Abstract
Purpose: Tight regulation of gene expression in the region where therapy is necessary and for the duration required to achieve a therapeutic effect and to minimise systemic toxicity is very important for clinical applications of gene therapy. Hyperthermia in combination with a temperature sensitive heat shock protein (Hsp70) promoter presents a unique approach allowing non-invasive spatio-temporal control of transgene expression. In this study we investigated the in vivo and ex vivo relationship between temperature and duration of thermal stress with respect to the resulting gene expression using an Arrhenius analysis.
Materials and methods: A transgenic mouse expressing the luciferase reporter gene under the transcriptional control of a thermosensitive promoter was used to assure identical genotype for in vivo (mouse leg) and ex vivo (bone marrow mononuclear and embryonic fibroblast cells) studies. The mouse leg and cells were heated at different temperatures and different exposure times. Bioluminescence imaging and in vitro enzymatic assay were used to measure the resulting transgene expression.
Results: We showed that temperature-induced Hsp70 promoter activation was modulated by both temperature as well as duration of hyperthermia. The relationship between temperature and duration of hyperthermia and the resulting reporter gene expression can be modelled by an Arrhenius analysis for both in vivo as well as ex vivo.
Conclusions: However, the increase in reporter gene expression after elevating the temperature of the thermal stress with 1°C is not comparable for in vivo and ex vivo situations. This information may be valuable for optimising clinical gene therapy protocols.
Introduction
Already since the first clinical trials, performed in the early nineties, gene therapy has been called a promising technique for curing diseases, either by repairing or replacing a defective gene or by expressing some therapeutic proteins. Autoimmune reactions and systemic toxicity in combination with low efficacy of gene delivery hamper the translation of this technique to daily clinical practice. Another key issue for application of gene therapy in the clinic is the requirement for a tight regulation of gene expression in the region where therapy is necessary and for the duration to achieve a therapeutic effect in order to minimise systemic toxicity. Several approaches for controlling gene expression have already been described in the literature Citation[1]. Tissue- or disease-specific promoters have been proposed for spatial control Citation[2], Citation[3] and small molecule-dependent gene switches can provide temporal control Citation[4–6]. Alternatively, external physical stimuli such as ionising radiation Citation[7] and hyperthermia Citation[8] offer a high spatial resolution and control over the time point of gene activation. Hyperthermia in combination with a temperature sensitive heat shock protein (HSP) promoter presents a unique approach allowing non-invasive spatio-temporal control of transgene activation Citation[9]. Recently, it was shown that magnetic resonance guided high-intensity focused ultrasound (MRgHIFU) allows for the accurate and precise, non-invasive in vivo local heating, and thus for local activation of gene expression Citation[10].
Inducible HSP promoters, particularly Hsp70, are promising for gene therapy because of their low basal and high induced activities. Several studies demonstrated that their activity is correlated with temperature and duration of hyperthermia in vitro Citation[11–14] as well as in vivo Citation[15–18]. A quantitative relationship between applied thermal stress and the level of transgene expression in time is essential for effective and safe application of thermally induced gene expression. By using the activity of the firefly luciferase (LucF) as a reporter of gene expression, Beckham et al. were the first to analyse in more detail the relationship between the level of LucF activity and the temperature and duration of hyperthermia in vitro Citation[19]. They investigated to what extent the Arrhenius relation can be used to describe the transgene expression controlled by the Hsp70 promoter. The Arrhenius equation is a well-known empirical relationship for describing the temperature dependence of thermally induced processes Citation[20], Citation[21]. The Arrhenius relationship is frequently used to describe the process of cell death with respect to temperature and duration of hyperthermia Citation[22], Citation[23], and also holds for HSP-controlled LucF expression after applying thermal stress Citation[19].
The observed correlation between HSP-dependent gene expression and thermal stress parameters might be advantageous for clinical applications of gene therapy. A quantitative model for temperature-induced gene expression could be used during several stages of the actual treatment. In the planning phase, the optimal heating strategy can be chosen depending on the treatment location and the desired level of therapeutic gene expression. During the treatment, continuous monitoring of the temperature using MR thermometry would give real-time information on the spatial distribution of the thermal dose, which correlates with the level of transgene expression. In addition, the real-time measured thermal dose distribution allows for the correction of spatio-temporal temperature variations during the treatment (e.g. due to heterogeneous tissue and high tissue blood flow) by dynamically adjusting the duration and/or temperature of hyperthermia. At the end of a treatment the efficacy of repeated treatments in one patient or treatments in different patients can be compared by normalising the spatio-temporal thermal dose distribution for each treatment.
