Abstract
Inconsistency is observed in comparing assessment data of applicators for endocavitary hyperthermia (EHT) with microwaves (MW) and radiofrequency (RF) obtained using the standard method of inserting bare applicators in phantom tissues. MW antennae exhibit overall average penetration depths of approximately 6 mm, excluding hot spots. RF radiators exhibit penetration depths of not more than approximately 3 mm, a value too low considering the superior penetration of the RF plane wave radiation. Assuming that a mismatch at the RF radiator–tissue interface is causing the poor energy transfer of RF energy, we developed new RF radiators with controlled dielectric matching interfaces for evaluating the potential of RF radiation in EHT and in interstitial hyperthermia (IHT) treatments. We designed, developed and assessed 27.12 MHz, 8 mm OD inductive and capacitive devices of novel and existing designs, each provided an optimised bi-layer matching interface. The assessment results reveal features such as customisable length and shape, independence of insertion depth, uncritical air gap, longitudinal heating uniformity, outstanding penetration depths (19–20 mm) and high SAR gradients at both radiator ends – i.e. prostatic urethra ends – for added safety. These data clear the way for the development of pre-optimised EHT inductive and capacitive RF applicators. Evidence of positive effects of high near-fields density in cavity microenvironments is given. Such devices show potential for more effective prostatic hyperplasia treatments and for improving the feasibility of more adequate treatment planning and thermal dosimetry of interstitial and transurethral hyperthermia treatments of prostate carcinoma.
Introduction
Conventional hyperthermia treatment for benign prostatic hyperplasia (BPH) and prostate carcinoma is commonly performed with endoscopic modalities favoured because access by the heating devices is minimally invasive to the prostatic urethra, which crosses the prostate gland axially. For endoscopic endocavitary hyperthermia (EHT) treatments that employ microwave (MW) antennae Citation[1–19], these goals are heavily hampered by intrinsic electromagnetic (EM) limitations of the MW radiation, technology limits, and problematic optimisation procedures Citation[5–13].
The requirements for transurethral BPH treatment are moderately demanding, and many types of MW devices are employed with relatively unsatisfactory results Citation[20]. Transurethral hyperthermia treatment of prostate carcinoma has the special requirements of adequate penetration and uniform temperatures in the range of 42°–44°C over the whole prostate gland, accurate treatment planning and thermal dosimetry, and the need for sharp specific absorption rate (SAR) gradients at both ends of the prostatic urethra to protect the external sphincters. To partly achieve these requirements, the use of a MW transurethral device combined with a transrectal device is also proposed Citation[7].
Analysis of the performance of MW endoscopic EHT devices in terms of understanding the reasons for their limitations reveals incongruous results. The fast growing alternative treatment modalities for the prostate, including high-temperature Citation[20], interstitial Citation[21–22], and tissue-destructive devices Citation[23–31] with their technological limitations and their poorly defined targets and goals, suggest the inadequacy of the EHT applicator paraphernalia. Endoscopic MW catheterised antennae, used for transurethral and transrectal EHT treatment of prostate disease Citation[1–8] and for endoluminal treatment of lesions in larger cavities such as the rectum, oesophagus, cervix, vagina, and bladder, among others Citation[9–19], exhibit average penetration depths not more than circa (c.a.) 6 mm, hot spots excluded. This common penetration depth does not appear coincidental, considering that the lengths of the MW antennae range from 5 to 150 mm, with diameters (OD) from 4 to 20 cm; that the antennae are implemented at different MW frequencies, with different air gaps and cooling fluid layers; and that they are used with catheters of different thicknesses and dielectric constants.
Experimental investigations of the potential of RF radiation at 27.12 MHz for EHT, performed using non-catheterised inductive helix radiators Citation[32] and catheterised capacitive intraluminal devices Citation[29] were found to deliver penetration depths of 3 mm c.a.; these findings are in conflict also with the established greater penetration of plane waves RF radiation versus MW radiation Citation[33].
Incongruous results are further evidenced by theoretical simulation in the very wide frequency range of RF through MW frequencies of plane-waves radially propagating inside cavities of 5 mm OD, in which the penetration depth results are almost independent of frequency over the entire RF-MW range Citation[34].
A clue to rationalising these data is given by the latter result that could be ascribed in part to not having considered the near-field component of RF and MW fields. Thus, an analysis of near fields in small radius cavities appears to have priority in trying to better define the EM cavity microenvironment under investigation. Near fields exhibit a rate of decrease with distance that is much greater than that for far fields. Moreover, near fields are stronger for dipole lengths L ≪ λ and RF radiators produce more reactive near fields than do MW antennae Citation[35]. For very short dipoles (L ≪ λ), the outer boundary of the reactive near fields is taken to exist to a radial distance r, given by 0 < r < 0.62 (L3/λ) from the antenna surface. Thus, the value of r for the RF radiation is of approximately one order of magnitude smaller than that for the MW radiation, even though the effective value of r also depends on the diameter and other dipole parameters and on the complex dielectric constant of the surrounding media Citation[35]. Thus, it is plausible that the RF near-field high energy density plays a significant role in small-radius cavity microenvironments. At MW, the near-field energy is spread over a much greater distance from the antenna Citation[35] and effects of dielectrics on the heating field of MW antennae for EHT are evidenced Citation[36–39].
