieve uniform temperature elevations in tissue (>0.2 Hz) Citation[25], taking advantage of simple multi-point control schemes previously used under thermocouple control Citation[26], Citation[27].
The objective of this study was to investigate the use of MRI-controlled focused ultrasound hyperthermia to achieve localised drug release in vivo. For this preliminary feasibility study, a rabbit model was used. Normal thigh muscle was heated using a mechanically scanned focused ultrasound system under MRI temperature control, and temperature-sensitive liposomes were administered during heating. The ability to maintain spatially and temporally uniform hyperthermia in 10–15 mm diameter regions was evaluated, as well as the ability to achieve localised accumulation of released drug.
Materials and methods
MRI-guided focused ultrasound system
Non-invasive heating of rabbit thigh was achieved by continuous sonication using a spherically focused, air-backed piezoceramic ultrasound transducer (f0 = 0.536 MHz, f-number = 2, OD = 5 cm). To achieve spatially uniform temperature distributions in the thigh under MRI temperature feedback control, the transducer was mounted to a custom-made MRI-compatible three-axis positioning system Citation[49] programmed to move along a 1–2 cm diameter circular trajectory at a speed of 1 revolution per second, for simultaneous ultrasound heating and MRI thermometry in the plane of the ultrasound focus. The positioning system was constructed from non-magnetic components, piezoceramic actuators and optical encoders, and was capable of simultaneous transducer motion and MR imaging with minimal mutual interference. The system was able to achieve a spatial positioning precision of approximately 0.1 mm with linear speeds up to 50 mm/s per axis over a total travel of 4 cm in each direction.
The transducer diameter (5 cm) and focal length (10 cm) were chosen to reduce magnetic susceptibility artifacts caused by the motion of the transducer during heating. Driving the transducer at 2.787 MHz (fifth harmonic) resulted in a focal spot with lateral and axial FWHM of 1 and 15 mm, respectively. This spans the length of most preclinical tumours of interest with minimal energy deposition beyond the focal point, but allows for modulation of heating in the transverse plane. The transducer was driven by an arbitrary waveform generator (33250A, Agilent, Santa Clara, CA) and radiofrequency power amplifier (NP2912, NP Technologies, Newbury Park, CA). The electrical power was measured with a power meter (438A, Hewlett Packard, Palo Alto, CA) connected to the forward and reverse signal of a dual directional coupler (C1373, Werlatone, Brewster, NY). The transducer's electrical impedance was matched to the output impedance of the amplifier (50 Ω) with a custom-made passive matching circuit. At the driving frequency, the emitted acoustic power was approximately 70% of the applied electrical power (forward minus reflected) when measured with a radiation force balance technique using a laboratory balance (AE200, Mettler-Toledo, Columbus, OH). This efficiency was used to calculate the electric power required to achieve a desired acoustic power during sonication.
Animals
The experiments described in this study were approved by the institutional Animal Care Committee at Sunnybrook Health Sciences Centre. New Zealand White rabbits (3–4 kg, n = 10) were anaesthetised with an intramuscular injection of 50 mg/kg ketamine and 10 mg/kg xylazine administered away from the planned sonication site, before intubation and maintenance under 2–4% isoflurane. To prevent motion, an additional 1 mL injection of 3 parts ketamine/xylazine diluted with 7 parts saline was administered into a cannulated ear vein approximately 5 min prior to hyperthermia.
For this study a rabbit model was selected in order to provide a large enough tissue mass for targeting, and to reduce the effect of prolonged localised heating on core body temperature that might be seen in smaller animal models. Anaesthetised rabbits had their thighs shaved and depilated, and were placed on a stage above the focused ultrasound system in the lateral decubitus position, as illustrated in . Degassed water was used to couple the target thigh through an 80 mm square window of 25 µm polyimide film into the reservoir of degassed water in which the transducer was submerged. A square (87 mm inner side length) custom-made single-loop RF receive coil with a square opening in the middle (85 mm inner side length) was designed to fit underneath the animal around the window for optimal SNR in the heated region. The thigh was positioned such that the ultrasound beam made approximately normal incidence with the skin through the coil. The transducer position was adjusted to locate the focus at a depth of 1–2 cm from the skin, at the interface between the biceps femoris and the semimembranosus or semitendinosus muscles of the thigh. Once the correct focal depth was achieved, the transducer was scanned along a circular trajectory transverse to the ultrasound beam direction. The diameter of the circular trajectory was varied between 1 and 1.5 cm across experiments based on the available acoustic window. To prevent undesired tissue heating due to acoustic reflections and propagation into the contralateral thigh, a saline bag and polyurethane rubber acoustic absorber (AptFlex F28, Precision Acoustics, Dorchester, UK) were placed between the rabbit's thighs, coupled with ultrasound gel. Thermosensitive liposomal doxorubicin and MR contrast agents were injected into a cannulated ear vein.
Figure 1. Experimental setup for in vivo MRI-controlled focused ultrasound hyperthermia. (a) Axial depiction of rabbit in lateral decubitus position. (b) Axial T2-weighted image along beam path, indicating the coronal plane used for MRI thermometry and the acoustic field path at the boundaries of the heating trajectory. (c) Coronal T2-weighted image taken through the plane indicated in (b), with eight control regions shown on the periphery of the 10mm diameter target region. (b) and (c) both have a 120 mm field of view.
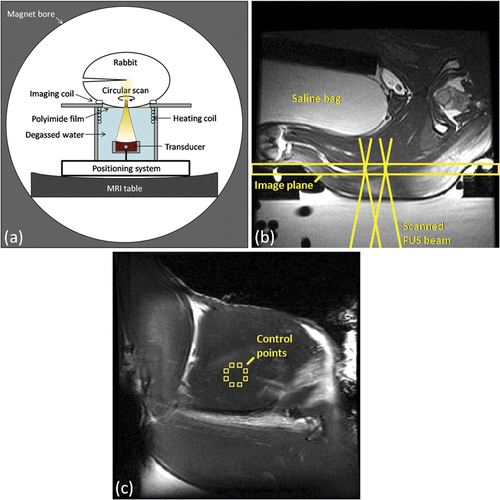
The rabbit's body temperature was monitored by a fibre-optic temperature probe inserted into the rectum (3100, Luxtron, Santa Clara, CA), and maintained by regulating the temperature of a hot water blanket covering the animal (Gaymar T/Pump Model TP-500, Orchard Park, NY). The temperature of the degassed water reservoir below was maintained by pumping warm water through a heat exchanger made from a coil of polyurethane tubing. These temperatures were monitored with additional fibre-optic probes at the skin/blanket interface and in the water bath. Oxygen saturation (SpO2) and heart rate were monitored by a veterinary pulse oximeter (8600V, Nonin Medical, Plymouth, MN).
In early experiments, discrete movements of 2–3 mm were likely the result of bone heating caused by transmission of ultrasound through the animal to the contralateral thigh, as well as acoustic reflection at skin/air interfaces. In subsequent experiments motion was avoided by careful positioning to avoid bone, and by an intramuscular injection of local analgesic (2% Lidocaine HCl Injection USP, Alveda Pharmaceuticals, Toronto, ON) immediately before hyperthermia, administered upstream from the planned sonication site.