The correct usage of a quantitative model for temperature-induced gene expression, such as the Arrhenius relationship, requires an in-depth analysis of its validity. Several studies showed that the optimal conditions for Hsp70 activation are different for in vivo and in vitro experiments Citation[9]. Furthermore, it is known from other studies that different tissues vary in thermal sensitivity and in their ability to induce Hsp70 protein expression following (thermal) stress Citation[24–26]. However, these studies did not investigate whether the Arrhenius relationship changed for the different tissues and environments. Therefore the aim of this study is to determine whether the Arrhenius relationship between thermal stress and reporter gene expression is valid for different tissues in different environments. For this purpose we used a transgenic mouse model expressing the LucF reporter gene under control of the murine Hspa1b promoter and cells isolated from the same mouse to ensure identical genotype Citation[27]. Bioluminescence imaging (BLI) and luciferase enzymatic assay allow the quantifying of the temporal distribution of luciferase activity, which correlates with the levels of Hsp70 transcriptional activation following thermal stress Citation[28], Citation[29]. Here, we demonstrate that the Arrhenius relation can be used to describe Hsp70 promoter-induced transgene expression after thermal stress ex vivo as well as in vivo. However, the increase in reporter gene expression after elevating the temperature of the thermal stress with 1°C is not comparable for in vivo and ex vivo situations.
Materials and methods
Animals
Mice were reared at University of Bordeaux transgenic facilities. Male homozygote transgenic mice (NLF-1) ages 15–22 weeks were used in this study. NLF-1 mice contain a transgene that allows firefly luciferase expression under control of the Hsp70 promoter 1B (Hspa1b) Citation[27]. Before the study the posterior part of the mice was shaved with a clipper and a depilatory cream to facilitate light propagation. All mice were tested with regard to their phenotype one week before the beginning of the heating protocol. For this purpose bioluminescence images were acquired to verify the presence of low light emission due to basal promoter activity (see below for details of the BLI protocol). All procedures were performed according to protocols approved by French law and the rules of animal care of the institute.
In vivo heating
In vivo heating was done in a large water bath (30 L) with automatic temperature regulation preventing fluctuations in temperature during the experiment. Water temperature was measured with a calibrated thermometer (±0.1°C; Luxtron, CA, USA). Before and during heating, mice were sedated with isoflurane (2% in air, AErane, Baxter, Maurepa, France). For applying the heat shock, the left leg was put in the water while the rest of the body was lying on isolation material to prevent heating of the mouse.
To assess the luciferase activity with respect to temperature and duration of hyperthermia, nine groups of four mice were heated at different constant temperatures (43°, 44° and 45°C) for three different exposure times (ranging between 1 and 32 min, depending on the chosen temperature). The indicated temperatures correspond to the water bath temperature. In a separate experiment the intradermal, subcutaneous and intramuscular temperatures of a mouse leg were measured with a fibre optic thermometer (Luxtron) during exposure to these water bath temperatures ().
Figure 1. Temperature profile in time measured with a fiber optic probe at three positions in mouse leg (intradermal, subcutaneously and intramuscular) during warm water bath heating at three different temperatures.
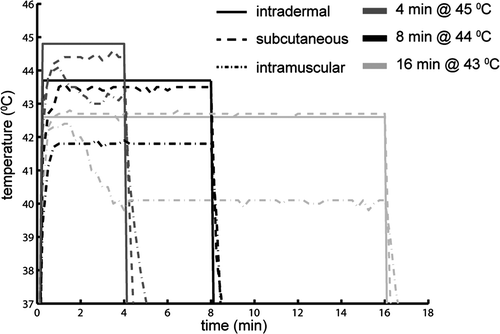
Note that we performed an Arrhenius analysis for Hsp70 promoter activity for temperatures of 43°C and above, both ex vivo as well as in vivo. From literature it is known that there exists a different temperature–time relationship for temperatures below 42°C Citation[30]. Lower temperature range would lead to very long heating durations before Hsp70 promoter induction is observed. For obtaining iso-effects for temperatures above 45°C, the duration of hyperthermia becomes very short, leading to more variations of the resulting light intensity.
In vivo BLI acquisition and analysis
To establish the time course of luciferase activity after applying different heating protocols, BLI was performed 5 times with 2 h intervals after applying the thermal stress. Bioluminescence images of mice were acquired using a NightOWL LB 981 system equipped with a NC 100 CCD camera (Berthold Technologies, Bad Wildbad, Germany). Mice were injected intraperitoneally with D-luciferin (Promega, Madison, WI, 2.9 mg in 100 µL sterile phosphate buffer (PBS, Invitrogen, Carlsbad, CA)). Two minutes later, mice were sedated with isoflurane (2% in air) and bioluminescence images (2 min integration period, 2 × 2 binning) were taken 7 and 10 min after the luciferin injection in prone and supine positions, respectively. A low light imaging standard (Glowell, LUX Biotechnology, Edinburgh) was placed next to the animal during each image acquisition to provide a constant reference for the resulting images.