A preliminary analysis of cavity EM microenvironments shows that for catheterised MW antennae Citation[1–19], assessment is performed by the common practice of insertion into a phantom bulk (i.e. in direct contact). This procedure is prone to uncontrolled mismatch and instability Citation[8], depending on undefined cavity EM microenvironments. For non-catheterised RF helix radiators Citation[32], uncontrolled fixed mismatch occurs.
The aim of the present investigation is to further pursue a previous attempt to evaluate the potential of RF radiators for prostate transurethral treatments Citation[32] and validate the assumption that is the RF device–tissue mismatch that accounts for the observed incongruence in penetration. The matching of three models of RF radiators provided of stepwise adjustable bi-layer dielectric interface to manipulate the RF near fields within the EM microenvironments of small-radius cavities is investigated. The 27.12 MHz prototypes of radiator models considered are: (1) a novel open-mode toroidal inductive miniaturised radiator producing intense, highly symmetric and substantially pure H-fields, (2) a novel capacitive current device to help improving the limited penetration of 27 MHz interstitial and intraluminal capacitive current devices Citation[29]; and: (3) a helix radiator similar to an existing helix radiator tested without interface Citation[32], included for comparison purposes.
Materials and methods
Prototypes of novel RF radiators
show the 27.12 MHz prototypal structures of the RF radiators developed in the present investigation. The radiating circuits are constructed over the surface of the insulating cable mandrels (i.e. the flexible coaxial cable cylindrical nylon segments deprived of the shield) the lengths of which establish the active lengths of the radiators. Each radiator is part of a resonant circuit connected to the RF power source through a match-and-tune (M&T) external box (not shown) that includes a manually controlled tuning capacitor and matching air-transformer Citation[40].
Figure 1. New and existing prototypes of 27.12 MHz radiators developed for endocavitary hyperthermia (EHT) of small-radius cavities. (1A) the inductive helix (H) radiator; (1B) the new inverse capacitive current (C) applicator; (1C) the new inductive toroidal (T) radiator; and (1D) the existing helix U-10 radiator Citation[32] included for comparison. Physical data for each radiator are provided in .
![Figure 1. New and existing prototypes of 27.12 MHz radiators developed for endocavitary hyperthermia (EHT) of small-radius cavities. (1A) the inductive helix (H) radiator; (1B) the new inverse capacitive current (C) applicator; (1C) the new inductive toroidal (T) radiator; and (1D) the existing helix U-10 radiator Citation[32] included for comparison. Physical data for each radiator are provided in Table I.](/cms/asset/0016c330-2b7b-4d49-ac81-4bbf61f427ad/ihyt_a_521886_f0001_b.gif)
Inductive H-radiator
The helix winding of the H-radiator () is made of thin and narrow (2 × 0.1 mm) phosphor-bronze ribbon spiralled over the mandrel Citation[32]. The radius R of the coil is much smaller than the 27.12 MHz wavelength, a condition exciting the normal EM mode of the coil winding, producing significant internal and external longitudinal heating components that contribute to the heating of exposed tissues Citation[41]. This helix presents non-significant wavelength because the winding length is kept sufficiently short with respect to the RF wavelength. This H-radiator was developed using the same coaxial cable and same technology, and a similar length as the existing U-10 helix prototype Citation[32], shown in for comparison. Physical data of both H-radiators are provided in .
Table I. Radiators and heads physical data. Radiator circuits lie over the surface of a cylindrical insulating mandrel (); L and OD are radiator length and diameter; the U-10 helix radiator data are retrieved from Citation[32]. The TAA, CAW and HAW interface includes a cannula forming inner and outer interstices to be filled with specific dielectric media (); the CAW length is inclusive of both electrodes; heads cross-sections are in and longitudinal sections in . Data in mm.
Inductive T-radiator
The radiating circuit of the T-radiator prototype () consists of four symmetric and radially orientated rectangular loops, having the central conductor of the insulating mandrel as a common inner loop side. The four copper wire conductors are shaped as external loop sides and are soldered at the top to the central conductor tip, lie longitudinally on the surface of the insulating mandrel, and are soldered at the bottom to the cable shield end. Thus, the RF current from the tip of the central cable conductor splits into four currents that flow along the four parallel-connected outer loop sides to the cable shield end, producing an axial flux tube of azimuthal H-field that induces radial solenoidal currents underneath the exposed conductive layer of the cavity wall.
This short and parallel-connected circuit presents very low RF impedance and resistive losses, non-significant wavelength effects and parasitics, and does not require specific optimisation procedures. In EM terms, this structure could substantially be considered a scaled-down lumped-constant equivalent to the RF toroidal flux tube inductive applicator for external hyperthermia Citation[43], Citation[44], in which four very large circumferential apertures are made. The RF currents along the short longitudinal loop sides contribute to effective and safe heating as short strip inductors Citation[40]. Physical data of the T-radiator are provided in .
Inverse-capacitive C-radiator
The C-radiator prototype is shown in . The distal ring electrode is soldered to the tip of the central conductor at the ends of the insulating mandrel and the proximal ring electrode is soldered over the end of the cable shield. The C-radiator operates on the wall of cylindrical cavities in an inverse manner to the external capacitive devices developed for heating internal cylindrical loads. The C-radiator retains the configuration of the external devices in which the ribbon electrode widths are substantially smaller than the space between the electrodes to help limit the preferentially superficial heating of the in-between superficial tissue layer that is deleterious to the penetration Citation[42], Citation[44]. Significant wavelength effects and tissue overheating at the electrodes at the testing power level are not detected. Physical data of the C-radiator are provided in .