In one of the treated animals, oxygen saturation and heart rate dropped suddenly from 99% to 50% and from 150 to 100 beats per min shortly after drug injection during heating, and required resuscitation before post-treatment imaging. These symptoms are consistent with liposome-related anaphylactoid reactions reported previously in dogs Citation[13], pigs Citation[50] and some humans Citation[51], and could likely be prevented by premedication with steroids and antihistamines Citation[13], Citation[50].
MR imaging
Experiments were performed with the positioning system placed in the bore of a clinical 1.5T MR imager (Signa, GE Medical Systems, Milwaukee, WI). Co-registration of the ultrasound focus in the MRI coordinate system was accomplished by first heating a gel phantom and locating the region of temperature increase with MRI thermometry.
Next, the animal was positioned on the FUS system in the magnet, and axial and coronal anatomical T1-weighted (fast spin echo (FSE-XL), echo time (TE) = 15 ms, repetition time (TR) = 500 ms, echo train length (ETL) = 2, bandwidth (BW) = 15.63 kHz, number of excitations (NEX) = 3, 256 × 128 matrix, field of view (FOV) = 12 cm, slice thickness = 3 mm, no phase wrap) images were acquired to locate the target region in the thigh before heating ( and ). These images were re-acquired after heating to evaluate changes in tissue perfusion and/or tissue damage caused by ultrasound heating. The post-sonication T1-weighted images were acquired before and continuously for 5 min after administration of a 2-s bolus ear vein injection of MR contrast agent (0.2 mmol/kg, Omniscan, GE Healthcare) followed by a 1 mL injection of saline.
During mechanical scanning of the ultrasound transducer, fast spoiled gradient-echo images (fast spoiled gradient recalled (FSPGR), TE = 10 ms, TR = 38.6 ms, 30° flip angle, BW = 31.25 kHz, 128 × 128 matrix, FOV = 12 cm, slice = 5 mm, NEX = 1, acquisition time = 5 s) were continuously acquired in a coronal plane through the acoustic focus to measure the spatial heating pattern in the target region. A real-time image acquisition interface Citation[52], Citation[53] was used to transfer raw k-space data to a control computer immediately after acquisition for image reconstruction and processing of temperature maps using software written in MATLAB (Mathworks, Natick, MA). Temperature maps were calculated by performing a complex phase subtraction between treatment images and the average of five initial baseline images acquired prior to heating, scaling the result to a relative temperature using a proton resonance frequency shift coefficient of −0.01 ppm/°C Citation[54], and then adding the baseline temperature measured by a fibre-optic temperature probe in the rectum. Unheated regions of the FSPGR images were identified to measure phase drift over the experiment duration.
Accurate measurement of temperature from the phase difference between MR images acquired during mechanical scanning of an ultrasound transducer is a significant technical challenge. A moving object in the bore of the MRI causes a local change in the main magnetic field related to its magnetic susceptibility Citation[55] violating the assumption of static field inhomogeneities in the PRF shift subtraction method Citation[30]. The circular scan trajectory of the ultrasound transducer was found to cause periodic phase shifts, whose frequency was related to the speed of rotation and whose amplitude increased with proximity of the transducer to the imaging plane. As temperatures were expected to be relatively stable during treatment, the resulting temperature artefacts were mitigated by temporal averaging. Tests of several window lengths indicated that by averaging over a 30-s sliding window, the standard deviation of temperatures measured during transducer motion could be matched to that seen without motion. This window was applied during treatment to temperature images used for feedback control, as well as for images used to calculate thermal dose.
Closed-loop feedback control
Previous studies Citation[26], Citation[48] suggest that single-point fixed-gain proportional-integral (PI) control applied at multiple points along a rapidly scanned peripheral energy deposition trajectory can be used to achieve uniform temperatures in a targeted region with sharp gradients at the boundaries and a reasonable degree of steady-state temperature accuracy, robust to spatial and temporal changes in blood flow.
In this study, spatial heating was controlled by eight independent PI feedback controllers along the scanned heating trajectory, rapidly switching the applied power as the transducer scanned along the circular trajectory, and updating the controller outputs after each image acquisition according to the following equation:
For temperature image n, the temperature T in each of the eight pre-defined 3 × 3 pixel control regions was compared with the desired target temperature Tgoal to calculate an error term. This error term was scaled by proportional gain KP, while the integral of the error over all previous images was scaled by integral gain KI to specify a new acoustic power P to be delivered by the transducer as the focus crossed that region. When the transducer position coincided with one of the control locations during scanning, the function generator output amplitude was adjusted to achieve the acoustic power determined by the control algorithm. This modulation of function generator amplitude occurred at a frequency of 8 Hz during circular scanning at 1 Hz and temperature imaging at 0.2 Hz. Applied power was limited to 16 acoustic watts, corresponding to a measured peak negative pressure of 2.5 MPa at the focus, safely below the predicted threshold for cavitation to occur at this frequency (0.6 MPa + 5.3 MPa MHz−1, or 15.37 MPa at 2.787 MHz) Citation[56].
During gel and in vivo heating experiments, eight equally spaced independent control points were selected around the target trajectory with the controller gains identified in simulations, as described below. Circular transducer motion and then continuous FSPGR image acquisition were initiated. After five reference FSPGR images were acquired and averaged, twelve additional images were acquired to determine the standard deviation of temperature measurements before ultrasound heating was initiated under temperature feedback control with the positioner rapidly scanning the focus along a circular trajectory. In early experiments, periodic temperature artefacts and proportional-integral control of voltage instead of power (thus varying with the temperature error squared) caused excessive temperature fluctuations in the heated region. Averaging control point temperatures over a 30 s sliding window and using a less aggressive power-based controller (KP = 4.5, KI = 0.03) that tracked an exponential rather than step input function, gave accurate temperature control with steady-state oscillations of less than 1°C and no overshoot.
To evaluate the temporal and spatial uniformity of heating, the mean, T90 and T10 temperatures were measured at each time-point across circular regions of interest (ROIs) matching the intended target diameter. The steady-state mean and standard deviation of each measure were calculated over all images where Tgoal was >95% of the final target temperature elevation.
Numerical simulations
Numerical simulations of scanned ultrasound heating were used to identify appropriate controller gain values as in previous simulation studies of scanned focused ultrasound heating Citation[25], Citation[26]. Briefly, a multilayer transmission model of the acoustic field pattern Citation[57], Citation[58] with the focal plane at 3 cm into a 5 × 5 × 7 cm block of homogeneous, non-perfused tissue was shifted off-centre by the scan radius and moved in a circular path around the centre using affine transformations and linear interpolation. At each 0.01 s time-step, the three-dimensional temperature distribution was recalculated by the Pennes bio-heat transfer equation Citation[59] using a previously described finite-difference time-domain implementation Citation[58]. The simulations assumed a tissue density of 1000 kg m−3, specific heat capacity of 3700 J kg−1°C−1, thermal conductivity of 0.5 W m−1°C−1, amplitude attenuation coefficient of 4.1 Np m−1 MHz−1, and speed of sound of 1570 m s−1, with a grid size of 0.25 × 0.25 × 1 mm and boundary conditions of water at 37°C on all surfaces. To model feedback control, temperatures on the simulation grid were averaged spatially and temporally, and zero-mean Gaussian noise with a standard deviation of 0.5 was added to match the resolution, sampling rate and background temperature measurement noise of MR thermometry (1 × 1 × 5 mm, 5 s, ± 0.5°C). Controller settings that appeared promising in simulation were tested in a tissue-mimicking gel made primarily of agar and condensed milk Citation[60], under similar conditions to those used for in vivo experiments.