Analysis of the BLI was done manually by placing a small region of interest (ROI) at the level of the knee of the heated leg (see ). This region was chosen because it does not suffer from hyper-intense light spots due to small skin wounds. Within this ROI, the mean light intensity (in photons s−1 mm−2) was measured using IndiGO® and WinLight® software (Berthold Technologies).
Figure 2. Bioluminescence image taken 4 hours after heating the right leg of NLF-1 mouse in a water bath. The colors show the light intensity measured with an optical camera from low intensity (violet) to high intensity (red). The position of the ROI used for quantification of the bioluminescence signal is also indicated.
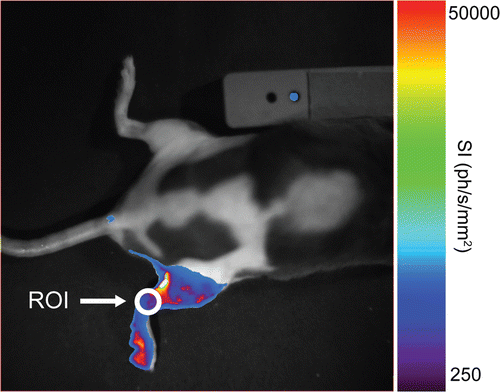
Control experiment: Source of in vivo bioluminescence
To determine the contribution of skin and muscle to the measured signal intensity during in vivo BLI acquisition some control experiments were performed. The back left leg of two mice was heated for 16 min at 43°C or for 4 min at 45°C using a warm water bath; 4 h after heating bioluminescence images were taken (using the protocol described above) before and after removing locally the skin of the heated leg to show the signal coming from the muscle.
Bone marrow mononuclear cell (BMMC) isolation
NLF-1 mice were anaesthetised (2% isoflurane in air) and euthanised (cervical dislocation). Bone marrow cells were flushed out of the femoral bone shafts with RPMI medium 1640 (Invitrogen) supplemented with 2% fetal calf serum (FCS) (Invitrogen). Bone marrow mononuclear cells were isolated on Ficoll (Eurobio, Courtaboeuf, France) by centrifugation (400 g, 20 min) and washed three times in RPMI medium 1640 plus 10% FCS (300 g, 5 min). Viable cells were counted with trypan blue exclusion dye and plated (1 × 105 cells/100 µL per tube) in RPMI medium 1640 supplemented with 10% FCS and mouse interleukin 3 (mIL-3; 5 ng/mL) (Immunotools, Friesoythe, Germany).
Mouse embryonic fibroblasts
Mouse embryonic fibroblasts (MEF) were isolated from E14.5 NLF-1 embryos. Pregnant NLF-1 mice were anaesthetised, euthanised, and uterine horns were dissected out and washed in PBS without calcium/magnesium (Invitrogen). Embryos were removed from uterine horns and separated from the placenta. Head, limbs and organs were cut away and embryos were washed in PBS without calcium/magnesium. Embryos were then minced and incubated in trypsine (1%, 2 mL/embryo) (Invitrogen) for 15 min at 37°C, with gentle shaking every 5 min. Trypsine activity was stopped with addition of 20 mL of DMEM (Invitrogen) supplemented with 10% FBS, and 1% of a penicillin, amphotericin and streptomycin mixture (PSA) (Invitrogen). Undigested embryo pieces settled in the bottom and supernatant was removed and centrifuged (250 g, 5 min). The cell pellet was resuspended in 10 mL of DMEM supplemented with 10% FBS, 1% PSA and seeded in a dish. About 2–3 embryos were pooled for one dish. The medium was changed the following day to eliminate non adherent cells. Cells were split at confluence in DMEM supplemented with 10% FBS, 1% PSA.
For experiments, MEF were plated in DMEM containing 10% FBS, 1% PSA, 2 days before heating in cell culture inserts for 12-well plates (BD Bioscience) at 2800 cells/cm2. All experiments in this study were performed in triplicate.
Ex vivo heating and luciferase activity measurement
BMMCs in suspension were heated immediately after isolation by using a thermal cycler (T gradient; Biometra, Göttingen, Germany) at different temperatures (43°, 44° and 45°C) and for different durations (ranging between 1 and 32 min) and finally incubated at 37°C in a 5% CO2 incubator for 5 h. The technical specifications of the thermal cycler assure a temperature precision of ±0.1°C, thermal homogeneity of 0.3°C within 15 s and changes in temperature of 4°C/s. Cells were washed twice in PBS, dried and frozen at −20°C before analysis.
MEF heating was performed with a water bath in combination with cell culture inserts for 12-well plates assuring a good heat transfer from the water bath to the cells. The temperatures in the water bath, the well plate and the inserts were verified using a calibrated thermometer (±0.1°C). Cell supernatant in the inserts was replaced by pre-heated medium. Next, the inserts were placed in the well-plates, which were incubated at different temperatures (43°, 44° and 45°C). Insert plus cells were kept at these temperatures for different durations (ranging between 2 and 16 min depending on the temperature). After heating, cells were incubated at 37°C and 5% CO2 for 5 h, washed twice with PBS, dried and frozen at −20°C before analysis.