RF-matching interfaces and the T, H, C heads
Prior to insertion into the 8-mm inner diameter (ID) hole in the phantom cavity, each radiator described above was covered with a bottom-tapped thin plastic cannula in order to create inner and outer cylindrical interstices after insertion in the cavity. These interstices were filled with a selected combination of dielectric media to constitute a bi-layer structure–tissue interface () that operates as EM-matching transformer. Matching was sought by stepwise modifications of radial combinations of the filling dielectric media.
Figure 2. Schematic view and reference system of an RF head in the phantom cavity. The bottom-tapped thin cannula covers the RF radiator to establish inner and outer interstices that are filled with the specific dielectric media combination of the matching interface.
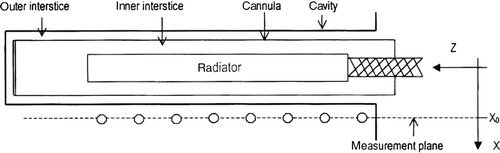
Non-conductive water and air were the only dielectric media used. Non-conductive water (W) was selected because it has a very high dielectric constant, minimal losses, is readily available, and can be easily set into any shape and thickness. Air (A) is an unavoidable part of the gap and its layer can also be easily sized and shaped. The very thin plastic cannula of fixed OD is physically compatible with all active structures, does not significantly interfere with the RF field, and establishes the precise inner and outer cylindrical interstices reported in . Each radiator composed of a bi-layer interface is referred to as a heating head. The heads 8 mm OD were chosen to be compatible with prostatic urethrae and with interstices of practical and feasible thickness. Cross-sections of the T, H, and C heads are depicted in . Diagrams of the longitudinal sections of these heads are shown in the Results section below. Physical data of the heads are reported in .
Figure 3. Cross-sectional view of TAA, HAW and CAW in the phantom cavity (not shown). The outlined components are: the insulating mandrels (grey sections) supporting the radiator structures, i.e., the four outer loop sides of the T-radiator, the helix winding of the H-radiator, and the capacitive electrodes of the C radiator. The head cannulae (dashed lines) separate the inner and outer interstices to be filled with air (A) and/or non-conducting water (W) in each radial specific matching sequence. The H, C, and T radiator prototypes are shown in , physical data of the radiators and their matching interfaces in , and longitudinal sections of all the heads in .
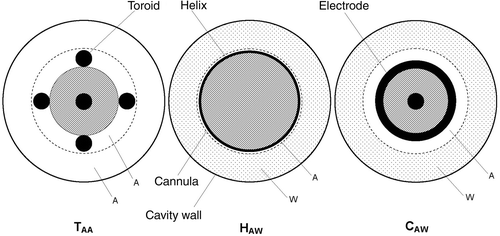
Cavity phantom
shows the phantom assembly from a previous study Citation[32] that is also used for head assessments in the present study. The phantom consists of a poly-methyl-methacrylate box sized 700 × 800 × 1400 mm, filled with polycarbonate gel that is loaded with carbon black and aluminium powder to give σ ∼ 0.2 S/m and ϵ ∼ 85 (as measured with the HP4193A Vector Impedance Analyzer (Hewlett Packard, Palo Alto, CA) and equipped with a temperature-controlled coaxial cell. A wax layer was spread over the gel surface to limit water loss. A stand was used to vertically position the heads along the z-axis of the 8-mm-ID cylindrical cavity. The phantom reference system is shown in .
Figure 4. Cavity phantom with the temperature measurement assembly Citation[32].
![Figure 4. Cavity phantom with the temperature measurement assembly Citation[32].](/cms/asset/42111c9c-07df-4fa8-8dfd-c871641ed618/ihyt_a_521886_f0004_b.gif)
A layer of 10 glass parallel capillaries (0.5 mm ID; 0.7 mm OD; Wilmad Glass, Buena, NJ) spaced at intervals of 10 mm, was supported by two x,z opposite-box own templates to provide 10 temperature measurement channels along the y-axis at x0 = 5.5 mm from the cavity z axis. A further x,z template was mounted on the carriage of a linear guide (THK, Kofu, Japan) that was driven along the y axis in 4-mm steps by a controlled step-motor. A linear array of 10 T-type wire thermocouples (0.005 mm OD; Scientific Wire, London) protected by very thin steel tubes was supported by a further x,z mobile template supporting the thermocouples to slide inside the boxed capillaries. shows heat diffusion data of capillary-thermocouple assemblies in which the selected glass assembly yields the smallest time constant.
Table II. Time constant t of capillary-thermocouple assembly immersed in controlled water bath at 24 ± 0.1°C. Capillaries are of different materials: Teflon, poly-urethane, glass, and of different OD and thickness; t is the readout time at 95% of the exponential temperature-time curves. OD and ID are in mm, t in s.
SAR data are obtained with the temperature–time method using an RF power generator (Henry Radio, Los Angeles, CA) and a dual-channel RF Wattmeter (Model 43, Bird Electronic, Bellevue, WA). The readouts of the temperature jumps Δt following the power pulses (15 W, 60 s) were obtained through a thermocouple multi-channel interface (MDP-8250; Mess Technik, Ortenburg, Germany). The five Δti (i = 0–5) readouts at radial distances ri(x0, yi) were normalised to the ΔtMAX readout of the whole set. The ΔtMAX readout is close to similar to the 7.4°C obtained with the same protocol in assessment of the RF U-10 radiator Citation[32].
