Abstract
Transurethral ultrasound therapy uses real-time magnetic resonance (MR) temperature feedback to enable the 3D control of thermal therapy accurately in a region within the prostate. Previous canine studies showed the feasibility of this method in vivo. The aim of this study was to reduce the procedure time, while maintaining targeting accuracy, by investigating new combinations of treatment parameters. Simulations and validation experiments in gel phantoms were used, with a collection of nine 3D realistic target prostate boundaries obtained from previous preclinical studies, where multi-slice MR images were acquired with the transurethral device in place. Acoustic power and rotation rate were varied based on temperature feedback at the prostate boundary. Maximum acoustic power and rotation rate were optimised interdependently, as a function of prostate radius and transducer operating frequency. The concept of dual frequency transducers was studied, using the fundamental frequency or the third harmonic component depending on the prostate radius. Numerical modelling enabled assessment of the effects of several acoustic parameters on treatment outcomes. The range of treatable prostate radii extended with increasing power, and tended to narrow with decreasing frequency. Reducing the frequency from 8 MHz to 4 MHz or increasing the surface acoustic power from 10 to 20 W/cm2 led to treatment times shorter by up to 50% under appropriate conditions. A dual frequency configuration of 4/12 MHz with 20 W/cm2 ultrasound intensity exposure can treat entire prostates up to 40 cm3 in volume within 30 min. The interdependence between power and frequency may, however, require integrating multi-parametric functions in the controller for future optimisations.
Introduction
Low to intermediate-risk localised prostate cancer represents the majority of newly diagnosed disease as a result of widespread screening with PSA Citation1 and transrectal biopsy Citation2, Citation3. Conventional treatments such as radiation therapy or radical prostatectomy can offer good control of local disease Citation4, but are associated with significant long-term complications to sexual, urinary, and bowel function Citation5, Citation6. For patients with favourable-risk disease, the influence of treatment strategy on quality of life becomes a primary consideration. One approach to address this gap between effective treatment and preservation of quality of life has been the development of minimally invasive, image-guided treatments for localised prostate cancer Citation7, Citation8. Part of the philosophy behind these technologies is that accurate knowledge of the extent and location of prostate cancer within the gland and surrounding tissues during treatment could result in more precise treatments with reduced complications. In addition, an ability to treat localised prostate cancer in a single session with short recovery time could have significant benefits in terms of patient acceptance and health care costs. Transrectal high intensity focused ultrasound (HIFU) therapy is one alternative to classical prostate treatments Citation9–12. Other investigations have demonstrated the capability of high-intensity ultrasound energy for treating prostate tissues using transrectal, interstitial or transurethral devices Citation13–17. Long-term clinical follow-up of ultrasound-guided transrectal HIFU confirms that this treatment of localised prostate cancer is feasible, and can achieve acceptable local control of disease with the potential for a reduced impact on quality of life Citation7, Citation8, Citation18–20.
In parallel, significant progress has been made in the use of magnetic resonance imaging (MRI) for the identification of cancer within the gland. Integrating high-intensity ultrasound treatments into the MRI environment for combined diagnosis and treatment could present many advantages for the management of localised prostate cancer. Additionally, the capability of MRI to perform non-invasive thermometry during treatment enables accurate monitoring of the spatial heating pattern produced by ultrasound therapy, as has been shown in many applications Citation21–28. Interstitial and intracavitary ultrasound devices have been developed for the treatment of various cancers Citation29, with the ability of producing localised heat deposition in a target tissue volume with little heating of the surrounding tissues Citation17, Citation30–36. Accurate control of treatment can be achieved by combining intracavitary ultrasound devices with MR thermometry (MRT) feedback Citation37–40.
Our group has been developing an MRI-compatible transurethral ultrasound therapy system for treatment of localised prostate cancer Citation13, Citation41–47. Preclinical canine studies have demonstrated the feasibility of generating a targeted volume of thermal coagulation within the prostate gland with an accuracy of approximately ±2 mm, using real-time MR temperature feedback control Citation46, Citation48. In current practice, however, it is important to treat the entire prostate gland, due to the multi-focal nature of prostate cancer (50 to 90% of the cases) Citation49, Citation50, and poor spatial localisation of disease within the gland with existing diagnostic approaches. By investigating full gland treatments in our study as a first approach, the aim was to develop a configuration which minimises the risk of cancer recurrence in remaining prostate or the risk of missing some tumour tissue during treatment. It was also important to show the ability of the technique to treat all prostate regions, particularly those with large prostate radii. Most studies on interstitial ultrasound treatments have reported specific configurations involving various acoustic parameters (frequency, power, transducer geometry); however, few of these studies have evaluated the trade-offs associated with varying these parameters over a wide range to highlight treatment dependencies. The use of a planar transducer has already been shown to be well suited for generating thermal subvolume lesions in the prostate gland. However, decisions on acoustic power and frequencies are critical and have to be made carefully in order to ensure successful treatment outcomes. For example, lower frequency can be important to ensure efficient heating of large prostate radii. However, low frequencies can potentially affect treatment accuracy due to insufficient attenuation in the prostate. In addition, low frequencies increase risk to surrounding tissue by depositing more energy outside the prostate gland. Wootton et al. Citation51 have investigated those effects in simulation in the context of pelvic bone safety.
Higher acoustic power can be used to ensure treatment homogeneity and to deposit ultrasound energy more rapidly in the targeted tissues. However, the use of high acoustic powers can reduce the control of heat deposition and can cause non-linear phenomena in tissues. This study evaluates acoustic parameters both in simulation and during in-vitro gel phantom experiments for ensuring full prostate heating with accurate spatial control of the heat deposition. The size and shape of the coagulated region is used to evaluate the use of low and high power and frequency to meet the objectives of complete coagulation within the prostate, treatment accuracy at the targeted boundaries, and treatment safety in surrounding tissues such as the rectum. The goal of this study, therefore, was to investigate the roles of ultrasound parameters (frequency and power) for producing spatial heating patterns that conform accurately to the entire prostate gland, and to assess any advantage in using a dual frequency heating strategy. Both simulations and subsequent validation experiments in gel phantoms were used in this study, with a collection of 3D realistic target prostate geometries obtained from previous canine studies, where multi-slice MR images covering the entire prostate were acquired with the transurethral device in place.
Materials and methods
Numerical simulations
MRI-controlled transurethral ultrasound therapy was simulated using a numerical model previously described by Burtnyk et al. Citation41, Citation42 . Briefly, the pressure distribution from a multi-element ultrasound heating applicator was calculated Citation52 and the 3D power distribution in tissue computed using prostate tissue physiological parameters summarised in . The subsequent temperature elevation was calculated, and the spatial redistribution of heat was determined using a finite-difference time-domain solution to the Pennes bio-heat transfer Citationequation 53. The target boundary in this study was the entire 3D prostate boundary, obtained by segmenting MR images of canine prostate glands. The ultrasound power produced by each transducer element was modulated independently and the direction of the ultrasound field was rotated in the 3D calculation volume according to a feedback control algorithm based on the temperature at the target boundary. Simulated treatments continued for a full 360° rotation to heat the entire target volume to a critical temperature of 55°C, chosen to represent irreversible thermal damage. Analysis parameters consisted of minimum and maximum treatable radii, treatment time, spatial targeting accuracy, volumetric undertreatment and overtreatment to evaluate the performance of various treatment regimes. Treatable radii are measured by considering the range of radii (minimum and maximum) which could be homogeneously treated, i.e. temperature ≥55°C along the entire radius. Spatial targeting accuracy was measured as the radial difference between the target boundary and the 55°C isotherm sampled along the entire prostate boundary. Then volumetric undertreatment and overtreatment were calculated by considering, respectively the total untreated tissue volume within the target volume and the total volume of tissues heated to ≥ 55°C outside the target volume. Nine canine prostate geometries with an ultrasound applicator in the urethra were used, segmented from T2-weighted MR images obtained during a previous preclinical in vivo study of MRI-guided transurethral ultrasound therapy at our institution Citation46, Citation48. Previous treatments in these canines were conservative, involving less than 30% of the gland Citation48. The simulations conducted in this study aimed to treat the prostate gland volume, using a multi-point temperature feedback controller as described by Burtnyk et al. Citation42. Prostate radius values, defined as the radial distance from the urethra centre to the outer prostate boundary, ranged from 6 to 35 mm. Prostate lengths were on average 22 ± 3 mm (range 20–25 mm) from base to apex, and prostate volumes were 22 ± 8 cm3 (range 14–41 cm3), as detailed in . The number of active elements (n) on the modelled heating applicator varied depending on the prostate length from base to apex (LB2A). The device was positioned such that the first element started at the apex of the gland. An integer number of 5-mm elements that achieved a length less than or equal to LB2A was activated for the treatment to avoid heating beyond the base of the prostate gland. Among the 1-mm-spaced prostate boundaries, the prostate boundaries chosen for treatment control were those centred at each element (i.e. 5 mm apart). In the simulation study, the temperature of the transurethral device was maintained at 37°C to match the in vivo conditions used experimentally. Prostate tissue was considered to undergo irreversible thermal damage when the temperature was elevated to 55°C. Since the resolution of MR thermometry measurements affects treatment accuracy Citation42, Citation54, Citation55, the numerical simulations were performed using realistic conditions for MR temperature feedback which incorporated appropriate temperature noise, spatial and temporal resolution parameters observed in previous in vivo studies, as described in Citation46, Citation48.