The luciferase activity was measured using the luciferase assay system (Promega) according to the manufacturer's instructions. Briefly, cells were incubated in lysis buffer (15 min, room temperature) with shaking, and samples (10 µL) were mixed with luciferase assay substrate (LAS) (40 µL). Luminescence was measured 10 s after mixing for 10 s with a luminometer apparatus (Lumat, Berthold Technologies).
Arrhenius analysis
The Arrhenius equation gives the temperature dependence of the rate of different processes Citation[20], Citation[21]. The Arrhenius integral is commonly used to describe the measure of thermal damage/cell killing as a function of temperature and time of exposure:where Ω is the tissue damage, defined as the natural logarithm of a ratio of the concentration of native (undamaged) tissue before heating (C0) to the concentration of native tissue after heating (C(t)), A is the frequency factor (s−1), Ea is the activation energy (J mol−1), T is the temperature of exposure (K) and R is the universal gas constant (R = 8.32 J mol−1 K−1). For a constant temperature exposure the Arrhenius integral can be converted into a linear relationship for damage increase with increasing exposure time:
The linear relationship is clearly observed in an Arrhenius plot of ln(t)-ln(Ω) versus 1/T, wherein the activation energy and frequency factor can be determined from the slope and y-intercept of the linear fit, respectively.
The empirical relationship as described by the Arrhenius equation has been described mathematically as a relationship between time (t) and temperature (T):where K can be calculated as a function of activation energy and absolute temperature from an Arrhenius plot by:
Thus, for an iso-effect at various temperatures, a decrease of 1°C requires a hyperthermic exposure time increase by a factor K.
Historically Ω has been interpreted as a measure of thermal damage, however, in this study the temperature dependence of luciferase activity is investigated and is assumed to correlate with the HSP promoter activity and hence transgene expression. Therefore, Ω is interpreted as a measure of light emission (i.e. luciferase activity) and maximum light emission is chosen as an arbitrary end point corresponding to Ω = 1.
Results
Luciferase activity in vivo
For the in vivo study, transgenic mice (NLF-1) expressing the firefly luciferase under control of a mouse Hsp70 promoter (Hspa1b) were used. Mouse legs were heated in a water bath with different exposure times at three different constant temperatures. After heating, the time course of luciferase activity was acquired using BLI. In , the evolution in time of the measured light intensities for the different heating protocols is shown. Luciferase activity was already detected 2 h after heating and reached maximal intensity 4 h after heating. The time course of luciferase activity was similar for all combinations of temperature and time of hyperthermia. In contrast, the light emission levels changed according to the different heating protocols. At constant temperature the amount of light emission increased with increasing duration of hyperthermia (). A comparable increase of light emission was obtained by increasing temperature at a constant exposure time as shown in .
Figure 3. Time course of luciferase activity after heating a mouse leg with different heating protocols (A) 4, 8 and 16 min at 43°C, (B) 2, 4 and 8 min at 44°C, and (C) 1, 2 and 4 min at 45°C. The signal intensity (SI) is the average light intensity measured in the ROI, which is indicated in figure 2. The control measurement is done at the same position in the non-heated leg of the mice that were heated for the longest duration at each temperature.
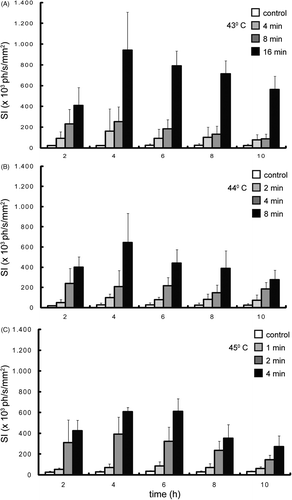
Figure 4. Time course of luciferase activity after heating a mouse leg. The data from figure 3 are rearranged in such a way that the luciferase activity is shown for constant heating duration (4 min) at 3 different temperatures. The signal intensity (SI) is the average light intensity measured in the ROI, which is indicated in figure 2. The control measurement is done at the same position in the non-heated leg of the mice that were heated at 45°C.
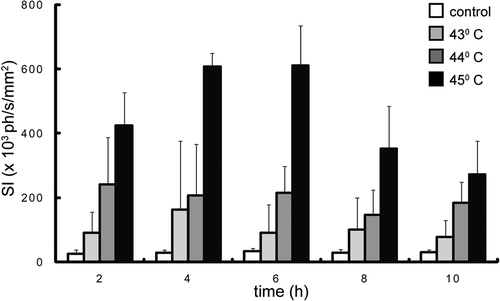
Control experiments showed that the muscle only contributes about 10% of the total signal intensity measured during in vivo BLI acquisitions ().