Radial {R, z} iso-SAR contours of the T-head, H-head, and C-head in were obtained from readouts and normalisation of the arrays of Δt values on the x0, y, z measurement plane through the MDP-8250, and by further graphic processing (Golden Software™, Golden, CO) after symmetrisation (not shown). The radial R, z iso-SAR normalised contours of the U-10 radiator illustrated in Citation[32] are retrieved for direct comparison. Matching of the heads did not change significantly during power operations or at different insertion depths, and the reflected power remained below 3%. The phantom box is not shielded.
Figure 5. Radial iso-SAR normalized contours above the 50% SAR efficacy level for T-heads, each with a different matching interface consisting of a dual interstitial gap filled with either air (A) and/or non-conducting water (W) in different radial sequences: (top) TAW head, (middle) TWW head, (bottom) TAA head. The TAA is the only acceptable performer and the related D1/2 parameter is provided in . The heads longitudinal sections are depicted in the diagrams. The TAA cross-section is shown in and the physical data in .
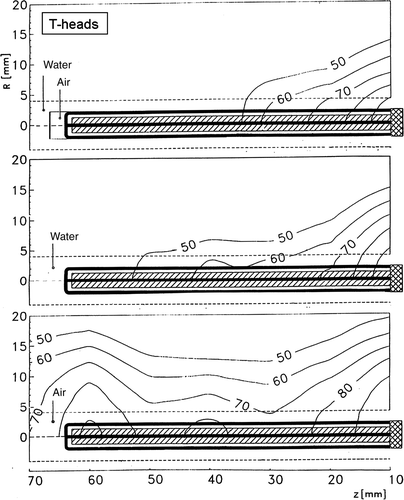
Figure 6. Radial iso-SAR normalized contours above the 50% SAR efficacy level for H-heads, each with a different matching interface consisting of a dual interstitial gap filled with either air (A) and/or non-conducting water (W) in different sequences: (top) HAW head, (middle) HWW head, (bottom) HAA head. The HAW is the only acceptable performer and the related D1/2 parameter is provided in . The heads longitudinal sections are depicted in the diagrams. The HAW cross-section is shown in and the physical data in .
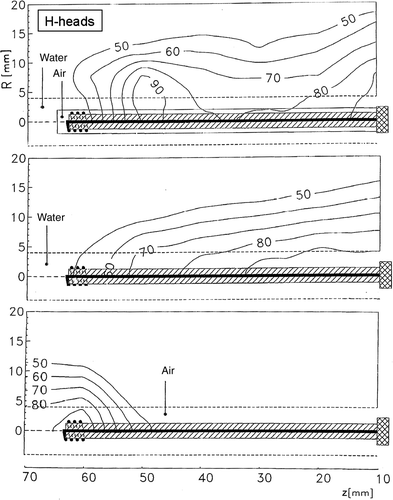
Figure 7. Radial iso-SAR normalized contours above the 50% SAR efficacy level for C-heads, each with a different matching interface consisting of a dual interstitial gap filled with either air (A) and/or nonconducting water (W) in different radial sequences: (top) CAW head, (middle) CWW head, (bottom) CAA head. The CAW is the only acceptable performer and the related D1/2 parameter is provided in . The heads longitudinal sections are depicted in the diagrams. The CAW cross-section is shown in and the physical data in .
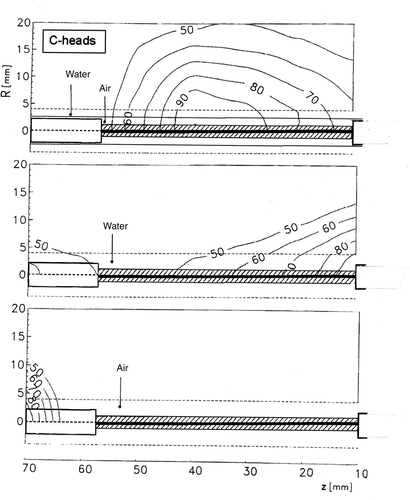
Results
The acceptance criteria for the heads include adequate longitudinal heating uniformity and penetration, absence of insertion depth effects, stability of heating features, and reproducibility. show the normalised iso-SAR contours; it is clear that only the TAA, HAW, and CAW heads show acceptable heating features. Each head was tested twice in succession under equivalent heating conditions with the selected A-A, W-W, and A-W media combinations.
illustrate the iso-SAR normalised contours of the triple interface tests of the selected TAA, HAW, and CAW heads. shows the longitudinal normalised SAR profiles constructed with points of the z-axis and the related points of the normalised SAR contours from 50%SAR to 100%SAR on the R,z planes of . The heating parameters provided in are introduced for comparison among the features of the heads and with those obtained from other representative EHT devices.
Figure 8. Normalized longitudinal SAR profiles above the 50% SAR efficacy level for the TAA, HAW and CAW heads constructed with data retrieved from the iso-SAR contours of and from . The profiles of the HLDA dipole Citation[8] and of the U-10 RF radiator Citation[32] are added for comparison. Included are traces of the active lengths of the radiators and the trace of the 80% SAR level, which are used to evaluate the numerical parameters of the profiles: Z50, Leff, Q80, d-GZ, and p-Gz that are provided in . Physical data of the heads are provided in .