Table I. Prostate tissue physiological parameters used during numerical modelling of transurethral ultrasound prostate treatment.
Table II. Canine prostate geometries and consequences on the transducer total length and number of active elements.
Table III. MRI acquisition parameters based on preclinical canine studies.
Influence of ultrasound parameters on treatment outcomes
Targeting accuracy and treatment time achieved with a single or dual frequency approach were investigated. In addition, the influence of acoustic power on the treatment was investigated. For single frequency treatments, the range studied was 1 to 15 MHz, with maximum time-averaged surface acoustic powers (Pmax) of 10 or 20 W cm−2. During rotation of the device, acoustic powers were modulated between zero and Pmax as described in detail in paragraph 2.4. For dual frequency treatments, the transducer was driven using the fundamental frequency (flow) for large prostate radii or the third harmonic component (fhigh) for small radii (). The crossover radius (Rco) for switching between frequencies was chosen to achieve minimum treatment time with optimal treatment accuracy as described previously Citation13, Citation41. If the prostate radius was lower than Rco, then frequency was fhigh, otherwise treatment was performed using flow. The fundamental frequency of each dual frequency couple (flow/fhigh) was selected over a frequency range which enabled decreasing volumetric undertreatment while enhancing targeting accuracy and treatment time. Full treatment was defined when at least 95% of the total prostate volume exceeded 55°C. To ensure full prostate gland treatment, the effects of ultrasound parameters on minimum and maximum treatable prostate radii were studied. The influence of ultrasound power and frequency on treatment time and targeting accuracy were subsequently quantified by characterising total treatment time (s), treatment rate (cm3 min−1), lesion volume (cm3), volumetric overtreatment (cm3 and %), volumetric undertreatment (cm3 and %), and radial treatment difference (mm). Although thermal injury measured with equivalent time at 43°C is a standard for description of tissue damage induced by ultrasound therapy, the analyses were done based on the temperature data, since the goal was to control temperature during the treatment. Chopra et al. Citation48 and Yung et al. Citation56 have previously shown that differences between isotherm and isodose were very consistent over the range of temperature and treatment time studied. They have reported that comparable interpretation of the results could be done by using either temperature or thermal dose data, with differences between boundaries on the order of 1 mm. Identical analyses were done for modelling and gel phantom results.
Figure 1. (a) The concept behind 3D MRI-controlled transurethral ultrasound therapy using a dual-frequency approach. During device rotation, the ultrasound frequency is switched between the fundamental frequency flow of the transducer and its 3rd harmonic fhigh ≈ 3flow. The crossover radius, Rco, determines the distance threshold for switching frequencies and was chosen to optimize treatment time and accuracy. If the prostate radius is lower than Rco, then f = fhigh, otherwise f = flow. (b) A photograph is shown of a multielement transurethral applicator consisting of 9 active elements, each measuring 5 mm long.
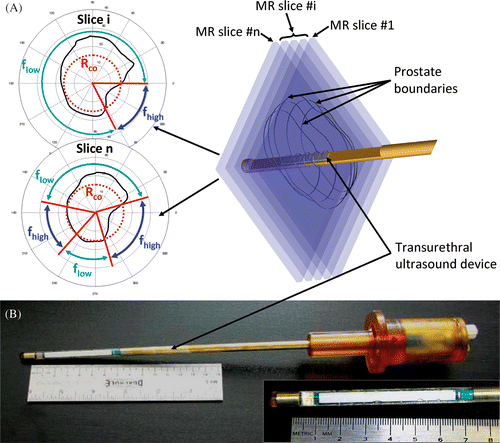
The simulations used a dynamic perfusion coefficient (constant reported by Wiart et al. Citation57 until the temperature reaches Tc = 55°C, then 0 after T ≥ Tc) and a constant attenuation coefficient for prostate tissues. Previous simulations showed no significant difference in treatment accuracy over the range of prostate volume studied in this paper (14–41 cm3) Citation42, when using for a temperature-dependent attenuation coefficient which increased significantly with increasing temperature Citation58. In this study, the attenuation increase was highly dependent on the rate of thermal dose delivery and was lower when high rates of dose were delivered, such as those delivered in ultrasonic surgery. Other studies have described less significant changes in ultrasonic attenuation when the attenuation was measured for coagulated tissues at the elevated temperature Citation59–61. In our configuration, ultrasound exposures are delivered dynamically during a rotation of the transurethral device and treated tissues are always at an elevated temperature, a situation best described by Techavipoo et al. Citation61. Based on this description, a second simulation model accounting for a temperature-dependent attenuation of the tissues was implemented. The attenuation function α(T) was defined over the temperature range 22° to 90°C as a sawtooth function composed of three different slopes. Each attenuation slope α(T)/dT was determined by considering the averaged data provided by Techavipoo et al. Citation61. Temperature derivatives of the attenuation were equal to −0.062, 0.114 and −0.034 Np m−1 MHz−1 °C−1 over the temperature ranges 22–50°C, 50–70°C and 70–90°C respectively. The 1.4 attenuation increase ratio over the temperature range 50–70°C also conformed to that reported by Gertner et al. Citation59. The initial condition was defined at ambient temperature and the initial attenuation was set to α(T = 37°C) = 6.9 Np m−1 MHz−1 (0.6 dB cm−1 MHz−1), to agree with the original simulation model based on the constant attenuation assumption. Treatment outcomes were compared between the original model and the temperature-dependent attenuation model. In particular, the influence of the attenuation variations on treatment time was discussed in the context of transurethral full prostate treatment. In vitro confirmations performed using gel phantoms enabled assessment of effects due only to acoustic parameter choices, by removing the uncertainties due to animal/tissues variations. Initial satisfactory comparisons with in vivo data have previously been done using these simple assumptions Citation13, Citation62. Although dynamic changes in attenuation probably occur in vivo, the choice to keep this parameter constant was made for comparison with the gel phantom experiments. Although an increase in attenuation with heating would result in a sharper temperature gradient, which might shift the overtreatment/undertreatment curves towards lower frequencies, the trends shown in the graphs are expected to be preserved with either set of assumptions.
Experimental transurethral ultrasound applicator
Based on the results of the simulations, a prototype MRI-compatible transurethral ultrasound applicator was fabricated (). The applicator contained a multi-element planar transducer capable of transmitting ultrasound at two discrete frequencies, 4.05 and 13.10 MHz (fundamental and third harmonic). The transducer was constructed from a rectangular plate of PZT piezoceramic (K320, PiezoTechnologies, USA). The transducer contained 10 elements, each 4 mm wide × 5 mm long, enabling simultaneous heating up to 50 mm along the device. The two resonant frequencies of the device and the electro-acoustic efficiencies at these frequencies were determined by measuring the acoustic power at discrete frequencies ranging from 1 to 18 MHz. These values were determined for each element individually using the radiation force measurement method Citation63 and a dedicated radiation force balance (Ohmic Instruments, Easton, MD, USA). The electro-acoustic transmission spectrum showed two distinct peaks at fundamental and third harmonic frequencies. Over the 10 elements of the device, the fundamental and third harmonic frequencies were on average measured at 4.05 ± 0.02 MHz (range 4.02–4.08 MHz) and 13.10 ± 0.01 MHz (range 13.08–13.12 MHz) respectively. The experimental third harmonic was slightly greater than the theoretical third harmonic which is three times the fundamental frequency. This phenomenon, previously described by Onoe et al. Citation64, was attributed to the influence of electromechanical coupling in thickness-mode resonators, leading to an fhigh/flow ratio >3 which increases with the coupling. This was also observed in previous studies investigating multi-frequency ultrasound transducers Citation44. Average electro-acoustic efficiencies for the fundamental and third harmonic frequencies were, respectively, 54 ± 5% (range 46–61%) and 29 ± 2% (range 25–33%). These transducer elements could reliably produce either Pmax = 10 or 20 W cm−2 acoustic, as required Citation43. For the gel experiments, the applicator was rotated and powered using a prototype therapy system described previously by Chopra et al. Citation43. Water cooling at 22°C was incorporated into the applicator and a flow rate of 350 mL min−1 was sufficient to remove thermal losses from the transducers. All temperatures are related to the in vivo conditions, but the gel experiments were conducted 15°C lower, for experimental convenience. This was possible since controller decisions are based on relative temperature differences Citation42 (Equation 1).