Figure 5. Bioluminescence image taken 4 hours after heating the left leg of NLF-1 mice for 16 min at 43°C (A, C) or 4 min at 45°C (B, D) in a water bath. In figures C and D the skin is locally removed (see inserts) to show the signal coming from the muscle. The numbers in figure indicate the average signal intensity (counts per second) measured in the ROIs as indicated in the figures. The colors show the light intensity measured with an optical camera from low intensity (violet) to high intensity (red).
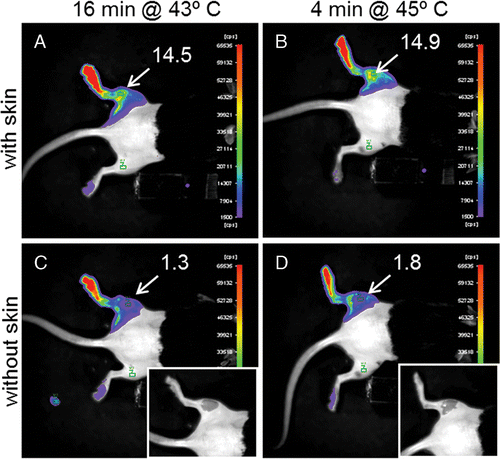
Arrhenius analysis in vivo
The in vivo light emission levels after heating, shown in , were used for an Arrhenius analysis. All temperature–time combinations were normalised to the maximum light intensity found at each time point. For all time points the maximum light emission was found after heating 16 min at 43°C, except at t = 2 h where the maximum light emission was found for the protocol of 4 min heating at 45°C. These maximum light emission data points correspond to Ω = 1. In , the natural logarithm of the normalised light intensity 4 h after heating is plotted as a function of the inverse of the absolute temperature. In this Arrhenius plot the solid line is a linear fit, with error weighting performed on three data points corresponding to 43°, 44° and 45°C. The correlation coefficient of the linear fit (r2) equalled 0.95, indicating that in vivo the luciferase activity follows an Arrhenius relationship in the temperature range 43–45°C. The activation energy (Ea), derived from the slope of the linear fit, is 481 kJ mol−1, which corresponds with a K-factor of 1.78 (Equation 4). Similar Arrhenius analyses were also performed for the other post-heating time points. The K-factor was fairly constant over time after heating with a mean value of 1.96 and a standard deviation of 0.17.
Figure 6. Arrhenius plot for constant-temperature in vivo water bath experiments 4 hours after heating. The error bars represent the standard deviation of the averages for each temperature. The solid line is a linear fit with error weighting performed on three data points, corresponding to 43, 44 and 45°C.
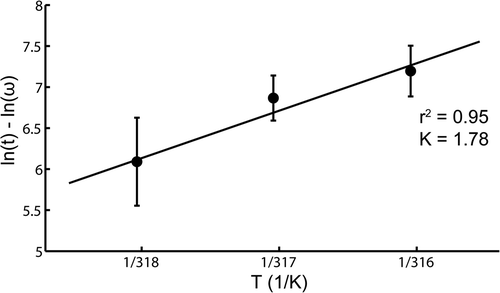
Arrhenius analysis ex vivo
Subsequently, the in vivo results of the Arrhenius analysis were compared to ex vivo results of an Arrhenius analysis performed with two different cell types originating from the NLF-1 mice thus exhibiting the same genomic background. For this purpose the luciferase activity was assessed with respect to temperature and duration of hyperthermia in BMMC obtained from the femoral bones of NLF-1 mice and in MEF obtained from NLF-1 embryos at E14.5. In the normalised natural logarithm of the measured light emission for both tissues are plotted as function of the inverse of the absolute temperature. The solid lines are linear fits with error weighting performed on three data points corresponding to 43°, 44° and 45°C. The correlation coefficient of the linear fits for the BMMC and MEF data were 0.99 and 0.92, respectively. This indicates that ex vivo Hsp70 expression in both BMMC as well as MEF follows an Arrhenius relationship in the temperature range 43–45°C. The activation energy of BMMC was 356 kJ mol−1, which corresponds to a K-factor of 1.53. In contrast, the activation energy of MEF was 845 kJ mol−1, leading to a K-factor of 2.75.
Figure 7. Arrhenius plot for constant-temperature ex vivo hyperthermia experiments 5 hours after heating. The solid line is a linear fit with error weighting performed on three data points, corresponding to 43, 44 and 45°C for the MEF. The dashed line is a linear fit with error weighting performed on three data points for BMM cells. The error bars represent the standard deviation of the averages for each temperature.