![Figure 8. Normalized longitudinal SAR profiles above the 50% SAR efficacy level for the TAA, HAW and CAW heads constructed with data retrieved from the iso-SAR contours of Figures 5–7 and from Table I. The profiles of the HLDA dipole Citation[8] and of the U-10 RF radiator Citation[32] are added for comparison. Included are traces of the active lengths of the radiators and the trace of the 80% SAR level, which are used to evaluate the numerical parameters of the profiles: Z50, Leff, Q80, d-GZ, and p-Gz that are provided in Table III. Physical data of the heads are provided in Table I.](/cms/asset/75ebd758-1674-4db0-acc4-f9d6b7fc4421/ihyt_a_521886_f0008_b.gif)
Table III. RF heads heating features. The CAW Leff is inclusive of both ring electrodes and for both inductive HAW and TAA heads a pair of SAR maximum show up at each Z50 end (). The heating features of the RF U-10 radiator and of the MW HLDA, dipole and helix antennae are added for comparison: the HLDA D1/2 values are taken off the hot spot and at the hot spot (in parenthesis). Data are in mm: precision: ±15% (this work), ±20% (other sources).
The following parameters are introduced from the 50%SAR profiles shown in . The Leff ratio of the longitudinal extension Z50 of each 50%SAR profile to the related radiator length L is a measure of the relative length of the effective heating field. The Q80 ratio of the longitudinal extension Z80 of the 80%SAR level to the Z50 extension is a measure of the longitudinal uniformity of the effective heating field. The distal (d-GZ) and proximal (p-GZ) longitudinal SAR gradients of the effective heating field at the Z50 ends – together with the pair of weak SARMAX at the Z50 ends in some of the 50% SAR profiles – help to determine the sharpness of the SAR clearance at the Z50 ends (information of clinical interest regarding the safety of the prostate distal and proximal sphincters). The conservative penetration depth D1/2 parameter – i.e. the maximum of the radial half-power distance – is determined from the 50% iso-SAR contours shown in . The corresponding parameters for the HLDA dipole and the U-10 are obtained from Debicki et al. Citation[8] and Franconi et al. Citation[32], respectively.
Discussion
The TAA head – i.e. the simple T-radiator toroidal radiating structure () – is the top-performing and most versatile and safe RF device, on account of full uncritical air gap, immunity from parasitics and no access tissues overheating, advantages due to the azimuthal H-field fluxtube Citation[43] carrying highest congruent symmetry with the wall tissue and to the complementary safe and congruent heating of the parallel connected four longitudinal short loop side strip inductors Citation[40].
TAA exhibits top penetration (D1/2 ∼ 19 mm) with dumbbell-like SAR profiles and high side protrusions of Leff over L, with the SARMAX close to the proximal and the distal radiator ends producing high p-GZ, d-GZ SAR terminal gradients, and the top longitudinal heating Q80 value (, and ). Because the TAA loop sides can be adjusted to any length and easily shaped and optimised, even to a non-uniform radius, in the absence of wavelength effects and parasitics, they can easily be shaped to match the contour of different body cavities. Short and moderately flexible T-radiators such as the prototype of can be shifted back and forth along the cavity retaining stable external tuning and matching during the heating.
The TAA loop sides may be grouped in an azimuthal direction – by arranging correspondingly larger apertures along opposite loop sides – to focus RF energy for directional treatment of a localised wall lesion. Umbrella shaped top loop sides are candidates for end-fire heating of large cavity bottoms. Flexible and cooled TAA loopsides can be implemented with thin small scale metallic expansion pipes (i.e.: bellows). To the TAA highest heating rate and to the substantial absence of wavelength effects substantially contribute the parallel connection of the short radiating strips and the load-radiator conformal symmetry.
HAW heads can administer uniform and stable RF heating fields at variable insertion depths, together with dependable heating parameters similar to those of the TAA head. The side protrusions are not significant and the D1/2 is 19 mm. (, ). HAW produces iso-SAR contours similar to TAA; however, only with the contribution of the dielectric interface (), likely due to the complex modes of helix resonators Citation[41]. HAW heads are suitable for treatment requiring very flexible radiators. Optimised combinations of length and pitch are obtained for most targets provided that coiled conductor lengths are much smaller than the RF wavelength Citation[32]. The high winding losses, the complex H-field modes of the spiralled winding Citation[41] and the length-pitch ratio Citation[32] are major contributors to the lowest HAW heating rate ().
The CAW head iso-SAR contours () show a distinct SARMAX close to the proximal electrode that causes a substantial protrusion of the distal electrode beyond Z50. The Leff value is small, and the p-GZ, d-GZ, and Q80 values are similar to those of the inductive heads; the D1/2 is 20 mm in line with the other heads (; and ), a datum that asks for a reconsideration of the capacitive RF energy transfer mechanism. The CAW heating rate is acceptable.
Customisation of CAW heads includes setting the separation between electrodes required to include the target using as much as possible narrow electrodes Citation[42],Citation[44], and providing the bi-layer interface conformal to the wall cavity surface. The latter may be implemented with conformal double catheter flexible balloons. Equipped with conformal electrodes, CAW appears to have potential for heating wall segments of body cavities that have quasi-cylindrical symmetry and variable radius and length (e.g. the urethra, rectum, oesophagus, bladder, and uterus). For large cavities, CAW heads are eligible for focused treatment of localised wall lesions with conformal patch electrodes at opposite extents of lesions on wall sites of limited curvatures. Patch electrodes offer advantages over the existing RF capacitive devices for treating localised wall lesions consisting of an intraluminal electrode facing one or more external ground electrodes only in those directions in which transverse current pathways are made possible by the site anatomy Citation[45].