Equation 1. Control algorithm: calculating rotation rate, acoustic power and frequency.
Temperature feedback control algorithm
The control algorithm was based on temperature feedback using multi-planar MR thermometry. For each element i, the temperature at two spatial locations along the direction of ultrasound beam propagation was considered – the maximum temperature Tmaxi and the temperature Tbi at the target boundary. The goal of the control algorithm was to raise Tbi to a temperature Tc during a single complete rotation of the transurethral device Citation41, Citation42 . To avoid excessive tissue heating close to the device which could degrade sound penetration, power to a transducer element was turned off when the maximum temperature Tmaxi along the beam direction exceeded the threshold Th = 90°C. During the exposures, acoustic power (pi), frequency (fi) and rotation rate (ωi) were varied, as described in Equation 1, based on the prostate radius (ri) and the temperature differences ΔTbi and ΔThi, where ΔTbi = Tc-Tbi and ΔThi = Th−Tmaxi. Rotation rate, acoustic power and frequency were also dependent on functions tuned in preliminary simulations: kω(ri, fi, Pmax) is the rotation gain, kp(ri, fi, Pmax) is the power gain and Rco (flow, fhigh, Pmax) the crossover radius for switching between the fundamental and third harmonic in dual frequency configurations. Tuning simulations have been used previously Citation41, Citation42 and consist of performing simulations with a mono-element transducer on several circular profiles of increasing radius. By using an incremental method, optimal parameters of rotation rate and acoustic power were determined to optimise the targeting accuracy for each specific radius and at each acoustic frequency studied. Theoretical rotation and power gain functions were obtained by applying a least square curve fitting procedure to the tuning data. During final simulations and gel phantom experiments on prostate, the targeted boundaries were more complex and a multi-element ultrasound device was required to deliver heating accurately in a complex volume. Gain functions kω(ri, fi, Pmax) and kp(ri, fi, Pmax) were used by the temperature feedback control algorithm as a reference to make a decision on the rotation rate and acoustic power for each ultrasound element. Based on real-time temperature feedback, the controller modulated the rotation rates and acoustic powers around ideal values provided by the gain functions, to account for differences between simple circular boundaries used in tuning and real prostate shapes. After the calculations of pi, fi and ωi for each element, the actual rotation rate of the device (ω) was imposed by the element which required the slowest rotation (Equation 1). The values for ωmin, ωmax, and Pmax were set within the experimental therapy system. This controller considers three different cases during the treatment based on the temperature feedback: one continuous state and two limit cases. The continuous state describes the majority of the treatment provided that tuning coefficients are designed properly according to the ultrasound exposure configuration. It takes place while the target temperature at the prostate boundary is not yet reached and while the maximum temperature within the prostate remains lower than the 90°C maximum temperature threshold. In order to compensate for the lack of heat deposition when the temperature at the prostate boundary is not sufficient, device rotation rate decreases with respect to the temperature difference while acoustic power increases continuously and proportionally. This energy compensation is balanced by the continuous rotation of the device depending on the choice made on the non-zero minimum rotation rate. One limit case occurs when Tmaxi reaches the maximum authorised temperature. The treatment controller waits for the medium to cool down by setting acoustic power and rotation rate to a minimum value. The last limit case appears when the temperature at the prostate boundary reaches the target temperature. In order to avoid having an element k generate overtreatment, acoustic power pk for this element is set to the minimum and theoretical element rotation rate ωk is set to the maximum, such that ωk does not influence the decision on the effective device rotation (ω = min(ωi)1 ≤ i ≤ n). For this study, the controller used a set of gain parameters which were individually tuned for all frequencies and maximum powers investigated.
Tissue-mimicking phantom experiments
The simulation results were compared to heating experiments performed in a homogeneous, Zerdine®polyacrylamide tissue-mimicking gel phantom (CIRS, Norfolk, VA, USA) of known ultrasound and thermal properties: density 1030 kg m−3 (CIRS data sheet), speed of sound 1540 m s−1 (CIRS data sheet), thermal conductivity and heat capacity 0.56 W m−1°C−1 and 3.8 J g−1°C−1 Citation65. The attenuation coefficient at 1 MHz was measured at 0.56 dB cm−1. This value was determined using the phase-insensitive method of total acoustic power insertion loss Citation66. A radiation force balance (Ohmic Instruments) measured the change in acoustic power resulting from the insertion of a Zerdine sample of known thickness in the ultrasound path. Further description on this measurement performed in a previous study is provided by Burtnyk et al. Citation41 . Transurethral ultrasound applicators were inserted into the centre of a cylindrical container (diameter 11 cm) containing the phantom material. The apparatus was placed in a single-channel head coil of a 1.5T MR imager (Signa, GE HealthCare, Milwaukee, WI, USA) and the ultrasound applicator was connected to the MRI-compatible treatment system Citation43 for rotation and power delivery. The ultrasound device was parallel to the main magnetic field B0. Axial gradient-echo images (transverse to the transurethral device) were obtained for MR thermometry using the following parameters: n = 5 slices, TE = 10 ms, TR = 69.2 ms, NEX = 1, FOV = 20 cm, slice thickness = 5 mm, image size = 128 × 64 pixels, bandwidth = ± 15.63 kHz, flip = 30°. In order to centre the image slices accurately on each element of the transducer, these images were carefully prescribed from high resolution T2-weighted fast-spin echo images acquired along the length of the device. MR thermometry was performed using the proton resonance frequency (PRF) shift method Citation67. Treatment delivery was implemented such that each element i of the n-element transducer was controlled independently by an individual 2D treatment plane (TPi). The juxtaposition of these multiple planes led to a 3D treatment volume (TV) such that (). Three of the nine canine prostate geometries representing small, medium and large volumes were used for the gel experiments as given in . Decisions on the number of active ultrasound elements were similar to those described earlier for the simulations. Two surface acoustic power levels (Pmax = 10 or 20 W cm−2) were tested as well as single and dual frequency strategies (4.05/13.10 MHz) ().
Table IV. Prostate geometries studied during in vitro ultrasound treatments.
Results
Numerical simulations
Ultrasound parameters affecting treatable prostate radii
The range of prostate radius values which could be treated accurately, dependent on both acoustic power and frequency, was studied initially using stationary (non-rotating) ultrasound exposures performed with a five element device. Increasing the range of treatable radius is desirable to demonstrate the interest of transurethral treatment approach. The minimum treatable prostate radius (Rmin) which achieved homogeneous treatment along the entire prostate radius (all temperatures > Tc for R < Rmin) decreased with increasing frequency and increasing acoustic power ( and ). For example, at high surface acoustic power (20 W cm−2), different temperature distributions were produced for frequencies of 4, 8 and 12 MHz leading to Rmin values of 16, 7.5 and 4 mm respectively. As a consequence of Rmin being frequency dependent, the minimum prostate radius completely treated was reached at different times, from 17 to 3 s with respect to the frequencies observed (). The maximum treatable prostate radius () is of greater importance because it impacts significantly the ability of accomplishing full-gland prostate treatments. For example, the Rmax of 23 mm at 8 MHz (the frequency used in previous conservative studies), could be extended to 34 mm using 4 MHz, conferring a significant advantage for full prostate gland treatment (). The prostate radius achieved as a function of time confirms that 8 MHz cannot be used for full gland treatment, while 4 MHz seems suitable to complete a treatment of the whole gland, since our canine prostate database shows prostate radii up to 35 mm. The range of treatable prostate radii (Rmin ≤ R ≤ Rmax) extended with increasing power, and tended to narrow with decreasing frequency (). In addition, maintenance of temperature below the 90°C maximum was enhanced when using lower frequencies (). While of little effect on Rmax, increasing surface acoustic power from 10 to 20 W cm−2 caused maximal temperatures to increase, also illustrating the advantage of lower frequency for full treatment ().
Figure 2. Modeling of stationary transurethral ultrasound exposures performed with a five element planar applicator (size of each element: 4 × 5mm2). Acoustic power and frequency dependence of the minimum and maximum treatable prostate radii (homogeneous treatment).