Sonication, drug administration and tissue harvesting
MRI-controlled focused ultrasound was used to heat 10 to 15 mm diameter regions within the thigh to 43°C for a minimum of 20 min. The temperature elevation and exposure duration were chosen to be sufficient to trigger drug release from temperature-sensitive liposomes Citation[3] without causing significant tissue damage Citation[61].
Pegylated lyso-thermosensitive liposomal doxorubicin (LTLD) (Thermodox®, Celsion, Columbia, MD) was provided by the manufacturer at a concentration of 2 mg doxorubicin/mL. Rabbits were prescribed a doxorubicin dose of 2.5 mg/kg, administered as a dilution of LTLD in 5% dextrose sterile solution to an injection volume of 10 mL, infused slowly into the ear vein during MRI-controlled focused ultrasound heating. Once the average temperature in the target region reached 43°C (approximately 6 min), the 10 mL infusion volume was administered over 8 min at 1.2 mL/min using an MRI-compatible injection system (Spectris Solaris EP, MEDRAD, Warrendale, PA). The dose used in these experiments corresponds to a human equivalent dose of approximately 30 mg/m2 Citation[62], which is similar to what has been used in dogs (14–20 mg/m2 Citation[13]) and rats (30 mg/m2 Citation[15]), and consistent with what is being administered in current clinical trials.
Upon completion of heating, image acquisition continued until target temperatures returned to resting temperature to quantify the total thermal dose accumulated in the target region and surrounding tissues. Post-treatment T2-weighted and contrast-enhanced T1-weighted images (0.2 mmol/kg, Omniscan, GE Healthcare) were acquired to assess changes in tissue perfusion and/or tissue damage caused by ultrasound heating. After imaging, skin in the beam path was examined for burns, and trypan blue (2% weight/volume) was administered intravenously at 1.5 mL/kg to facilitate identification of sonicated regions during dissection.
Approximately 2 h after LTLD infusion, unabsorbed liposomal drug was flushed from the vasculature by transcardiac perfusion with saline. After sacrifice, 25–50 mg tissue samples placed in 1.5 mL microcentrifuge tubes were snap-frozen in liquid nitrogen and stored at −80°C. Several samples of muscle tissue were collected from both the heated region of the treated thigh, as well as unheated regions in both the treated and untreated thighs to evaluate the spatial heterogeneity of drug release.
Analysis of drug concentration and release
Tissue doxorubicin concentrations were measured by the fluorescence intensity of doxorubicin in homogenised tissue samples using published extraction techniques Citation[12], Citation[17], Citation[63–65]. Upon removal from storage, tissue samples were pulverised with pestle and mortar immersed in liquid nitrogen. The resulting powder was weighed and added to 20 volumes of acidified ethanol extraction solvent (0.3N HCl in 50% ethanol) before homogenisation with a pestle and tube grinder (Kontes® Tenbroeck, Kimble Chase LLC, Vineland, NJ), and overnight refrigeration in acidified ethanol solvent. The next day, homogenates were centrifuged at 16,000 g for 30 min at 4°C using a benchtop centrifuge (5417C, Eppendorf, Hamburg, Germany). Care was taken to pipette as much of the clear supernatant as possible into a fluorometry cuvette, but the amount varied, primarily by the mass of the sample. Acidified ethanol was added to make a standard volume of 2 mL, matching the volume used for calibration. A benchtop fluorometer (VersaFluor, Bio-Rad Laboratories, Hercules, CA) was used to measure the fluorescence intensity of doxorubicin in the extracted supernatant, recorded as the average of three readings taken with a 480 nm excitation and 590 nm emission filter set. Relative fluorescence intensities were scaled to doxorubicin concentrations by comparison with a calibration curve made from the fluorescence intensities of a serial dilution of free doxorubicin (Doxorubicin HCl, Teva Novopharm, Toronto, ON) in acidified ethanol, normalised by the mass of the sample, and corrected for variations in added extraction fluid volume. When known amounts of doxorubicin were added to thigh muscle from untreated rabbits before homogenisation, this procedure resulted in extraction efficiencies of approximately 80%. Drug concentrations are reported in this study without adjustment.
The Wilcoxon matched pairs signed rank test was used to compare the difference in drug concentrations between tissue samples from heated and unheated regions of normal thigh muscle without assuming that the data are normally distributed. Differences were considered statistically significant for values of P less than 0.05.
Results
Simulations and in vitro temperature control
shows temperature elevations in a 1 cm diameter circular target for simulations of proportional-integral control using proportional gain KP = 4.5, integral gain KI = 0.03, and an exponential input function with a target temperature elevation of 10°C and time constant τ = 160 s. This time constant was chosen to give a rise time long enough that the input could be tracked at each control point, thus preventing an initial accumulation of error and allowing the use of integral smoothing without overshoot. Temperature control using the same controller gains and exponential time constant to create a 10°C temperature elevation in a tissue-mimicking gel phantom is shown in ), demonstrating good temporal and spatial control (time to reach 95% of target temperature elevation = 365 s, steady-state mean ± temporal standard deviation = 10° ± 0.3°C, T90 = 9.3°C, T10 = 10.6°C). Similar performance was observed in simulation (rise time = 515 s, mean = 9.9° ± 0.2°C, T90 = 9.7°C, T10 = 10.1°C). Temperature maps taken at 10 min after the start of heating are shown in and ), demonstrating spatially uniform heating. Thermal dose maps calculated from these simulation and gel experiments, assuming a target temperature of 43°C, are shown in and ). These results indicate that uniform heating was maintained over time to produce a uniform cumulative effect, using multi-point control robust to either normally distributed simulated noise or periodic MRI temperature measurement fluctuations caused by transducer motion during heating.
Figure 2. Simulations (a,c,e) and in vitro (b,d,f) testing of proportional-integral MRI temperature control for mechanically-scanned focused ultrasound hyperthermia. Mean, T90 and T10 temperatures in the 10 mm diameter circular region of interest for a target temperature elevation (Tgoal) of 10°C using controller gains KP = 4.5, KI = 0.03 and an exponential input function with time constant tau = 160 seconds, in simulation (a) and in vitro, heating a tissue-mimicking gel phantom (b). Temperature maps at 10 minutes after the start of heating, and thermal dose maps calculated from the same simulation (c,e) and in vitro (d,f) controlled heating data, assuming a target temperature of 43°C. 10 mm diameter circular scan trajectory outline in white.