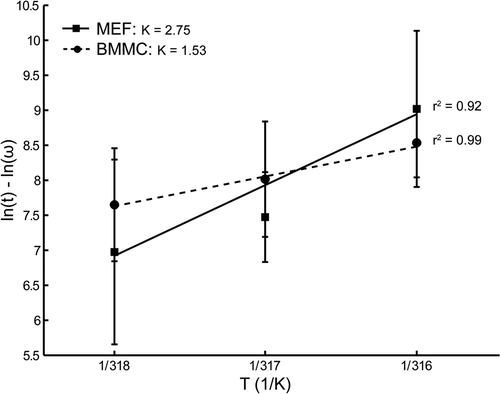
Discussion
HSP activation includes activation of the natural HSP promoter responsible for HSP protein synthesis and activation of experimental (added) HSP promoters responsible for transgene transcription. The resulting therapeutic/reporter protein concentration depends on HSP promoter activation, transcription of the transgene, translation into the therapeutic/reporter protein and subsequently to maturation and eventually therapeutic/reporter protein degradation. The combined effect of these mechanisms defines the half-life of the active protein. Because HSP proteins have a chaperone function, HSP proteins themselves may interfere with the therapeutic/reporter protein half-life. Note that firefly luciferase from the North American firefly (Photinus pyralis) shows a rapid maturation (no post-translational modification) and its half-life in eukaryotes cells is about 2 h Citation[31]. This may be completely different for other proteins.
In this study we evaluated the relationship between thermal stress and transgene expression for different cell preparations and compared these results with the relationship found in vivo in the same species. For this purpose we used a transgenic mouse model expressing the firefly luciferase reporter gene under control of an HSP promoter. By preparing cells from the transgenic mice tissues, we ensured a constant and reproducible genotype.
We showed that, under the conditions of heating by water bath, the skin is the major contributor to the in vivo signal detected by BLI as demonstrated by subsequent BLI data obtained with intact skin, and upon removal of skin (). Two factors contribute to this finding. Firstly, water bath heating leads to a negative temperature gradient from the skin. The muscle temperature was at least 2°C lower than the temperature of the skin (), leading to less promoter activation. Secondly, photons emitted from the skin are barely absorbed because of the short intra-tissue pathway in contrast to the photons coming from the muscle.
The in vivo time course of light emission after heating, which is assumed to be a measure for transgene expression Citation[28], Citation[29], was found to be transient with a maximum 4 h after heating. These results correspond with results found by other groups. Most studies that analysed the kinetics of Hsp70 promoter activity have been performed in cell cultures and the maximum activity for LucF was found in the range of 4–16 h after heating Citation[12], Citation[32], Citation[33]. Only recently the first in vivo studies analysing the Hsp70 activity kinetics were performed Citation[34], Citation[35]. Kruse et al. found the Hsp70 levels to peak 24–28 h after short duration, high intensity ultrasound exposures. The different nature of the applied heat shock (short duration, elevated temperature versus long duration, moderate temperature) may explain the discrepancy in time-to-peak with our study.
For a low temperature, long duration heating regime, we showed that the time-to-peak is independent of the chosen heating protocol. In contrast, Hundt et al. showed that the time-to peak increased with increasing heat shock temperature Citation[36]. This may be explained by the temperature zones introduced in the study by O’Connell-Rodwell et al., where a delayed time-to-peak was observed when cells were exposed to more severe heat shocks Citation[32].
Although the time-to-peak was independent of the chosen heating protocol, the in vivo HSP promoter activity was clearly modulated by both temperature as well as duration. This was already observed in other studies Citation[17], Citation[37]. The relation between promoter activity and thermal stress was analysed with an Arrhenius analysis. This mathematical description leads to the activation energy (Ea) of this thermally induced process. The average activation energy of the Hsp70 promoter found in the in vivo experiments was 559 kJ/mol. This activation energy leads to a K-factor of 1.96, indicating that an increase in temperature of 1°C is equivalent to decreasing the exposure time with a factor 1.96.
Similar activation energy values (140 kcal/mol ∼ 586 kJ/mol) for temperatures of 43°C and above were observed for thermal damage studies in vitro Citation[38], Citation[39], as well as in vivo Citation[40–43]. In addition, comparable activation energy values were found for thermal protein denaturation Citation[44], Citation[45]. Furthermore, Westra et al. and Li et al. observed similar activation energy values for induction of thermotolerance, for induction of Hsp70 synthesis and for cell killing Citation[39], Citation[46]. Some authors suggest that these activation energy values are rather similar because temperature-induced necrosis, induction of thermotolerance and induction of the Hsp70 promoter are all consequences of the same underlying process, i.e. detection of intracellular protein denaturation Citation[47], Citation[48].
However, the values for the activation energy found in our study for the BMMC (356 kJ/mol) and MEF (845 kJ/mol) ex vivo were significantly different from the in vivo value. This seems to be in contradiction to the large amount of data that indicates a fairly constant slope for different tissues in the Arrhenius plot above 43°C Citation[23], Citation[49]. However, in all these studies cell killing/tissue damage was used as the end point for the Arrhenius analysis. In contrast, in our study, HSP promoter activation was chosen as the end point for the Arrhenius analysis. HSP promoter activation precedes cell death and might, depending on the severity of the thermal shock, prevent cell death, which might explain the discrepancy Citation[33]. In addition, data from several papers that investigated heat-induced HSP expression in different tissues of different animals also suggest tissue-specific increase of HSP expression upon thermal exposure, though this was not discussed by the authors Citation[26], Citation[50].