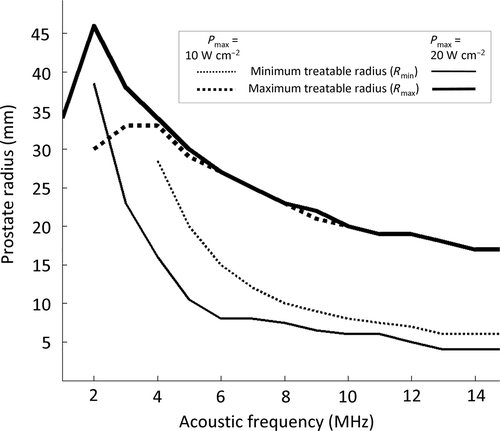
Figure 3. Simulations of stationary ultrasound heating with maximum acoustic power set to Pmax = 20 W cm−2. (a) Frequency dependency of the minimum treatable prostate radius; (b) Treatment radius as a function of time and frequency dependency of the maximum treatment radius; (c) Crossover radius for treatment optimization. Definition of the optimal range of prostate radii for a dual-frequency couple composed of fundamental and 3rd harmonic frequencies.
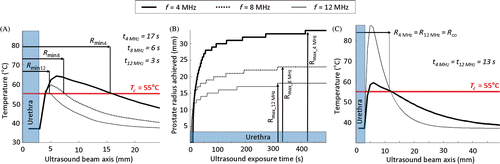
Table V. Treatable prostate radii during stationary ultrasound heating as a function of power and frequency. Maximum temperature and ultrasound exposure time associated with the maximum treatable radii.
The treatment of intermediate prostate radii was more complicated. For example, treatment time was dependent on frequency, acoustic power and prostate radius. Higher acoustic power led to shorter treatment times, all other parameters fixed. At the same acoustic power, lower frequencies were faster for large prostate radii while higher frequencies were more efficient in treating small radii homogeneously in a shorter time (). Although lower frequencies were necessary to treat large radii (maximum radius, lower maximum temperature), there were advantages for using higher frequencies (shorter treatment time for smaller radii). This observation suggested that a dual frequency approach might be beneficial for targeting a wide range of prostate radii, although such a strategy requires selecting a crossover radius (Rco) at which to switch between the low and high frequency. One approach for determining Rco is to select the radius which is heated to Tc at the same time for both frequencies. An example from a stationary exposure is shown in for frequencies 4/12 MHz where Rco = 12 mm. Frequencies 12 and 4 MHz enable reaching the same prostate radius of 12 mm at the same time (13 s). This limit radius separates two regions. 12 MHz is faster to treat radii lower than 12 mm (optimal for conservative treatments), while 4 MHz is faster to treat radii over 12 mm (optimal for full gland treatment). For dynamic exposures (with device rotation), it was also determined that Rco was dependent on the device rotation speed and acoustic power. The values reported in were determined using simulations with ideal conditions of MR temperature measurement (pixel size: 1 × 1 mm2, slice thickness: 1 mm, temperature noise: 0°C) in order to evaluate the acoustic parameters independently from imaging parameters. Similar patterns were observed for simulations with device rotation and realistic temperature measurement conditions.
Maximum temperature maps are shown in in a central thermometry slice of two simulated prostate treatments at two frequencies (4 and 8 MHz) and two surface acoustic powers (Pmax = 10 and 20 W cm−2). At 8 MHz and 10 W cm−2 (parameters that were used in previous canine studies Citation46, Citation48, and recently in a human pilot study Citation68), the device was able to treat the full prostate gland only for volumes less than 21 cm3 (, top), at the low end of expected prostate volumes in humans. For larger glands as shown in (bottom), the limited Rmax at 8 MHz led to significant undertreatment which was not improved by doubling the acoustic power due to Tmaxi reaching Th between the device and prostate boundary (). While 8 MHz was suitable for conservative treatments and full treatments in small prostates, adjustment of the frequency parameter was necessary to ensure full gland treatment in a range of canine prostate volumes. Reducing the frequency to 4 MHz greatly reduced the undertreated volume () while increasing Pmax to 20 W cm−2 further improved targeting and reduced treatment time significantly (). Treatment outcomes for the different single frequency approaches are summarised in .
Figure 4. Effects of acoustic frequency and power on transurethral prostate treatment outcomes. Modeling of the maximum temperature distribution calculated for a central plane in 2 prostate glands treated under different conditions. (a) f = 8 MHz, Pmax = 10 W cm−2, (b) f = 8 MHz, Pmax = 20 W cm−2, (c) f = 4 MHz, Pmax = 10 W cm−2, (d) f = 4 MHz, Pmax = 20 W cm−2.
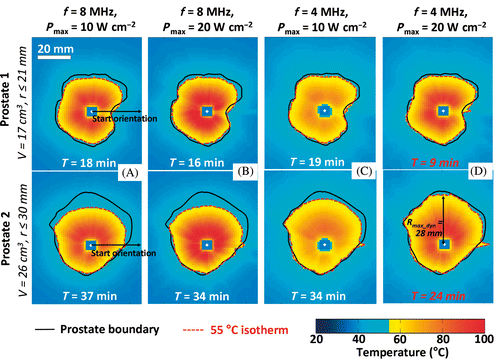
Table VI. Single frequency treatment outcomes. Comparison between f = 8 MHz and f = 4 MHz, Pmax = 10 W cm−2 and Pmax = 20 W cm−2.
In order to determine appropriate frequencies and powers for full-gland prostate therapy, data from all simulations were summarised in . The average undertreatment volumes (cm3) observed across all nine prostate geometries for treatments performed at single frequencies ranging from 1 to 15 MHz, at both 10 and 20 W cm-2, are shown in . The least undertreatment was observed between 3 and 5 MHz where eight of the nine prostates were treated to a volume fraction of 95% or greater. Using Pmax = 20 W cm−2, the undertreated volume was reduced further and treatment time was enhanced. shows the average treatment time for all single frequencies, with a minimum between 2 and 5 MHz, particularly for the higher power. In general, overtreatment almost doubled when increasing surface acoustic power from 10 to 20 W cm−2, but did not change significantly with frequency. The maximum average overtreatment volume was observed at 3 MHz yet remained acceptably small at 0.2 cm3 (range 0.1–0.2 cm3) over the nine prostate shapes studied. Lower frequency was important. Indeed, a constant enhancement was observable from frequency 15 to 5 MHz for both undertreatment and treatment time parameters. However, those same parameters were shown to be drastically affected if frequency was too low due to insignificant acoustic energy absorption. In addition, they increased risk to surrounding tissue by depositing more energy outside the prostate gland. shows the limits of using low frequencies 1 and 2 MHz, in terms of undertreated volume and treatment time. At low surface acoustic power 10 W cm−2, single frequency exposures at 1 MHz were not able to complete a treatment as there was insufficient temperature rise to satisfy to the 55°C requirement at the prostate boundary. When increasing Pmax to 20 W cm−2, the treatment was completed with low accuracy and high treatment time. Keeping high acoustic power and increasing the single frequency up to 2 MHz led to acceptable results which were comparable to those modelled for 3–5 MHz. The treatment controller accounted efficiently for non-uniform prostate shapes. The device rotation rate was modulated around the theoretical pre-tuned value according to the real-time temperature feedback ( and ). However, 2 MHz was not considered for further dual frequency investigations as preliminary tuning showed that the 2 MHz single frequency never produced better outcomes than 6 MHz for the range of prostate radii studied (). No crossover radius could be defined for switching between fundamental and third harmonic frequencies for 2/6 MHz, contrary to 3/9, 4/12, and 5/15 MHz. For example, at high surface acoustic power 20/20 W cm−2, ultrasound exposures at 4 MHz were associated with faster treatments than exposures at 12 MHz for prostate radii greater than 18 mm. Then, dual frequency dynamic exposures at 4/12 MHz were simulated at high power with a crossover radius Rco = 18 mm greater than the 12 mm suggested for static exposures ().
Figure 5. Analysis of ultrasound powers and frequencies for full canine prostate treatment. (a) Undertreated volume as a function of frequency; (b) Treatment time as a function of frequency for different powers. Appropriate choice of power/frequency enables decreasing undertreatment while reducing treatment time. Full treatment was defined when at least 95% of the total prostate volume exceeded 55°C. At Pmax = 20 W cm−2, f = 3–5 MHz, the average total undertreatment and the average treatment time reach minima. These parameters achieved full treatment in 8 of the 9 prostates. Reduced performance and extended treatment times were observed for frequencies above and below this range.
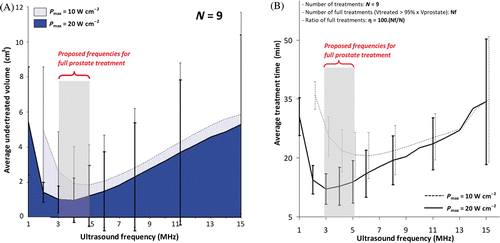
Figure 6. Pre-tuning and simulations of the rotation rate for a transurethral ultrasound device during full canine prostate treatment performed at high surface acoustic power. History of the controller chosen parameters during the rotation of the device as a function of the prostate radii faced by the transducers. (a) Single-frequency exposure at 2 MHz; (b) Frequency couple 2/6 MHz: treatments tuned at 6 MHz were faster than at 2 MHz for the entire range of radii studied. No crossover radius could be determined based on the tuning. (c) Single-frequency exposure at 4 MHz; (d) Dual-frequency exposure at 4/12 MHz: a cross-section between the tuning curves occurred in the range of radii studied and led to a crossover radius Rco of 18 mm.