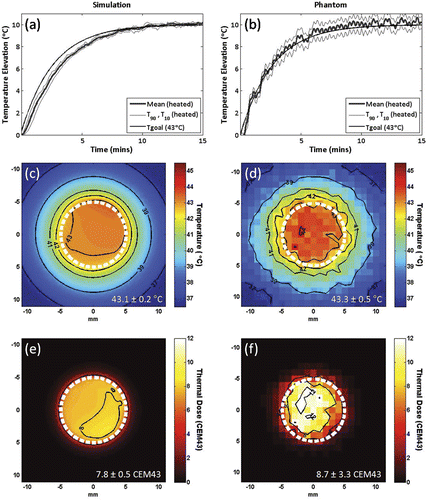
MRI-guided focused ultrasound hyperthermia in vivo
The spatial location of the heated region and the eight ROIs used for feedback control are shown for rabbit 9 in the coronal FSPGR magnitude image in , acquired in the same plane as the T2-weighted image in . The target was prescribed as a 10 mm diameter circle, centred 2 cm from the skin. Temporal evolution of the spatial temperature distribution in this plane during heating is shown in with temperatures greater than 37°C overlaid upon the corresponding magnitude images at selected time-points of 5, 10, and 20 min after heating began. Following an initial period of peripheral temperature elevation, a relatively uniform region of spatial heating was observed, with a rapid fall-off outside the target region.
Figure 3. Evolution of temperature distribution during MRI-controlled focused ultrasound heating of a 10 mm diameter region. Control ROIs, circular unheated ROI, and temperatures greater than 37°C are overlaid on coronal FSPGR magnitude images after (a) 0, (b) 5, (c) 10, and (d) 20 minutes of heating.
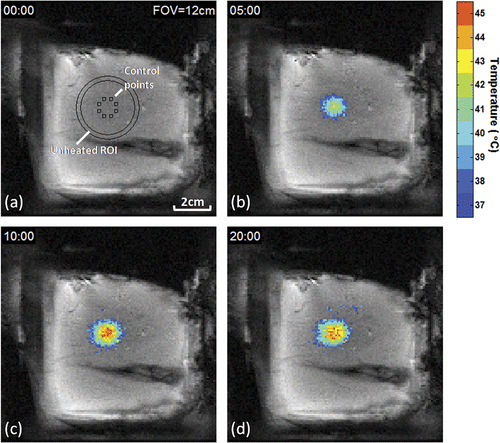
The temperature elevation in a circular ROI covering the 10 mm diameter target region is shown in for the same experiment. At each time-point, the mean ROI temperature, the temperature which 90% of the ROI exceeds (T90), and the temperature which 10% of the ROI exceeds (T10) are shown. Also shown are the mean temperatures in unheated regions 1.0 to 1.25 cm from the edge of the circular target, the controller input function Tgoal, and the start and end times of the LTLD infusion. The observed temperature increase of approximately 1°C in the unheated region represents a combination of heat conduction from the target region, variations in water bath temperature during treatment, and possible phase drift. Transducer motion caused periodic artefacts in raw FSPGR phase images that resulted in a temperature measurement standard deviation of approximately ±1°C in pre-treatment images across the target ROI, reduced to ±0.4°C when a 6-image windowed average was applied during treatment. After averaging, the mean temperature in the ROI over the treatment interval was 43.2° ± 0.3°C after a rise time of 415 s, with an average T90 and T10 of 41.7°C and 44.6°C, respectively. The thermal dose is shown as the number of cumulative equivalent min at 43°C (CEM43°C) in , and in this case had a median of 25 CEM43°C in the ROI, with CEM43°C,T90 and CEM43°C,T10 of 4 and 65 CEM43°C, respectively. Insight on the large variability in thermal dose can be gained from the mean ± standard deviation of temperature versus radius shown in , which suggests that variations result from consistently higher central temperatures rather than temporal fluctuations at a given point. This spatial non-uniformity could be decreased by adding ‘bang-bang’ control action to reduce output power if any measured temperature exceeds a certain value Citation[26], or by increasing the scan radius to reduce thermal build-up in the centre of the scan trajectory Citation[25], Citation[66]. This latter method was used in later experiments to avoid excessive thermal exposures in the central region.
Figure 4. Example of temporal and spatial temperature control over a 10 mm diameter circular area using MRI-controlled focused ultrasound hyperthermia. (a) Mean, T90 and T10 temperatures in the circular region of interest. Lyso-thermosensitive liposomal doxorubicin (LTLD) infusion duration is shaded. At the end of heating, the transducer returned to the center position and reduced temperature noise was observed. (b) Time-averaged mean ± SD temperature versus radius. Unheated region of interest is shaded. (c) Thermal dose map centered on target region, circular scan trajectory outlined in white.
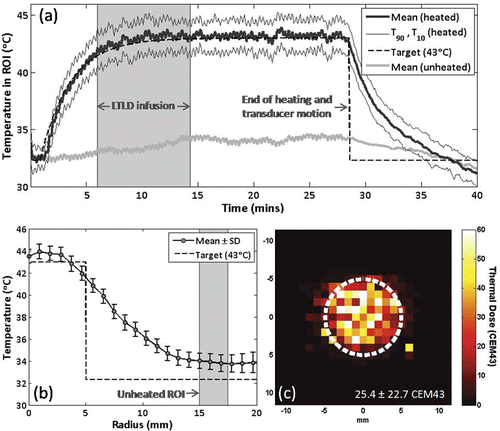
Hyperthermia experiments are summarised in , where the mean, T90 and T10 temperatures are reported as the average of each measure in the target ROI ± the standard deviation over the time interval during which the target temperature was maintained. On average, the steady state mean, T90 and T10 temperatures were 42.9°C, 41°C, and 44.8°C, respectively, and the mean temperatures had a standard deviation over the treatment duration of ±0.6°C. In four experiments, small amounts of motion (2–3 mm) caused temperature artefacts that varied across the image between 2° and 10° below the actual temperature. Continued closed-loop sonication caused higher achieved temperatures that resulted in coagulative necrosis of the target region, with an assumed thermal dose of greater than 240 CEM43°C. In these cases, tissue damage was detectable as regions of low signal intensity surrounded by high-intensity rings on post-treatment T2-weighted and contrast-enhanced T1-weighted images as shown in , and trypan blue extravasation observed after cardiac perfusion was localised to the periphery of coagulated regions. In all other experiments stable temperature imaging was achieved, and rabbits received a median thermal dose of 28.9 ± 23.4 CEM43°C. In these cases, no changes were observed on post-treatment imaging, and upon dissection little to no trypan blue extravasation was observed in the heated region.
Figure 5. Tissue damage observed using T2-weighted and contrast-enhanced T1-weighted imaging following thermal coagulation in rabbit thigh. (a,b) T2-weighted axial (a) and coronal (b) images through the center of the heated region. (c,d) Corresponding contrast-enhanced T1-weighted axial (c) and coronal (d) images from the same experiment. 80 mm field of view, target regions (outlined) and thermal damage boundaries (arrows) shown.
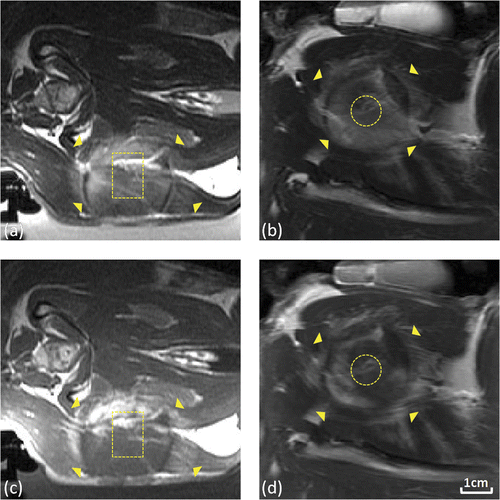
Table I. Summary of in vivo MRI-controlled FUS experiments for hyperthermia-mediated drug delivery.