Prospects for the development of RF devices for EHT and IHT
The heating features of the RF heads are compared with those of existing MW and RF devices for EHT and IHT. A quantitative synopsis of the main heating features of RF heads and of representative RF and MW devices is presented in .
RF heads versus MW antennae for EHT
Comparison data were obtained from a recent comprehensive study regarding catheterised MW antennae for EHT that includes helix and dipole antennae and also the hybrid HLDA dipole – a strongly hybridised dipole with attached lumped constant inductive loads – that delivered the best performance Citation[8]. The retrieved HLDA normalised SAR profile appears in , and related parameters appear in , together with those substantially similar of MW helix and linear dipole antennae.
shows that the strong HLDA hybridisation produces an intense hot spot resulting in a very poor Q80 longitudinal quality index (26%) (), indicating that most of the MW energy is concentrated in approximately one third of the antenna length. Comparison of HLDA parameters with those of the RF heads () shows that while the Leff values are comparable, the HLDA terminal SAR gradients p-GZ and d-GZ are significantly smaller, causing a decrease of the heating effectiveness outside the hot spot Citation[8]. For HLDA, the hot spot D1/2 is approximately 15 mm, which decreases approximating common values of MW helix and dipole antennae, hot spots excluded Citation[8].
The optimisation of the MW device–tissue matching Citation[1–19] involves laborious and critical cut-and-try adjustments of antennae Citation[8], on account of large wavelength effects and parasitics due to the MW wavelengths being of the same order of magnitude as those of the devices (and targets). The customisation TAA and CAW for small radius cavities is not affected by significant wavelength effects and the design of these heads is practically target guided, whilst HAW requires preliminary cut-and-try optimisation of helix pitch and length Citation[32].
is a comprehensive qualitative synopsis of the main heating features of the RF heads and representative MW devices for EHT.
Table IV. Heating features qualitative synopsis of RF heads, RF radiators and MW applicators for EHT.
RF heads versus bare RF radiators
shows the present helix H-radiator () of the HAW head and the similar existing RF helix radiator U-10 (). These radiators are developed using the same technology and their physical data are provided in . The U-10 radiator is assessed on the phantom of protected by a thin Mylar film Citation[32] whilst the H-radiator is provided with the A-W matching interface, and both deliver heating fields with comparable and excellent Leff, p-GZ, d-GZ, and Q80 parameters (, , ; ). However, whilst the D1/2 of the U-10 is ∼3 mm, the D1/2 of the HAW is five-fold higher, unambiguously confirming the key role of the A-W interface in determining the high levels of RF energy transfer to deep tissues for helix radiators. The 27 MHz capacitive device implemented according to the interstitial multi-electrode current source (MECS) technology Citation[29], consisting of a catheter over which a pair of substantially adjacent wide electrodes is painted and protected with a thin coating, exhibits a penetration depth about six times smaller than the CAW head. Thus, CAW heads of smaller OD may usefully be developed in arrays for interstitial hyperthermia treatments. The air-coupled and bare T-radiator exhibits superior features among all proposed and existing RF EHT devices.
Figure 9. Radial iso-SAR normalized contours of the 27.12 MHz U-10 helix radiator assessed by direct insertion in the phantom bulk, protected by a thin Mylar film, and retrieved for comparison from Citation[32]. The U-10 helix radiator is shown in and the physical data in . The D1/2 parameter is provided in .
![Figure 9. Radial iso-SAR normalized contours of the 27.12 MHz U-10 helix radiator assessed by direct insertion in the phantom bulk, protected by a thin Mylar film, and retrieved for comparison from Citation[32]. The U-10 helix radiator is shown in Figure 1 and the physical data in Table I. The D1/2 parameter is provided in Table III.](/cms/asset/5b2606d6-10f1-470d-8a43-f5c6ab37a321/ihyt_a_521886_f0009_b.gif)
Pre-optimised RF heads
Pre-optimised RF heads can be developed to reproduce the stable optimised cavity EM microenvironment in the clinic. In particular, the full air-gap T-radiators are easy to be pre-optimised by customising length, size and apertures of the toroidal structure. exemplifies a prototype of a pre-optimised HAW head externally protected by a RF-transparent 8-mm OD thin sleeve, reproducing the optimised microenvironment of cavities of compatibly resilient diameters. Pre-optimised heads potentially enable the development of customisable devices, improving the feasibility of both IHT and EHT treatment planning and thermal dosimetry for effective hyperthermia treatments while relieving clinicians of many critical on-field optimisation procedures.
Conclusions
To exploit the features of these RF models, it is necessary to investigate the specific interactions in the EM microenvironment of each cavity. The evident positive and negative effects of different dielectric interfaces support the presence of high-intensity near-fields that appear to improve the heating efficiency besides indicating the need for theoretical near-fields modelling to help rationalise the disclosed phenomenology. It is also shown that the potential of the heating mechanisms of large RF devices for external hyperthermia extends over scaled down devices and that the 27 MHz radiation is adequate for effective EHT treatments.