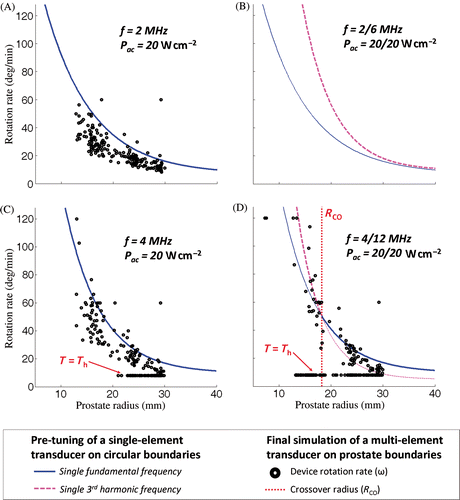
Dual frequencies for full-gland prostate treatments
For the frequency range of interest determined by the single frequency simulations (3 to 5 MHz), utilising the third harmonic for dual frequency treatments was investigated with frequency pairs 3/9, 4/12 and 5/15 MHz. Treatment time and targeting accuracy were compared for low surface acoustic power (Pmax = 10/10 W cm−2), high surface acoustic power (Pmax = 20/20W cm−2) and mixed surface acoustic power (Pmax = 20/10 W cm−2). The rationale for the mixed power was that at the higher frequencies, Th is reached very quickly during heating at 20 W cm−2. The radius where frequency was switched between fundamental and third harmonic (Rco) was pre-determined in simple tuning simulations; Rco was frequency and power dependent, varying from 9 to 18 mm. For 4/12 MHz and at high power, 12 MHz was used in 53 ± 23% (range 5–100%) of the boundaries for centre elements and in 67 ± 27% (range 4–100%) of the boundaries for side elements, due to the shorter prostate radii at the base and apex of the gland. Depending on length from base to apex (LB2A), there were respectively two centre elements on a four element transducer and three centre elements on a five-element transducer. With a dual frequency approach, targeting accuracy was conserved in prostate regions with large radii, and often improved at the base and apex of the gland. An influence on the temperature distributions inside the prostate boundaries was visible as a local temperature increase in regions of low radii and, during treatment, peak temperatures within the prostate were higher than those observed with single frequency treatment. These temperature increases had impacts on controller decisions and treatment time. This effect was particularly visible when high surface acoustic power (Pmax = 20/20 W cm−2) dual frequency configurations were used in small prostate volumes (∼20 cm3) with intermediary radii from 12–18 mm. In order to maintain the temperature in tissues below the safety threshold Th = 90°C, ultrasound elements using the third harmonic frequency at high power were frequently deactivated by the controller. The device rotation slowed to the minimum rotation rate affecting treatment time (). The use of mixed surface acoustic powers (Pmax = 20/10 W cm−2) balanced these effects by reducing the amount of energy delivered by the third harmonic frequency. In addition, the temperature gradients in regions treated with the high frequencies were steeper outside the prostate which has positive implications for treatment safety of prostate-surrounding tissues.
On average, the dual frequency ultrasound treatments maintained the treatment times and targeting accuracies obtained previously for full-gland prostate coagulation with single frequencies. Treatments at 4/12 MHz showed the best results at high surface acoustic powers (Pmax = 20/20 W cm−2) and mixed surface acoustic powers (Pmax = 20/10 W cm−2) by delivering successful full-gland treatments in eight of the nine prostates. Dual frequency configurations with mixed surface acoustic powers achieved faster treatment time with an average of 13 ± 5 min (range 8–24 min) and average untreated prostate volume was <1.0 cm3. At mixed powers, the targeting accuracy was, on average, −0.6 ± 0.4 mm (range −0.1 to −1.6 mm), and was not significantly different from the most accurate single frequency configuration (−0.6 ± 0.4 mm (range −0.2 to −1.6 mm), p = 0.9).
Average values are reported in for all ultrasound configurations simulated with the assumption of a temperature independent tissue attenuation. Additional simulations of full prostate heating were conducted on the same canine prostate boundaries with a temperature dependent attenuation. Those trials showed comparable treatment outcomes to those reported in the main study. The mixed surface acoustic power dual frequency configurations enabled achievement of full prostate heating within 16 ± 8 min (range 9–35 min), corresponding to an average treatment time difference of 3 min when compared to the equivalent treatments simulated with the temperature independent attenuation approximation. The average targeting accuracy was −0.5 ± 0.4 mm (range −0.1 to −1.5 mm) showing no significant difference with the main simulation study (p = 0.2).
Table VII. Comparison between single frequency and dual frequency simulated treatments. Highlighted in grey are the configurations evaluated experimentally. All provided values are averaged over nine different treatments simulated with nine canine prostate boundaries.
Temperature increases near the transurethral device due to the use of the third harmonic frequency 12 to 15 MHz were higher than those seen with fundamental frequencies 3 to 5 MHz. Low surface acoustic power (10/10 W cm−2) dual frequency 4/12 MHz configurations showed good temperature control with a maximum temperature Tmax over the procedure of 101 ± 1°C (range 99–103°C) on average. Despite advantages on treatment time, high surface acoustic powers 20/20 W cm−2 configurations showed less temperature control, with an average Tmax of 120 ± 3°C (range 116–123°C). Although the maximum temperatures are expected to be lower in vivo, a final alternative configuration was studied to decrease Tmax. Finally, mixed surface acoustic powers 20/10 W cm−2 were the most suitable by maintaining Tmax at 97 ± 2°C (range 95–100°C), while enhancing treatment time (). The control algorithm forced the maximum temperature under a particular temperature threshold by shutting down the element and slowing down the device (See “Materials and methods” section). Results have indicated that the maximum temperature in the prostate was still acceptable when the range of radii to be treated with a particular frequency was chosen appropriately. Configurations of mixed-power dual frequency ultrasound exposures were good compromises to optimise heat deposition while avoiding too frequent intervention of the controller to maintain the maximum temperature below an acceptable limit. Based on these results, a set of in vitro experiments was conducted in a subset of representative prostate boundaries to validate full-gland targeting accuracy.
In vitro heating experiments
Experimental comparison of single- and dual- frequency full-gland prostate treatments
The water cooling incorporated into the applicator has limited the temperature increases within the transducers due to unperfected transduction efficiency, particularly at the third harmonic frequency. After the treatment, no mechanical damage at the surface of the transducers and no decline in electro-acoustic efficiencies were noticed. The cumulative maximum temperature images measured for a centre and end thermometry slice of the prostate volume during MRI-controlled ultrasound therapy in a well-characterised gel phantom are shown in . Both single frequency (4.05 MHz) and dual frequency (4.05/13.10 MHz) treatments are shown in and for the same 21 cm3 prostate volume. The dual frequency treatment incorporated the mixed surface acoustic power configuration (Pmax = 20/10 W cm−2) and angular sectors using the high frequency are shown. For all configurations tested, full-gland canine prostates were treated with good radial targeting accuracy: 1 ± 1 mm (range −1 to +2 mm). Single frequency treatment times using 20 W cm−2 were reduced by approximately 50% compared to the 10 W cm−2 configuration (respectively averaging 10 and 21 min). The mixed surface acoustic power (Pmax = 20/10 W cm−2) dual frequency (4.05/13.10 MHz) approach was the most effective configuration overall, since it offered the best compromise between targeting accuracy and treatment time. For example, for the 21 cm3 prostate volume, a dual frequency treatment took 11 min while a single frequency treatment took 10 min ( and ). In addition, the targeting accuracy for low prostate radii (<12mm) was enhanced with the dual frequency configuration, particularly at the edges of the prostate.
Figure 7. Comparison between single- and dual-frequency treatments in gel phantoms for 2 different slices. (a) Single 4.05 MHz high power (20 W cm−2) showing the maximum temperature reached during the treatment in 2 different slices of the segmented canine prostate profile, respectively the middle and base slices; (b) Dual 4.05/13.10 MHz mixed power (20/10 W cm−2) ultrasound exposures with a crossover radius set at 12 mm; (c) Temperature profiles along the dash dot lines shown in (a) and (b). Use of the higher frequency during rotational ultrasound exposures creates a steeper temperature gradient beyond the target boundary.