Hyperthermia mediated drug delivery
The mean and standard deviation of doxorubicin concentration measured in tissue samples collected from heated and unheated regions of normal thigh muscle from each of ten rabbits is reported in . As summarised in across the six animals that received controlled hyperthermia, drug concentrations in heated regions were, on average 15.8 times higher than in the corresponding unheated regions of the contralateral thigh (95% confidence interval (CI) = 7 to 24.6 times). The pair-wise difference in doxorubicin concentration between heated and unheated regions was statistically significant (8.3 versus 0.5 ng/mg, per animal difference = 7.8 ng/mg, 95%CI = 0.8 to 14.9 ng/mg, P < 0.05, Wilcoxon matched pairs signed rank test). In the four cases where thermal coagulation occurred, coagulated regions had 9.7 times higher doxorubicin concentrations than the contralateral thigh (range = 3.7 to 18.8 times), but the pair-wise difference did not reach statistical significance (5.4 versus 0.6 ng/mg, per animal difference = 4.8 ng/mg, 95%CI = −2.2 to 11.8 ng/mg, P = 0.13).
Figure 6. Doxorubicin concentrations measured by fluorescence intensity in tissue samples harvested from heated and unheated regions of thigh muscle in rabbits receiving controlled hyperthermia (n = 6) or thermal coagulation (n = 4). Columns, mean; bars, SD. *P<0.05, Wilcoxon matched pairs signed rank test.
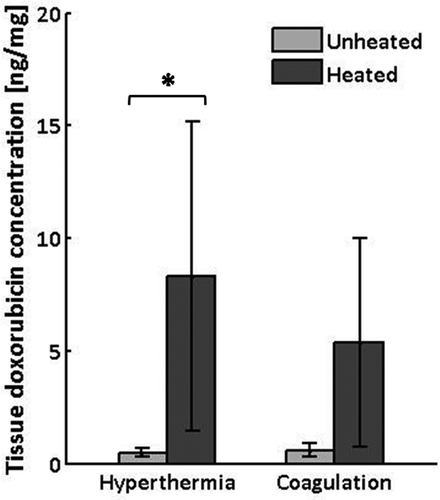
In one rabbit, additional tissue samples were harvested near the heated region to investigate drug deposition as a function of radius. shows mean and standard deviation tissue doxorubicin concentrations at 0, 5, 10, and 20 mm from the centre of the 5 mm radius heated region, as well as in the unheated contralateral thigh. Drug concentration in tissues sampled at 0, 5, and 10 mm from the centre of the treated region were higher than at 20 mm from the centre of the treated region, or in the contralateral thigh (3.3, 9.6, and 7.1 versus 0.5 and 0.3 ng/mg, respectively).
Figure 7. Doxorubicin concentrations measured by fluorescence intensity in tissue samples from rabbit #9, harvested at 0, 5, 10, and 20 mm away from the center of the heated region, as well as from the unheated contralateral thigh (mean ± SD). Overlay: time-averaged temperature profile. Inset: sample positions shown on image of mean temperatures greater than 37°C.
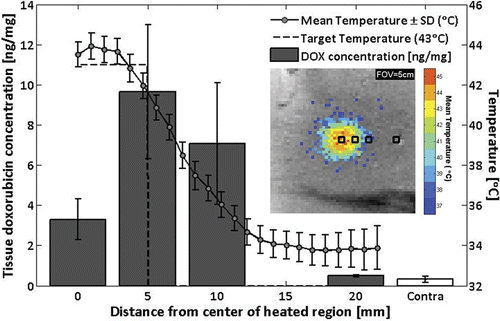
Discussion
MRI-controlled focused ultrasound hyperthermia
In this study, temporally and spatially uniform localised hyperthermia was achieved in normal rabbit thigh muscle using mechanically scanned focused ultrasound heating under multi-point proportional-integral MRI temperature control. Mean target temperatures of 42.9°C were maintained over approximately 20 min with a temporal standard deviation of 0.6°C and an average T90–T10 of 3.8°C across the 10 to 15 mm diameter circular target region.
Sources of error in MRI thermometry
A major drawback to heating with a moving ultrasound transducer in an MRI is that as the transducer moves along its circular scanning trajectory, the susceptibility distribution in the bore changes, giving rise to periodic variations in phase images and temperature measurements. Due to the exponential relationship between temperature and thermal dose, these artefactual oscillations would result in overestimated thermal dose. When applied as input for closed-loop temperature control, these fluctuations would also cause excessive fluctuations in output power, amplifying artefactual variations in temperature measurement with real fluctuations in heating. By averaging over a six-image sliding window, temperature standard deviation measured before heating was reduced from 1°C to 0.4°C, matching the temperature measurement noise observed without motion, but possibly reducing real temperature maxima and minima, resulting in an underestimation of thermal dose. Image artefacts due to transducer motion would be completely eliminated by the use of electronic scanning available with clinical phased-array systems Citation[67].
The use of rectal temperature measurements as the baseline temperature in MRI thermometry may have added a constant off-set to temperature measurements in muscle. A combination of factors, including heat conduction, variations in water bath temperature, and phase drift resulted in an observed temperature increase of approximately 1°C in untargeted muscle regions.
In these preliminary experiments, closed-loop heating was allowed to continue in cases where patient motion invalidated MRI temperature measurements, resulting in coagulation of large regions centred on the target. In future work, these sudden movements could be immediately detected in magnitude reconstructions of thermometry images to abort treatment and prevent uncontrolled heating.
Hyperthermia mediated drug delivery
The results of this study demonstrate the feasibility of using MRI-guided focused ultrasound hyperthermia with temperature-sensitive liposomes to achieve localised deposition of the anticancer drug doxorubicin in a prescribed region of rabbit thigh. Doxorubicin concentrations were significantly higher in heated than unheated tissues sampled from normal thigh muscle, and in one rabbit, doxorubicin concentrations sampled at locations 15 mm or more away from the boundary of the 10 mm diameter heated region were no different than in the unheated contralateral thigh. These results were obtained using similar LTLD and thermal dose prescriptions to those being used in Phase I/II clinical trials of LTLD combined with microwave hyperthermia in the treatment of breast cancer recurrence at the chest wall.
In previous work using thermosensitive liposomes and regional rather than localised hyperthermia in preclinical tumour models Citation[9], Citation[13], Citation[17], passive drug carrier accumulation augmented the localised enhancement in tumour drug concentration caused by triggered release. These and other studies using NTLD in various rodent tumours showed that hyperthermia increases both the rate and uniformity of liposome extravasation in angiogenic tumour vessels Citation[6], Citation[8], Citation[10], Citation[12], Citation[68], Citation[69]. Hyperthermia is thought to cause morphological changes in tumour endothelial cells, increasing the occurrence and size of pores in the vessel wall, enabling and increasing extravasation of normally impermeable particles such as 100 nm liposomes Citation[8], Citation[10]. This increased extravasation of liposomes is not seen in the vasculature of healthy muscle tissue Citation[8], suggesting that in the present study fluorescence measured in tissue after the vessels were perfused with saline may have come from bioavailable doxorubicin released from liposomes in the vasculature of the heated region. Without saline perfusion, intact liposomes would continue to circulate with a plasma half-life of approximately 1.75 h Citation[70]. These would add only slightly to the fluorescence signal measured in bulk tissue samples at the 2-h time-point because of the quenching of doxorubicin fluorescence when encapsulated at high concentration in liposomes Citation[6]. Due to the small fraction of the body being heated, free doxorubicin released in the vasculature of the target region during heating is also expected to have a minimal impact on systemic doxorubicin concentrations, even compared to the small amount of doxorubicin that leaks from the liposomes at body temperature Citation[71]. Free doxorubicin in the vasculature is rapidly taken up by tissue with an initial half-life of 8 min, followed by slow elimination with a terminal half-life of 30 h Citation[72].