More research is required concerning the number, thickness and diameter of interstices, the type and radial sequences of filling media and effects of dielectric properties of cooling fluids, catheters and mandrels. More research is also required regarding possible instabilities related to problematic contacts of the heads with the tissue. Nevertheless, the use of high permittivity and workable ceramics could be investigated with the aim of producing dependable and practical devices of different size and shape.
Prior to implementation in the clinical environment, further research and development is necessary to improve RF head technology with regard to versatility, dependability, and customisation; however, low frequency RF radiation shows promise as an effective EM field for hyperthermia treatment for BPH, and could improve planning and dosimetry in endoscopic as well as interstitial hyperthermia treatments of prostatic carcinoma.
Acknowledgements
The authors wish to thank Italo Vannucci, Paolo Necci, and Antonio Canichella for assistance and editing of figures.
Declaration of interest: The authors report no conflicts of interest. The authors alone are responsible for the content and writing of the paper.
References
- Mendeki J, Friedenthal E, Botslein C, Sterzer F, Paglione R, Nowodroski M, Beck E. Microwave-induced hyperthermia in cancer treatment: Apparatus and preliminary results. Int J Rad Onc Biol Phys 1978; 4: 1095–1103
- Debicki P, Okoniewski M, Oconiewska E, Shrivastava PN, Debicka AM, Baert LV, Petrovich Z. Cooled microwave transrectal applicator with adjustable directional beam for prostate treatment. Int J Hyperthermia 1995; 11: 95–108
- Sapozinc MD, Boyd SD, Astrahan MA, Gabor J, Petrovich Z. Transurethral hyperthermia for benign prostatic hyperplasia: Preliminary clinical results. J Urology 1990; 143: 944–950
- Roos D, Hamnerius Y, Alpsten M, Borghede G, Friberg L. Two microwave applicators for intracavitary hyperthermia treatment of cancer colli uteri. Phys Med Biol 1989; 34: 1917–1921
- Astrahan MA, Sapozink MD, Cohen D, Luxton G, Kampp TD, Boyd S, Petrovich Z. Microwave applicator for transurethral hyperthermia of benign prostatic hyperplasia. Int J Hyperthermia 1989; 5: 283–296
- Astrahan MA, Imanaka K, Jozsef G, Ameye F, Baert L, Sapozink MD, Boyd S, Petrovich Z. Heating characteristics of a helical microwave applicator for transurethral hyperthermia of benign prostatic hyperplasia. Int J Hyperthermia 1991; 7: 141–155
- Debicki P, Astrahan MA, Ameye F, Oven R, Baert L, Haczewski A, Petrovich Z. Temperature steering in prostate by simultaneous transurethral and transrectal hyperthermia. Urology 1992; 40: 300–307
- Debicki PS, Haczewski A, Baert LV, Petrovich Z. Microwave transurethral applicator with loaded-dipole-antenna (HLDA) for prostate treatment. Int J Hyperthermia 2001; 17: 302–320
- Liu RH, Zhang EY, Gross EJ, Cetas TC. Heating pattern of helical microwave intracavitary oesophageal applicator. Int J Hyperthermia 1991; 7: 577–586
- Chou CK, MacDougall JA, Chan KW, Vora H, Howard H, Straud C, Terr L. Intracavitary hyperthermia and radiation of esophageal cancer. Electricity and magnetism in Biology and Medicine, M Blanc. San Francisco Press, San FranciscoUSA 1993; 793–796
- Li DJ, Chou CK, Luk KH, Wang JH, Xie CF, McDougall JA, Huang GZ. Design of intracavitary microwave applicator for treatment of uterine cervix carcinoma. Int J Hyperthermia 1991; 7: 693–701
- Roos D, Seegenschmied MH, Klautke G, Erb J, Sorbe B. A new microwave applicator with integrated cooling system for intracavitary hyperthermia of vaginal carcinoma. Int J Hyperthermia 1996; 12: 743–756
- Luk KH, Jiang HB, Chou CK. SAR patterns of a helical microwave intracavitary applicator. Hyperthermic Oncology, J Overgaard. Taylor & Francis, London 1984; I: 591–594
- Li DJ, Luk KH, Jiang HB, Chou CK, Hwang GH. Design and thermometry of an intracavitary microwave applicator suitable for the treatment of some vaginal and rectal cancers. Int J Rad Onc Biol Phys 1984; 10: 2155–2162
- Astrahan MA, Sapozink MD, Luxton G, Kampp TD, Petrovich Z. A technique for combining microwave hyperthermia with intraluminal brachytherapy of the oesophagus. Int J Hyperthermia 1989; 5: 37–51
- Liru Z, Jianguo W, Zhong C, Guizhu W, Lingy L, Weilian L. 2450 MHz oesophagus applicators with multi-temperature sensors and its temperature control. Int J Hyperthermia 1990; 6: 745–753
- Hand JW, Blake PR, Hopewell JW, Lambert HW, Field SB. A coaxial applicator for intracavitary hyperthermia of carcinoma of the cervix. Biomedical Thermology. Alan Liss, New York 1982; 635–639
- Valdagni R, Amichetti A, Cristoforetti L. Intracavitary hyperthermia construction and heating pattern of individualised vaginal prototype applicator. Int J Hyperthermia 1988; 4: 457–463