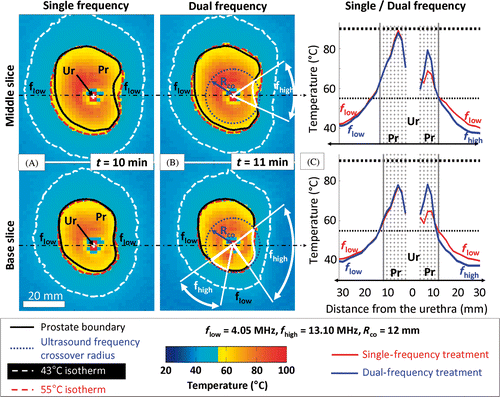
Outside the prostate gland, heat deposition extension was reduced in regions treated with the third harmonic frequency compared to the single frequency treatment. The steeper temperature gradient as a result of using the high frequency is illustrated in which shows the measured temperature profiles for both treatments at two locations (shown in and ). Improvements in treatment homogeneity were observed in cases where the prostate radii were very short (i.e. ends of the glands).
Dual frequency treatment robustness
Using the dual frequency technique and mixed surface acoustic power (Pmax = 20/10 W cm−2), full-gland prostate treatments were achieved for the three prostate volumes (21 to 41 cm3) tested. Treatments were performed in about 16.3 ± 6.1 min and treatment duration increased proportionally to prostate volume (). Treatment rate was very consistent at an average of 1.8 ± 0.2 cm3 min−1. Targeting accuracy (∼2 mm on average) was of the order of the MR thermometry in-plane voxel size used for temperature feedback (1.56 × 3.13 mm2).
Table VIII. Dual frequency mixed power treatment outcomes during gel phantom experiments in 3 different canine prostates.
Discussion
This study investigated the range of ultrasound parameters, specifically frequency and power, suitable for whole-gland prostate treatment using transurethral ultrasound therapy. In particular, the advantages of a dual frequency technique, operating at the fundamental frequency and third harmonic, were evaluated for the first time at different powers with simulations and experimentally. This study arose from observations during a previous preclinical study (8 MHz, 10 W cm−2) of an inability to treat to the outer boundary of some prostate glands. The primary result is identification of an appropriate range of operating ultrasound parameters (frequency/power) to achieve complete full-gland prostate treatments with small undertreated volumes and rapid treatment times. At Pmax = 20 W cm−2, frequencies between 3 and 5 MHz enabled treatment of large prostate glands (up to 41 cm3) in less than 30 min, while maintaining excellent targeting accuracy in the order of mm. Delivery of full-gland treatments under realistic conditions in a gel phantom confirmed the accuracy and validity of the simulations.
Single frequency treatments benefited from more aggressive parameters utilising lower frequencies and higher powers than the previous preclinical study. Treatment parameters must be carefully selected in order to cover a wide range of prostate radii, with a range from 5 mm to 35 mm observed in our database of canine prostates with the transurethral device in place. This wide range of radii can occur both from one transverse slice to the next, and within a single treatment slice. The appropriate single frequency range was selected to enable treatment of large radii up to 35 mm. This capability, however, comes with disadvantages such as difficulty in treating small radii (<12 mm) which typically occur near the base and apex of the gland. In addition, low frequencies deposit greater amounts of energy beyond the target radius which can make it difficult, in the canine model, to ensure full protection of the rectal wall.
To address these limitations, dual frequency treatments operating at the first and third harmonic offer a simple means to take advantage of the large treatment radii of low frequencies, and the improved energy deposition profile at low radii of high frequencies. A water cooling system working at room temperature enabled using both fundamental and third harmonic frequencies without damage to the transducers. The choice of crossover radius (Rco), where the frequencies switch to optimise treatment time and targeting accuracy, was found to depend on both power and frequency which required analysis of simple tuning simulations. The influence of the third harmonic on the total acoustic energy distribution was clearly visible, maintaining an equivalent treatment time while optimising the heat deposition in regions of prostate with low radii (<12 mm). One benefit was improved uniformity of the maximum temperature distribution throughout the prostate. Another benefit of the dual frequency technique was a steeper temperature gradient outside the prostate gland, which can contribute to treatment safety for surrounding tissues such as the rectum or at tissue–bone interfaces Citation41, Citation51. Finally, the last advantage was using a broader range of frequencies which widened the range of treatable radii while maintaining good accuracy.
One limitation of this approach is the use of only two discrete frequencies to treat a continuous spectrum of prostate radii. A continuous adjustment of the frequency as a function of radius would represent the ideal multi-frequency configuration, but is not yet possible from a technological point of view with the present system because standard piezoelectric materials show acceptable electro-acoustic efficiencies for ultrasound therapy only at discrete frequencies (fundamental, third harmonic). Another field of investigation for optimising dual frequency configurations could also be the use of a mixture of two frequencies for some range of prostate radii. In the present study, however, we explored two discrete frequencies as the simplest approach, which was also implementable for experimental validation in our system. In the future, many developments are possible on the basis of these first validations using dual frequencies. We are actively investigating ways to enhance the current limitations through effective combination of frequencies during heating. Some approaches have already been proposed in the context of cavitation enhancement Citation69, Citation70. However, mechanisms behind mixed frequency ultrasound exposures such as the vibration of the transducer material or the effects in biological tissues are more complex. These approaches bring up many questions requiring further investigation.
The prostate boundaries used in this study were from canine preclinical studies performed previously. Although there are differences in the shape of human and canine prostates, the range of radii (5 to 35 mm) and volumes (14–41 cm3) considered in this study are comparable to the low/middle ranges encountered in humans Citation20, Citation71 making these results relevant for developing clinical whole-gland prostate treatments. Investigating canine boundaries enabled direct comparison with previous results of conservative treatments Citation43 and allowed confident validations of more aggressive single frequency configurations in gel phantoms. This study also serves as in vitro feasibility of the dual frequency approach. If results seem to indicate that the heating times and targeting accuracies are only moderately improved when the higher harmonic is included, those results are very specific to canine boundaries. The urethra location within the gland and prostate-to-rectal-wall positions are quite different between canine and human pelvis. As a consequence, prostate radii distribution and the overall geometry lead to different treatment scenarios and controller decisions for use of the third harmonic. The slight improvements seen with dual frequency configurations in canine prostate geometries have confirmed the overall tendency. The rationale for dual frequency configurations will likely be reinforced in further investigations as much bigger improvements are expected for human geometries not only in targeting accuracy but also for rectal, neurovascular bundle and pelvic bone safety.
The experimental results of full gland prostate treatments confirm the predictions of the simulations for three prostate geometries covering a range of prostate volumes (21–41 cm3). Particularly, for dual frequency configurations, the treatment times and targeting accuracy achieved suggest possible clinical applicability compared to existing surgery and other treatment alternatives Citation8, Citation20, Citation72, Citation73. In addition, the enhancement provided by dual frequency configurations in reducing heat deposition outside the prostate gland suggests promising outcomes for safety, such as in the rectum. Further in vivo experiments in a canine model to test these parameters will be limited, however, by compromised temperature accuracy at the outer prostate boundaries and in surrounding tissues. This is due to fat surrounding the canine prostate which is a major difference compared with the human prostate. In fact, this is one of the reasons why transurethral ultrasound prostate treatments in a preclinical canine model were previously studied only for conservative treatments. Further testing of these new parameters for full-gland prostate treatment in vivo might require a different animal model.
The influence of attenuation variation due to temperature increase in prostate tissues has also been investigated in simulation and shown to have little effect on treatment outcomes. Tissue being treated is always at an elevated temperature in the technique studied and several references describe a modest attenuation increase at temperatures from 55° to 90°C Citation59–61. It is not clear that attenuation is significantly increased in damaged tissues when measured at temperatures in the range 55° to 90°C, especially when ultrasound exposures are delivered dynamically during a rotation of the device. For the slowest step corresponding to a static exposure of 2 min (no motion of the device), the ratio between the attenuation at 70°C and the attenuation at 55°C does not exceed 1.3 Citation59. Moreover, the attenuation tends to decrease for temperatures from 70°C to 90°C Citation61, compensating for the previous attenuation increase. Those observations may suggest that larger attenuation increases reported for some classical HIFU configurations preferentially occur in the presence of a HIFU lesion after the cooling of the tissues. However, for the modelling of the present prostate treatment approach, the first approximation of a temperature independent attenuation was confirmed to be acceptable, reinforcing the validity of experimental results obtained during gel phantom experiments. Thus, the approach of using detailed simulations which have been verified in well-characterised gel models is a powerful tool for investigating new treatment strategies. Applications with a relevant human prostate database are now being investigated.