Limitations and sources of variability in drug delivery
The LTLD formulation used in this study releases more than 80% of its payload in less than 20 s of exposure to temperatures greater than 41°C Citation[4], Citation[71], and remains in the bloodstream for about 4 h Citation[70], making release likely to occur as the liposome passes through the heated region. In experiments where mild hyperthermia was achieved, the duration over which the mean target temperature was greater than 41°C was 24 ± 5 min. In only two of these cases did mean temperatures ever exceed 45°C, and in those cases it was for less than two min. In experiments where motion occurred, controlled temperatures greater than 41°C were maintained for between 1 and 4 min prior to sudden motion resulting in high temperatures due to controlled heating based on invalid temperature measurements. In these cases, high power sonication continued for an average of 20 ± 6 min.
In cases where motion occurred and a high thermal dose was delivered, coagulative tissue damage may have changed the mechanism for drug accumulation. In some cases, hyperthermia may have allowed triggered release in healthy vessels prior to vascular shut-down. In other cases, high temperatures experienced early on may have caused liposome extravasation due to vessel damage, or rapid vessel collapse preventing liposomes from reaching the target tissue. Coagulation could also have prevented the removal of residual liposomes from damaged vessels by saline perfusion, exaggerating measurements of tissue drug concentration. These effects vary spatially within a heated region, and the locations from which tissue samples were collected created additional variability. In previous reports where non-thermosensitive liposomes were combined with radiofrequency ablation in canine tumours, normal rat muscle, and normal rabbit liver, high temperature ablation resulted in increased doxorubicin accumulation at the periphery of the heated region compared to the central region of coagulative necrosis Citation[16], Citation[64]. The efficacy of LTLD combined with radiofrequency ablation to treat hepatocellular carcinoma is being investigated in a series of clinical trials Citation[70].
For each experiment, total drug accumulation in heated and unheated regions was reported in as the average across 3–4 coarsely sampled 25–50 mg tissue sections. Variability in measured drug concentration between samples from the same region may be caused both by underlying variations in tissue drug concentration and sample loss during analysis. These measurements do not account for the spatial distribution of doxorubicin released in the microenvironment of the heated region, which requires further study using fluorescence microscopy in normal tissue and tumour models. Previous tumour studies demonstrated preferential liposome accumulation in some tumour regions, increased by hyperthermia Citation[10], but sequestered by their size to the perivascular space Citation[8]. Rapid triggered release of large concentrations of drug in the tumour vasculature and perivascular space may lead to the subsequent diffusion of free doxorubicin into deeper cell layers for greater antitumour effect. Further studies to characterise the spatial distributions of liposomes and bioavailable drug in the tumour microenvironment are required to evaluate the benefits of using MRI-guided FUS hyperthermia to mediate localised drug release.
Conclusions
Temporally and spatially uniform hyperthermia was localised to a target region in rabbit thigh muscle using mechanically scanned focused ultrasound under multi-point proportional-integral MRI temperature control. Administration of lyso-thermosensitive liposomal doxorubicin during localised hyperthermia resulted in localised drug release. Measured doxorubicin concentrations were significantly higher in muscle tissue sampled from heated regions 2 h after exposure. The results demonstrate the potential of MRI-controlled focused ultrasound hyperthermia for enhanced local drug delivery with temperature-sensitive drug carriers. Further experiments are required to determine the effects of temperature, heating duration, and thermal dose on drug deposition in heated regions, and on the distribution of released drug in the tumour microenvironment.
Acknowledgements
The authors thank Alexandra Garces and Shawna Rideout for providing their expertise during the rabbit experiments as well as Anthony Chau and Adam Waspe for their assistance with the positioning system.
Declaration of interest: Kullervo Hynynen and Rajiv Chopra are founders and have shares in FUS Instruments, a company that is commercialising the preclinical FUS system described in these experiments. Thermosensitive liposomes were provided by Celsion. This work is supported by funding through a Terry Fox Foundation New Frontiers Program project grant from the National Cancer Institute of Canada, and by an Ontario Research Fund grant from the Government of Ontario, Canada. The authors alone are responsible for the content and writing of the paper.
References
- Kong G, Dewhirst MW. Hyperthermia and liposomes. Int J Hyperthermia 1999; 15: 345–370
- Yatvin MB, Weinstein JN, Dennis WH, Blumenthal R. Design of liposomes for enhanced local release of drugs by hyperthermia. Science 1978; 202: 1290–1293
- Needham D, Dewhirst MW. The development and testing of a new temperature-sensitive drug delivery system for the treatment of solid tumors. Adv Drug Deliv Rev 2001; 53: 285–305
- Ponce AM, Vujaskovic Z, Yuan F, Needham D, Dewhirst MW. Hyperthermia mediated liposomal drug delivery. Int J Hyperthermia 2006; 22: 205–213
- Tashjian JA, Dewhirst MW, Needham D, Viglianti BL. Rationale for and measurement of liposomal drug delivery with hyperthermia using non-invasive imaging techniques. Int J Hyperthermia 2008; 24: 79–90
- Gaber MH, Wu NZ, Hong K, Huang SK, Dewhirst MW, Papahadjopoulos D. Thermosensitive liposomes: Extravasation and release of contents in tumor microvascular networks. Int J Radiat Oncol Biol Phys 1996; 36: 1177–1187
- Needham D, Anyarambhatla G, Kong G, Dewhirst MW. A new temperature-sensitive liposome for use with mild hyperthermia: Characterization and testing in a human tumor xenograft model. Cancer Res 2000; 60: 1197–1201
- Kong G, Braun RD, Dewhirst MW. Hyperthermia enables tumor-specific nanoparticle delivery: Effect of particle size. Cancer Res 2000; 60: 4440–4445