- Zong QR, Chou CK, MacDougall JA, Chan KW, Luk KH. Intracavitary hyperthermia applicators for treating nasopharyngeal and cervical cancers. Int J Hyperthermia 1990; 6: 997–1004
- Hoffman RM, Macdonald R, Monga M, Wilt TJ. Transurethral microwave thermotherapy versus transurethral resection for treating benign prostatic hyperplasia: A systematic review. Br J Urology 2004; 94: 1031–1036
- Kaatee RSJP, Crezee J, Kanis AP, Lagendijk JJW, Levendag PC, Visser AG. Design of applicators for a 27 MHz multielectrode current source interstitial hyperthermia system: Impedance matching and effective power. Phys Med Biol 1997; 42: 1087–1108
- Deurloo IKK, Visser AG, Morawscat M, van Geel CAJF, van Roon OC, Levendag PC. Application of a capacitive-coupling interstitial hyperthermia system at 27 MHz: Study of different applicator configurations. Phys Med Biol 1991; 36: 119–132
- Chen X, Diederich CJ, Wootton JH, Pouliot J, Hsu I-C. Optimisation-based thermal treatment planning for catheter-based ultrasound hyperthermia. Int J Hyperthermia 2010; 26: 39–55
- Shinohara K. Thermal ablation of prostate diseases: Advantages and limitations. Int J Hyperthermia 2004; 20: 679–697
- Stauffer P. Evolving technology for thermal therapy of cancer. Int J Hyperthermia 2005; 21: 731–744
- Burtnyk M, Chopra R, Bronskill M. Quantitative analysis of 3-D conformal MRI-guided transurethral ultrasound therapy of the prostate: Theoretical simulations. Int J Hyperthermia 2009; 25: 116–131
- Johannsen M, Gneveckow U, Taymoorian K, Thiesen B, Waldöfner N, Scholz R, Jung K, Jordan A, Wust P, Loening SA. Morbidity and quality of life during thermotherapy using magnetic nanoparticles in locally recurrent prostate cancer: Results of a prospective phase I trial. Int J Hyperthermia 2007; 23: 315–323
- Barry SE. Challenges in the development of magnetic particles for therapeutic applications. Int J Hyperthermia 2008; 24: 451–466
- Kok HP, van Haaren PMA, van de Kamer JB, Creeze J. Theoretical comparison of intraluminal heating techniques. Int J Hyperthermia 2007; 23: 395–411
- Malek RS, Nahen K. Laser treatment of obstructive BPH. Contemp Urology 2004; 1–5
- Diederich C. Thermal ablation and high temperature thermal therapy. Overview of technology of technology and clinical implementation. Int J Hyperthermia 2005; 21: 745–753
- Franconi C, Holowacsz J, Vrba J, Micali F, Bonacina R, Pesce F. 27 MHz flexible heating field for interstitial and endocavitary applicators. Hyperthermic Oncology, EW Gerner, TC Cetas. Arizona University Press, Tucson 1992; I: 270
- Guy AW, Lehmann J, Stonebridge JB. Therapeutic application of electromagnetic power. Proc IEEE 1974; 62: 55–75
- Vrba J, Franconi C, Lapes M. Theoretical limits for the penetration depth of intracavitary applicators. Int J Hyperthermia 1996; 12: 737–742
- Balanis CA. Antenna Theory. Wiley, New York 1982
- King R, Trembly B, Strohbehn J. The electromagnetic field of insulated antenna in a conducting or insulated medium. IEEE Trans Micro Theory Tech 1983; 31: 574–583
- Casey JP, Bausal R. The near field of an insulated dipole in a conducting or dielectric medium. IEEE Transactions MTT 1986; 34: 459–463
- Hamada L, Saito L, Yoshimura H, Ito K. Dielectric-loaded coaxial-slot antenna for interstitial microwave hyperthermia: Longitudinal control of heating patterns. Int J Hyperthermia 2000; 16: 219–229
- Gouzuonasis I, Karathanasis K, Karanasiou I, Uzunoglu N. Passive multi-frequency brain imaging and hyperthermia irradiation apparatus: The use of dielectric matching materials in phantom experiments. Meas Sci Technol 2009; 20: 104–122
- Franconi C, Tiberio CA, Raganella L, Begnozzi L. Low frequency RF twin dipole applicator for intermediate depth hyperthermia. IEEE Trans MTT 1986; 34: 612–619
- Ellinger DC, Chute FS, Vermeulen FE. Evaluation of a semi-cylindrical solenoid as an applicator for radiofrequency hyperthermia. IEEE Trans Biomed Eng 1989; 16: 967–994
- Raganella L, Banci G, Vannucci I, Franconi C, Tiberio CA. 27 MHz conformal capacitive ring (CR) applicators for uniform hyperthermic/diathermic treatment of body segments with axial fields. IEEE Trans Biomed Eng 1989; 36: 1124–1132
- Franconi C, Banci G, Tiberio CA. RF H-field fluxtubes for safe and controlled hyperthermia. Int J Hyperthermia 1994; 10: 537–551
- Franconi C. Hyperthermia heating technology and devices. Physics and Technology of Hyperthermia, SB Field, C Franconi. Nijhoff, Amsterdam 1987; 80–122
- Hirachi Y, Nakajo M, Takeshita T, Churei H. The position of the opposite flat applicator changes the SAR and thermal distributions of the RF capacitive intracavitary hyperthermia. Int J Hyperthermia 2000; 16: 193–203