Interestingly, independent of the activation energy values, the relation between temperature and duration of hyperthermia and the level of promoter activation/luciferase activity was well described by the Arrhenius equation for both the in vivo as well as the ex vivo situation, though with a different K-factor. This implies that if the activation energy is known, modulation of transgene expression by heating parameters could be predicted. Therefore, for clinical applicability, the key issue is to find a way to determine the specific activation energy (Ea) for the target tissue. Currently it is unknown whether Ea in vivo can be determined by experiments ex vivo. Our approach did not allow for comparison between activation energy value in vivo and ex vivo for a single tissue. Molecular imaging may provide a way to calculate Ea in vivo Citation[51].
Conclusion
In conclusion, we showed that thermally induced Hsp70 promoter activation was modulated by both temperature as well as duration of hyperthermia. The relationship between temperature and duration of hyperthermia and the resulting gene activity can be modelled by an Arrhenius analysis for both in vivo as well as ex vivo tissues. However, the increase in promoter activity after elevating the temperature of the thermal stress by 1°C is not comparable for in vivo and ex vivo tissues. Similar discrepancies were found for different ex vivo tissues. This is valuable information for optimising clinical gene therapy protocols.
Acknowledgements
The authors thank Josette Arsaut and Coralie Genevois for their help with the experiments.
Declaration of interest: The authors would also like to acknowledge the financial support of the Conseil Régional d’Aquitaine, InNaBioSante Foundation (project ULTRAFITT) and Diagnostic Molecular Imaging EC-FP6-project LSHB-CT-2005-512146. The authors alone are responsible for the content and writing of the paper.
References
- Vilaboa N, Voellmy R. Regulatable gene expression systems for gene therapy. Curr Gene Ther 2006; 6: 421–438
- Gorski K, Carneiro M, Schibler U. Tissue-specific in vitro transcription from the mouse albumin promoter. Cell 1986; 47: 767–776
- Melo LG, Gnecchi M, Pachori AS, Kong D, Wang K, Liu X, Pratt RE, Dzau VJ. Endothelium-targeted gene and cell-based therapies for cardiovascular disease. Arterioscler Thromb Vasc Biol 2004; 24: 1761–1774
- Gossen M, Bujard H. Tight control of gene expression in mammalian cells by tetracycline-responsive promoters. Proc Natl Acad Sci USA 1992; 89: 5547–5551
- Rivera VM, Clackson T, Natesan S, Pollock R, Amara JF, Keenan T, et al. A humanized system for pharmacologic control of gene expression. Nat Med 1996; 2: 1028–1032
- Wang Y, O’Malley BW, Jr, Tsai SY, O’Malley BW. A regulatory system for use in gene transfer. Proc Natl Acad Sci USA 1994; 91: 8180–8184
- Hallahan DE, Mauceri HJ, Seung LP, Dunphy EJ, Wayne JD, Hanna NN, et al. Spatial and temporal control of gene therapy using ionizing radiation. Nat Med 1995; 1: 786–791
- Madio DP, van Gelderen P, DesPres D, Olson AW, de Zwart JA, Fawcett TW, et al. On the feasibility of MRI-guided focused ultrasound for local induction of gene expression. J Magn Reson Imaging 1998; 8: 101–104
- Vekris A, Maurange C, Moonen C, Mazurier F, De Verneuil H, Canioni P, et al. Control of transgene expression using local hyperthermia in combination with a heat-sensitive promoter. J Gene Med 2000; 2: 89–96
- Deckers R, Quesson B, Arsaut J, Eimer S, Couillaud F, Moonen CT. Image-guided, noninvasive, spatiotemporal control of gene expression. Proc Natl Acad Sci USA 2009; 106: 1175–1180
- Borrelli MJ, Schoenherr DM, Wong A, Bernock LJ, Corry PM. Heat-activated transgene expression from adenovirus vectors infected into human prostate cancer cells. Cancer Res 2001; 61: 1113–1121
- Gerner EW, Hersh EM, Pennington M, Tsang TC, Harris D, Vasanwala F, et al. Heat-inducible vectors for use in gene therapy. Int J Hyperthermia 2000; 16: 171–181
- Huang Q, Hu JK, Lohr F, Zhang L, Braun R, Lanzen J, et al. Heat-induced gene expression as a novel targeted cancer gene therapy strategy. Cancer Res 2000; 60: 3435–3439