- Kong G, Anyarambhatla G, Petros WP, Braun RD, Colvin OM, Needham D, Dewhirst MW. Efficacy of liposomes and hyperthermia in a human tumor xenograft model: Importance of triggered drug release. Cancer Res 2000; 60: 6950–6957
- Kong G, Braun RD, Dewhirst MW. Characterization of the effect of hyperthermia on nanoparticle extravasation from tumor vasculature. Cancer Res 2001; 61: 3027–3032
- Chen Q, Krol A, Wright A, Needham D, Dewhirst MW, Yuan F. Tumor microvascular permeability is a key determinant for antivascular effects of doxorubicin encapsulated in a temperature sensitive liposome. Int J Hyperthermia 2008; 24: 475–482
- Huang SK, Stauffer PR, Hong K, Guo JW, Phillips TL, Huang A, Papahadjopoulos D. Liposomes and hyperthermia in mice: Increased tumor uptake and therapeutic efficacy of doxorubicin in sterically stabilized liposomes. Cancer Res 1994; 54: 2186–2191
- Hauck ML, LaRue SM, Petros WP, Poulson JM, Yu D, Spasojevic I, Pruitt AF, Klein A, Case B, Thrall DE, et al. Phase I trial of doxorubicin-containing low temperature sensitive liposomes in spontaneous canine tumors. Clin Cancer Res 2006; 12: 4004–4010
- Viglianti BL, Ponce AM, Michelich CR, Yu D, Abraham SA, Sanders L, Yarmolenko PS, Schroeder T, MacFall JR, Barboniak DP, et al. Chemodosimetry of in vivo tumor liposomal drug concentration using MRI. Magn Reson Med 2006; 56: 1011–1018
- Ponce AM, Viglianti BL, Yu D, Yarmolenko PS, Michelich CR, Woo J, Bally MB, Dewhirst MW. Magnetic resonance imaging of temperature-sensitive liposome release: Drug dose painting and antitumor effects. J Natl Cancer Inst 2007; 99: 53–63
- Ahmed M, Goldberg SN. Combination radiofrequency thermal ablation and adjuvant IV liposomal doxorubicin increases tissue coagulation and intratumoural drug accumulation. Int J Hyperthermia 2004; 20: 781–802
- Dromi S, Frenkel V, Luk A, Traughber B, Angstadt M, Bur M, Poff J, Xie J, Libutti SK, Li KC, et al. Pulsed-high intensity focused ultrasound and low temperature-sensitive liposomes for enhanced targeted drug delivery and antitumor effect. Clin Cancer Res 2007; 13: 2722–2727
- Patel PR, Luk A, Durrani AK, Dromi S, Cuesta J, Angstadt M, Dreher M, Wood B, Frenkel V. In vitro and in vivo evaluations of increased effective beam width for heat deposition using a split focus high intensity ultrasound (HIFU) transducer. Int J Hyperthermia 2008; 24: 537–549
- O’Neill BE, Li KCP. Augmentation of targeted delivery with pulsed high intensity focused ultrasound. Int J Hyperthermia 2008; 24: 506–520
- Hynynen K, Watmough DJ, Mallard JR. Design of ultrasonic transducers for local hyperthermia. Ultrasound Med Biol 1981; 7: 397–402
- Lele PP. Physical aspects and clinical studies with ultrasound hyperthermia. Hyperthermia in Cancer Therapy, FC Storm. Hall Medical Publishers, Boston 1983; 333–367
- Hynynen K, Roemer R, Anhalt D, Johnson C, Xu ZX, Swindell W, Cetas T. A scanned, focused, multiple transducer ultrasonic system for localized hyperthermia treatments. Int J Hyperthermia 1987; 3: 21–35
- Cain CA, Umemura S. Annular and sector phased-array applicators for ultrasound hyperthermia. IEEE Trans Ultrason Ferroelectr Freq Control 1986; 33: 110–111
- Hynynen K, Roemer R, Moros E, Johnson C, Anhalt D. The effect of scanning speed on temperature and equivalent thermal exposure distributions during ultrasound hyperthermia in vivo. IEEE Trans Microwave Theory Tech 1986; 34: 552–559
- Moros EG, Roemer RB, Hynynen K. Simulations of scanned focused ultrasound hyperthermia: The effects of scanning speed and pattern on the temperature fluctuations at the focal depth. IEEE Trans Ultrason Ferroelectr Freq Control 1988; 35: 552–560
- Lin WL, Roemer RB, Hynynen K. Theoretical and experimental evaluation of a temperature controller for scanned focused ultrasound hyperthermia. Med Phys 1990; 17: 615–625
- Johnson C, Kress R, Roemer R, Hynynen K. Multi-point feedback control system for scanned, focused ultrasound hyperthermia. Phys Med Biol 1990; 35: 781–786
- Harari PM, Hynynen KH, Roemer RB, Anhalt DP, Shimm DS, Stea B, Cassady JR. Development of scanned focussed ultrasound hyperthermia: Clinical response evaluation. Int J Radiat Oncol Biol Phys 1991; 21: 831–840
- Ishihara Y, Calderon A, Watanabe H, Okamoto K, Suzuki Y, Kuroda K, Suzuki Y. A precise and fast temperature mapping using water proton chemical shift. Magn Reson Med 1995; 34: 814–823
- Denis de Senneville B, Quesson B, Moonen CT. Magnetic resonance temperature imaging. Int J Hyperthermia 2005; 21: 515–531
- Cline HE, Hynynen K, Watkins RD, Adams WJ, Schenck JF, Ettinger RH, Freund WR, Vetro JP, Jolesz FA. Focused US system for MR imaging-guided tumor ablation. Radiology 1995; 194: 731–737
- Hynynen K, Pomeroy O, Smith DN, Huber PE, McDannold NJ, Kettenbach J, Baum J, Singer S, Jolesz FA. MR imaging-guided focused ultrasound surgery of fibroadenomas in the breast: A feasibility study. Radiology 2001; 219: 176–185
- Tempany CMC, Stewart EA, McDannold N, Quade BJ, Jolesz FA, Hynynen K. MR imaging-guided focused ultrasound surgery of uterine leiomyomas: A feasibility study. Radiology 2003; 226: 897–905
- Gianfelice D, Gupta C, Kucharczyk W, Bret P, Havill D, Clemons M. Palliative treatment of painful bone metastases with MR imaging-guided focused ultrasound. Radiology 2008; 249: 355–363
- McDannold N, Clement GT, Black P, Jolesz F, Hynynen K. Transcranial magnetic resonance imaging-guided focused ultrasound surgery of brain tumors: Initial findings in 3 patients. Neurosurgery 2010; 66: 323–332
- Hutchinson E, Dahleh M, Hynynen K. The feasibility of MRI feedback control for intracavitary phased array hyperthermia treatments. Int J Hyperthermia 1998; 14: 39–56
- Salomir R, Vimeux FC, de Zwart JA, Grenier N, Moonen CTW. Hyperthermia by MR-guided focused ultrasound: Accurate temperature control based on fast MRI and a physical model of local energy deposition and heat conduction. Magn Reson Med 2000; 43: 342–347
- Vanne A, Hynynen K. MRI feedback temperature control for focused ultrasound surgery. Phys Med Biol 2003; 48: 31–43
- Arora D, Cooley D, Perry T, Guo J, Richardson A, Moellmer J, Hadley R, Parker D, Skliar M, Roemer RB, et al. MR thermometry-based feedback control of efficacy and safety in minimum-time thermal therapies: Phantom and in-vivo evaluations. Int J Hyperthermia 2006; 22: 29–42
- Smith NB, Merrilees NK, Dahleh M, Hynynen K. Control system for an MRI compatible intracavitary ultrasound array for thermal treatment of prostate disease. Int J Hyperthermia 2001; 17: 271–282