In conclusion, we have reported a technique using transurethral high intensity ultrasound which enables fast treatments of large prostate-shaped volumes. Short treatment times (less than half an hour) and high accuracy (on the order of ±1 mm) associated with this technique support consideration of this procedure in practical terms for future in vivo full prostate gland treatment. The importance of the choice of acoustic parameters was shown in simulation by comparing various treatment aggressiveness over a range of acoustic frequency relevant for ultrasound therapy. Treatments performed at high surface acoustic power (20 W cm−2) and low frequency (4 MHz) were the best single frequency compromise for achieving full prostate gland treatment while conserving competitive treatment time and accuracy. A new dual frequency treatment configuration using either a low fundamental frequency or a high third harmonic frequency was investigated to optimise treatment outcomes in small prostate regions. Mixed surface acoustic power dual frequency treatments (20/10 W cm−2 at 4/12 MHz) enable decreasing temperature outside the prostate while conserving treatment time and accuracy as seen in optimised single frequency configurations. MR-guided dual frequency transurethral ultrasound prostate therapy shows interesting advantages by providing relatively fast and accurate treatment compared to classical HIFU techniques, with a great potential for safety enhancement in prostate surrounding tissues.
Acknowledgements
The authors thank Stephen McCormick, Ilya Kobelevskiy and James Kendall for their technical assistance, and the reviewers for suggesting investigation of temperature-dependent tissue attenuation.
Declaration of interest: Financial support was received from the Terry Fox Foundation, the Ontario Institute of Cancer Research, the Ontario Research Foundation and the Health Technology Exchange. Drs Bronskill, Chopra and Burtnyk are shareholders in Profound Medical, Toronto. The authors alone are responsible for the content and writing of the paper.
References
- Horwich A. Prostate cancer management. Ann Oncol 2004; 15S4: iv307–312
- Guichard G, Larre S, Gallina A, Lazar A, Faucon H, Chemama S, et al. Extended 21-sample needle biopsy protocol for diagnosis of prostate cancer in 1000 consecutive patients. Eur Urol 2007; 52: 430–435
- Hodge KK, McNeal JE, Stamey TA. Ultrasound guided transrectal core biopsies of the palpably abnormal prostate. J Urol 1989; 142: 66–70
- Potosky L, Davis WW, Hoffman RM, Stanford JL, Stephenson RA, Penson DF, Harlan LC. Five-year outcomes after prostatectomy or radiotherapy for prostate cancer: The prostate cancer outcomes study. J Natl Cancer Inst 2004; 96: 1358–1367
- Penson DF, McLerran D, Feng Z, Li L, Albertsen PC, Gilliland FD, Hamilton A, et al. 5-year urinary and sexual outcomes after radical prostatectomy: Results from the prostate cancer outcomes study. J Urol 2005; 173: 1701–1705
- Potosky AL, Legler J, Albertsen PC, Stanford JL, Gilliland FD, Hamilton AS, et al. Health outcomes after prostatectomy or radiotherapy for prostate cancer: Results from the prostate cancer outcomes study. J Natl Cancer Inst 2000; 92: 1582–1592
- Chapelon JY, Ribault M, Birer A, Vernier F, Souchon R, Gelet A. Treatment of localised prostate cancer with transrectal high intensity focused ultrasound. Eur J Ultrasound 1999; 9: 31–33
- Uchida T, Shoji S, Nakano M, Hongo S, Nitta M, Murota A, Nagata Y. Transrectal high-intensity focused ultrasound for the treatment of localised prostate cancer: Eight-year experience. Int J Urol 2009; 16: 881–886
- Chapelon JY, Margonari J, Vernier F, Gorry F, Ecochard R, Gelet A. In vivo effects of high intensity ultrasound on prostatic adenocarcinoma Dunning R3327. Cancer Res 1992; 52: 6353–6357
- Foster RS, Bihrle R, Sanghvi NT, Fry FJ, Donohue JP. High-intensity focused ultrasound in the treatment of prostatic disease. Eur Urol 1993; 23: S29–33
- Illing R, Chapman A. The clinical applications of high intensity focused ultrasound in the prostate. Int J Hyperthermia 2007; 23: 183–191
- Madersbacher S, Pedevilla M, Vingers L, Susani M, Marberger M. Effect of high-intensity focused ultrasound on human prostate cancer in vivo. Cancer Res 1995; 55: 3346–3351
- Chopra R, Burtnyk M, Haider MA, Bronskill MJ. Method for MRI-guided conformal thermal therapy of prostate with planar transurethral ultrasound heating applicators. Phys Med Biol 2005; 50: 4957–4975
- Diederich CJ, Burdette EC. Transurethral ultrasound array for prostate thermal therapy: Initial studies. IEEE Trans Ultrason Ferroelectr Freq Control 1996; 43: 1011–1022
- Gelet A, Chapelon JY, Margonari J, Theillere Y, Gorry F, Souchon R, et al. High-intensity focused ultrasound experimentation on human benign prostatic hypertrophy. Eur Urol 1993; 23: S44–47
- Hutchinson EB, Hynynen K. Intracavitary ultrasound phased arrays for prostate thermal therapies: MRI compatibility and in vivo testing. Med Phys 1998; 25: 2392–2399
- Lafon C, Koszek L, Chesnais S, Theillere Y, Cathignol D. Feasibility of a transurethral ultrasound applicator for coagulation in prostate. Ultrasound Med Biol 2004; 30: 113–122
- Crouzet S, Murat FJ, Pasticier G, Cassier P, Chapelon JY, Gelet A. High intensity focused ultrasound (HIFU) for prostate cancer: Current clinical status, outcomes and future perspectives. Int J Hyperthermia 2010; 26: 796–803
- Crouzet S, Rebillard X, Chevallier D, Rischmann P, Pasticier G, Garcia G, et al. Multicentric oncologic outcomes of high-intensity focused ultrasound for localized prostate cancer in 803 Patients. Eur Urol 2010; 58: 559–566
- Gelet A, Chapelon JY, Bouviera R, Rouvière O, Lyonneta D, Dubernarda JM. Transrectal high intensity focused ultrasound for the treatment of localized prostate cancer: Factors influencing the outcome. Eur Urol 2001; 40: 124–129
- Gianfelice D, Gupta C, Kucharczyk W, Bret P, Havill D, Clemons M. Palliative treatment of painful bone metastases with MR imaging-guided focused ultrasound. Radiology 2008; 249: 355–363
- Gianfelice D, Khiat A, Amara M, Belblidia A, Boulanger Y. MR imaging-guided focused US ablation of breast cancer: Histopathologic assessment of effectiveness – Initial experience. Radiology 2003; 227: 849–855
- Huber PE, Jenne JW, Rastert R, Simiantonakis I, Sinn HP, Strittmatter HJ, et al. A new noninvasive approach in breast cancer therapy using magnetic resonance imaging-guided focused ultrasound surgery. Cancer Res 2001; 61: 8441–8447
- Hynynen K, Freund WR, Cline HE, Chung AH, Watkins RD, Vetro JP, et al. A clinical, noninvasive, MR imaging-monitored ultrasound surgery method. Radiographics 1996; 16: 185–195
- Hynynen K, Pomeroy O, Smith DN, Huber PE, McDannold NJ, Kettenbach J, et al. MR imaging-guided focused ultrasound surgery of fibroadenomas in the breast: A feasibility study. Radiology 2001; 219: 176–185
- Mougenot C, Salomir R, Palussière J, Grenier N, Moonen TW. Automatic spatial and temporal temperature control for MR-guided focused ultrasound using fast 3D MR thermometry and multispiral trajectory of the focal point. Magn Reson Med 2004; 52: 1005–1015
- Tempany CM, Stewart EA, McDannold N, Quade BJ, Jolesz FA, Hynynen K. MR imaging-guided focused ultrasound surgery of uterine leiomyomas: A feasibility study. Radiology 2003; 226: 897–905
- Zhou X, He Q, Zhang A, Beckmann M, Ni C. Temperature measurement error reduction for MRI-guided HIFU treatment. Int J Hyperthermia 2010; 26: 347–358
- Lafon C, Melodelima D, Salomir R, Chapelon JY. Interstitial devices for minimally invasive thermal ablation by high intensity ultrasound. Int J Hyperthermia 2007; 23: 153–163
- Chen X, Diederich CJ, Wootton JH, Pouliot J, Hsu IC. Optimisation-based thermal treatment planning for catheter-based ultrasound hyperthermia. Int J Hyperthermia 2010; 26: 39–55
- Deardorff DL, Diederich CJ. Axial control of thermal coagulation using a multi-element interstitial ultrasound applicator with internal cooling. IEEE Trans Ultrason Ferroelectr Freq Control 2000; 47: 170–178