- Chopra R, Baker N, Choy V, Boyes A, Tang K, Bradwell D, Bronskill MJ. MRI-compatible transurethral ultrasound system for the treatment of localized prostate cancer using rotational control. Med Phys 2008; 35: 1346–1357
- Palussiere J, Salomir R, Le Bail B, Fawaz R, Quesson B, Grenier N, Moonen CT. Feasibility of MR-guided focused ultrasound with real-time temperature mapping and continuous sonication for ablation of VX2 carcinoma in rabbit thigh. Magn Reson Med 2003; 49: 89–98
- McNichols RJ, Gowda A, Kangasniemi M, Bankson JA, Price RE, Hazle JD. MR thermometry-based feedback control of laser interstitial thermal therapy at 980 nm. Lasers Surg Med 2004; 34: 48–55
- Keserci BM, Kokuryo D, Suzuki K, Kumamoto E, Okada A, Khankan AA, Kuroda K. Near-real-time feedback control system for liver thermal ablations based on self-referenced temperature imaging. Eur J Radiol 2006; 59: 175–182
- Weihrauch M, Wust P, Weiser M, Nadobny J, Eisenhardt S, Budach V, Gellermann J. Adaptation of antenna profiles for control of MR guided hyperthermia (HT) in a hybrid MR-HT system. Med Phys 2007; 34: 4717–4725
- Salomir R, Palussiere J, Vimeux FC, de Zwart JA, Quesson B, Gauchet M, Lelong P, Pergrale J, Grenier N, Moonen CTW, et al. Local hyperthermia with MR-guided focused ultrasound: Spiral trajectory of the focal point optimized for temperature uniformity in the target region. J Magn Reson Imaging 2000; 12: 571–583
- Mougenot C, Salomir R, Palussiere J, Grenier N, Moonen CT. Automatic spatial and temporal temperature control for MR-guided focused ultrasound using fast 3D MR thermometry and multispiral trajectory of the focal point. Magn Reson Med 2004; 52: 1005–1015
- Mougenot C, Quesson B, de Senneville BD, de Oliveira PL, Sprinkhuizen S, Palussiere J, Grenier N, Moonen CT. Three-dimensional spatial and temporal temperature control with MR thermometry-guided focused ultrasound (MRgHIFU). Magn Reson Med 2009; 61: 603–614
- Chopra R, Curiel L, Staruch R, Morrison L, Hynynen K. An MRI-compatible system for focused ultrasound experiments in small animal models. Med Phys 2009; 36: 1867–1874
- Szebeni J, Alving CR, Rosivall L, Bunger R, Baranyi L, Bedocs P, Toth M, Barienholz Y. Animal models of complement-mediated hypersensitivity reactions to liposomes and other lipid-based nanoparticles. J Liposome Res 2007; 17: 107–117
- Chanan-Khan A, Szebeni J, Savay S, Liebes L, Rafique NM, Alving CR, Muggia FM. Complement activation following first exposure to pegylated liposomal doxorubicin (Doxil): Possible role in hypersensitivity reactions. Ann Oncol 2003; 14: 1430–1437
- Tang K, Choy V, Chopra R, Bronskill MJ. Conformal thermal therapy using planar ultrasound transducers and adaptive closed-loop MR temperature control: Demonstration in gel phantoms and ex vivo tissues. Phys Med Biol 2007; 52: 2905–2919
- Santos JM, Wright GA, Pauly JM. Flexible real-time magnetic resonance imaging framework. Conf Proc IEEE Eng Med Biol Soc 2004; 2: 1048–1051
- Peters RD, Hinks RS, Henkelman RM. Ex vivo tissue-type independence in proton-resonance frequency shift MR thermometry. Magn Reson Med 1998; 40: 454–459
- Schenck JF. The role of magnetic susceptibility in magnetic resonance imaging: MRI magnetic compatibility of the first and second kinds. Med Phys 1996; 23: 815–850
- Hynynen K. The threshold for thermally significant cavitation in dog's thigh muscle in vivo. Ultrasound Med Biol 1991; 17: 157–169
- Sun J, Hynynen K. Focusing of therapeutic ultrasound through a human skull: A numerical study. J Acoust Soc Am 1998; 104: 1705–1715
- Pichardo S, Hynynen K. Circumferential lesion formation around the pulmonary veins in the left atrium with focused ultrasound using a 2D-array endoesophageal device: A numerical study. Phys Med Biol 2007; 52: 4923–4942
- Pennes HH. Analysis of tissue and arterial blood temperatures in the resting human forearm. J Appl Physiol 1948; 1: 581–598
- Madsen EL, Frank GR, Dong F. Liquid or solid ultrasonically tissue-mimicking materials with very low scatter. Ultrasound Med Biol 1998; 24: 535–542
- McDannold NJ, King RL, Jolesz FA, Hynynen KH. Usefulness of MR imaging-derived thermometry and dosimetry in determining the threshold for tissue damage induced by thermal surgery in rabbits. Radiology 2000; 216: 517–523
- Reagan-Shaw S, Nihal M, Ahmad N. Dose translation from animal to human studies revisited. FASEB J 2008; 22: 659–661
- Bachur NR, Moore AL, Bernstein JG, Liu A. Tissue distribution and disposition of daunomycin (NCS-82151) in mice: Fluorometric and isotopic methods. Cancer Chemother Rep 1970; 54: 89–94
- Ahmed M, Liu Z, Lukyanov AN, Signoretti S, Horkan C, Monsky WL, Torchilin VP, Goldberg SN. Combination radiofrequency ablation with intratumoral liposomal doxorubicin: Effect on drug accumulation and coagulation in multiple tissues and tumor types in animals. Radiology 2005; 235: 469–477
- Treat LH, McDannold N, Vykhodtseva N, Zhang Y, Tam K, Hynynen K. Targeted delivery of doxorubicin to the rat brain at therapeutic levels using MRI-guided focused ultrasound. Int J Cancer 2007; 121: 901–907
- Lin WL, Roemer RB, Moros EG, Hynynen K. Optimization of temperature distributions in scanned, focused ultrasound hyperthermia. Int J Hyperthermia 1992; 8: 61–78
- Kohler MO, Mougenot C, Quesson B, Enholm J, Le Bail B, Laurent C, Moonen CT, Ehnholm GJ. Volumetric HIFU ablation under 3D guidance of rapid MRI thermometry. Med Phys 2009; 36: 3521–3535
- Wu NZ, Braun RD, Gaber MH, Lin GM, Ong ET, Shan S, Paphadjopoulos D, Dewhirst MW. Simultaneous measurement of liposome extravasation and content release in tumors. Microcirculation 1997; 4: 83–101
- Ning S, Macleod K, Abra RM, Huang AH, Hahn GM. Hyperthermia induces doxorubicin release from long-circulating liposomes and enhances their anti-tumor efficacy. Int J Radiat Oncol Biol Phys 1994; 29: 827–834
- Poon RT, Borys N. Lyso-thermosensitive liposomal doxorubicin: A novel approach to enhance efficacy of thermal ablation of liver cancer. Expert Opin Pharmacother 2009; 10: 333–343
- Gasselhuber A, Dreher MR, Negussie A, Wood BJ, Rattay F, Haemmerich D. Mathematical spatio-temporal model of drug delivery from low temperature sensitive liposomes during radiofrequency tumour ablation. Int J Hyperthermia 2010; 26: 499–513
- Greene RF, Collins JM, Jenkins JF, Speyer JL, Myers CE. Plasma pharmacokinetics of adriamycin and adriamycinol – Implications for the design of in vitro experiments and treatment protocols. Cancer Res 1983; 43: 3417–3421