- Diederich CJ, Hynynen K. The development of intracavitary ultrasonic applicators for hyperthermia: A design and experimental study. Med Phys 1990; 17: 626–634
- Hynynen K. The feasibility of interstitial ultrasound hyperthermia. Med Phys 1992; 19: 979–987
- Lafon C, Chapelon JY, Prat F, Gorry F, Margonari J, Theillère Y, et al. Design and preliminary results of an ultrasound applicator for interstitial thermal coagulation. Ultrasound Med Biol 1998; 24: 113–122
- Makin IR, Mast TD, Faidi W, Runk MM, Barthe PG, Slayton MH. Miniaturized ultrasound arrays for interstitial ablation and imaging. Ultrasound Med Biol 2005; 31: 1539–1550
- Melodelima D, Lafon C, Prat F, Birer A, Cathignol D. Ultrasound cylindrical phased array for transoesophageal thermal therapy: Initial studies. Phys Med Biol 2002; 47: 4191–4203
- Diederich CJ, Stafford RJ, Nau WH, Burdette EC, Price RE, Hazle JD. Transurethral ultrasound applicators with directional heating patterns for prostate thermal therapy: In vivo evaluation using magnetic resonance thermometry. Med Phys 2004; 31: 405–413
- Kinsey AM, Tyreus PD, Rieke V, Butts K, Nau WH, Sommer G, et al. Interstitial ultrasound applicators with dynamic angular control for thermal ablation of tumors under MR-guidance. Conf Proc IEEE Eng Med Biol Soc 2004; 4: 2496–2499
- Melodelima D, Salomir R, Mougenot C, Prat F, Theillère Y, Moonen C, et al. Intraluminal ultrasound applicator compatible with MRI ‘real-time’ temperature mapping for the treatment of oesophageal tumours: An ex vivo study. Med Phys 2004; 31: 236–244
- Ross AB, Diederich CJ, Nau WH, Gill H, Bouley DM, Daniel B, et al. Highly directional transurethral ultrasound applicators with rotational control for MRI-guided prostatic thermal therapy. Phys Med Biol 2004; 49: 189–204
- Burtnyk M, Chopra R, Bronskill M. Simulation study on the heating of the surrounding anatomy during transurethral ultrasound prostate therapy: A 3D theoretical analysis of patient safety. Med Phys 2010; 37: 2862–2875
- Burtnyk M, Chopra R, Bronskill MJ. Quantitative analysis of 3-D conformal MRI-guided transurethral ultrasound therapy of the prostate: Theoretical simulations. Int J Hyperthermia 2009; 25: 116–131
- Chopra R, Baker N, Choy V, Boyes A, Tang K, Bradwell D, et al. MRI-compatible transurethral ultrasound system for the treatment of localized prostate cancer using rotational control. Med Phys 2008; 35: 1346–1357
- Chopra R, Luginbuhl C, Foster FS, Bronskill MJ. Multifrequency ultrasound transducers for conformal interstitial thermal therapy. IEEE Trans Ultrason Ferroelectr Freq Control 2003; 50: 881–889
- Chopra R, Luginbuhl C, Weymouth AJ, Foster FS, Bronskill MJ. Interstitial ultrasound heating applicator for MR-guided thermal therapy. Phys Med Biol 2001; 46: 3133–3145
- Siddiqui K, Chopra R, Vedula S, Sugar L, Haider M, Boyes A, et al. MRI-guided transurethral ultrasound therapy of the prostate gland using real-time thermal mapping: Initial studies. Urology 2010; 76: 1506–1511
- Tang K, Choy V, Chopra R, Bronskill MJ. Conformal thermal therapy using planar ultrasound transducers and adaptive closed-loop MR temperature control: Demonstration in gel phantoms and ex vivo tissues. Phys Med Biol 2007; 52: 2905–2919
- Chopra R, Tang K, Burtnyk M, Boyes A, Sugar L, Appu S, et al. Analysis of the spatial and temporal accuracy of heating in the prostate gland using transurethral ultrasound therapy and active MR temperature feedback. Phys Med Biol 2009; 54: 2615–2633
- Ahmed HU, Pendse D, Illing R, Allen C, van der Meulen JHP, Emberton M. Will focal therapy become a standard of care for men with localized prostate cancer?. Nat Clin Pract Oncol 2007; 4: 632–642
- Miller GJ, Cygan JM. Morphology of prostate cancer: The effects of multifocality on histological grade, tumor volume and capsule penetration. J Urol 1994; 152: 1709–1713
- Wootton JH, Ross AB, Diederich CJ. Prostate thermal therapy with high intensity transurethral ultrasound: The impact of pelvic bone heating on treatment delivery. Int J Hyperthermia 2007; 23: 609–622
- Ocheltree KB, Frizzel LA. Sound field calculation for rectangular sources. IEEE Trans Ultrason Ferroelectr Freq Control 1989; 36: 242–248
- Pennes HH. Analysis of tissue and arterial blood temperatures in the resting human forearm. J Appl Physiol 1948; 1: 93–122
- Chopra R, Wachsmuth J, Burtnyk M, Haider MA, Bronskill MJ. Analysis of factors important for transurethral ultrasound prostate heating using MR temperature feedback. Phys Med Biol 2006; 51: 827–844
- Pisani LJ, Ross AB, Diederich CJ, Nau WH, Sommer FG, Glover GH, et al. Effects of spatial and temporal resolution for MR image-guided thermal ablation of prostate with transurethral ultrasound. J Magn Reson Imaging 2005; 22: 109–118
- Yung JP, Shetty A, Elliott A, Weinberg JS, McNichols RJ, Gowda A, et al. Quantitative comparison of thermal dose models in normal canine brain. Med Phys 2010; 37: 5313–5321
- Wiart M, Curiel L, Gelet A, Lyonnet D, Chapelon JY, Rouviere O. Influence of perfusion on high-intensity focused ultrasound prostate ablation: A first-pass MRI study. Magn Reson Med 2007; 58: 119–127
- Damianou CA, Sanghvi NT, Fry FJ, Maass-Moreno R. Dependence of ultrasonic attenuation and absorption in dog soft tissues on temperature and thermal dose. J Acoust Soc Am 1997; 102: 628–634
- Gertner MR, Wilson BC, Sherar MD. Ultrasound properties of liver tissue during heating. Ultrasound Med Biol 1997; 23: 1395–1403
- Parmar N, Kolios MC. An investigation of the use of transmission ultrasound to measure acoustic attenuation changes in thermal therapy. Med Biol Eng Comput 2006; 44: 583–591
- Techavipoo U, Varghese T, Chen Q, Stiles TA, Zagzebski JA, Frank GR. Temperature dependence of ultrasonic propagation speed and attenuation in excised canine liver tissue measured using transmitted and reflected pulses. J Acoust Soc Am 2004; 115: 2859–2865
- Patel PR, Luk A, Durrani A, Dromi S, Cuesta J, Angstadt M, et al. In vitro and in vivo evaluations of increased effective beam width for heat deposition using a split focus high intensity ultrasound (HIFU) transducer. Int J Hyperthermia 2008; 24: 537–549
- Davidson F. Ultrasonic power balances. Output measurements for medical ultrasound, RC Preston. Springer, Berlin 1991; 75–90
- Onoe M, Tiersten HF, Meitzler AH. Shift in the location of resonant frequencies caused by large electromechanical coupling in thickness-mode resonators. J Acoust Soc Am 1963; 35: 36–42
- Davidson SR, Sherar MD. Measurement of the thermal conductivity of polyacrylamide tissue-equivalent material. Int J Hyperthermia 2003; 19: 551–562
- Parker KJ. Ultrasonic attenuation and absorption in liver tissue. Ultrasound Med Biol 1983; 9: 363–369
- Ishihara Y, Calderon A, Watanabe H, Okamoto K, Suzuki Y, Kuroda K, Suzuki Y. A precise and fast temperature mapping using water proton chemical shift. Magn Reson Med 1995; 34: 814–823
- Chopra R, Burtnyk M, N’Djin WA, Bronskill M. MRI-controlled transurethral ultrasound therapy for localised prostate cancer. Int J Hyperthermia 2010; 26: 804–821
- Liu HL, Hsieh CM. Single-transducer dual-frequency ultrasound generation to enhance acoustic cavitation. Ultrason Sonochem 2009; 16: 431–438
- Saletes I, Gilles B, Bera JC. Promoting inertial cavitation by nonlinear frequency mixing in a bifrequency focused ultrasound beam. Ultrasonics 2011; 51: 94–101
- Boyle P, Gould AL, Roehrborn CG. Prostate volume predicts outcome of treatment of benign prostatic hyperplasia with finasteride: Meta-analysis of randomized clinical trials. Urology 1996; 48: 398–405
- Berryhill R, Jhaveri J, Yadav R, Leung R, Rao S, El-Hakim A, Tewari A. Robotic prostatectomy: A review of outcomes compared with laparoscopic and open approaches. Urology 2008; 72: 15–23
- Moule N, Hoskin PJ. Non-surgical treatment of localised prostate cancer. Surg Oncol 2009; 18: 255–267