- Smith RC, Machluf M, Bromley P, Atala A, Walsh K. Spatial and temporal control of transgene expression through ultrasound-mediated induction of the heat shock protein 70B promoter in vivo. Hum Gene Ther 2002; 13: 697–706
- Guilhon E, Voisin P, de Zwart JA, Quesson B, Salomir R, Maurange C, et al. Spatial and temporal control of transgene expression in vivo using a heat-sensitive promoter and MRI-guided focused ultrasound. J Gene Med 2003; 5: 333–342
- Plathow C, Lohr F, Divkovic G, Rademaker G, Farhan N, Peschke P, et al. Focal gene induction in the liver of rats by a heat-inducible promoter using focused ultrasound hyperthermia: Preliminary results. Invest Radiol 2005; 40: 729–735
- Silcox CE, Smith RC, King R, McDannold N, Bromley P, Walsh K, et al. MRI-guided ultrasonic heating allows spatial control of exogenous luciferase in canine prostate. Ultrasound Med Biol 2005; 31: 965–970
- Xu L, Zhao Y, Zhang Q, Li Y, Xu Y. Regulation of transgene expression in muscles by ultrasound-mediated hyperthermia. Gene Ther 2004; 11: 894–900
- Beckham JT, Mackanos MA, Crooke C, Takahashi T, O’Connell-Rodwell C, Contag CH, et al. Assessment of cellular response to thermal laser injury through bioluminescence imaging of heat shock protein 70. Photochem Photobiol 2004; 79: 76–85
- Arrhenius S. On the reaction rate of the inversion of non-refined sugar upon souring. Z Phys Chem 1889;4:226–248.
- Stiller W. Arrhenius equation and non-equilibrium kinetics. Teubner Texte zur Physik B21, Leipzig 1989
- Henriques FC, Moritz AR. Studies of thermal injury: I. The conduction of heat to and through skin and the temperatures attained therein. A theoretical and an experimental investigation. Am J Pathol 1947; 23: 530–549
- Yarmolenko PS, Moon EJ, Landon C, Manzoor A, Hochman DW, Viglianti BL, Dewhirst MW. Thresholds for thermal damage to normal tissues: An update. Int J Hyperthermia 2011; 27: 320–343
- Flanagan SW, Ryan AJ, Gisolfi CV, Moseley PL. Tissue-specific HSP70 response in animals undergoing heat stress. Am J Physiol 1995; 268: R28–32
- Manzerra P, Rush SJ, Brown IR. Tissue-specific differences in heat shock protein Hsc70 and Hsp70 in the control and hyperthermic rabbit. J Cell Physiol 1997; 170: 130–137
- Ruell PA, Hoffman KM, Chow CM, Thompson MW. Effect of temperature and duration of hyperthermia on Hsp72 induction in rat tissues. Mol Cell Biochem. 2004; 267: 187–194
- Christians E, Campion E, Thompson EM, Renard JP. Expression of the Hsp70.1 gene, a landmark of early zygotic activity in the mouse embryo, is restricted to the first burst of transcription. Development 1995; 121: 113–122
- Brasier AR, Tate JE, Habener JF. Optimized use of the firefly luciferase assay as a reporter gene in mammalian cell lines. Biotechniques 1989; 7: 1116–1122
- Contag CH, Spilman SD, Contag PR, Oshiro M, Eames B, Dennery P, et al. Visualizing gene expression in living mammals using a bioluminescent reporter. Photochem Photobiol 1997; 66: 523–531
- Dewey WC. Arrhenius relationships from the molecule and cell to the clinic. Int J Hyperthermia 1994; 10: 457–483
- Gheysens O, Mottaghy FM. Method of bioluminescence imaging for molecular imaging of physiological and pathological processes. Methods 2009; 48: 139–145
- O’Connell-Rodwell CE, Shriver D, Simanovskii DM, McClure C, Cao YA, Zhang W, et al. A genetic reporter of thermal stress defines physiologic zones over a defined temperature range. FASEB J 2004; 18: 264–271
- Rylander MN, Feng Y, Zimmermann K, Diller KR. Measurement and mathematical modeling of thermally induced injury and heat shock protein expression kinetics in normal and cancerous prostate cells. Int J Hyperthermia 2010; 26: 748–764
- Kruse DE, Mackanos MA, O’Connell-Rodwell CE, Contag CH, Ferrara KW. Short-duration-focused ultrasound stimulation of Hsp70 expression in vivo. Phys Med Biol 2008; 53: 3641–3660
- O’Connell-Rodwell CE, Mackanos MA, Simanovskii D, Cao YA, Bachmann MH, Schwettman HA, et al. In vivo analysis of heat-shock-protein-70 induction following pulsed laser irradiation in a transgenic reporter mouse. J Biomed Opt 2008; 13: 030501
- Hundt W, O’Connell-Rodwell CE, Bednarski MD, Steinbach S, Guccione S. In vitro effect of focused ultrasound or thermal stress on Hsp70 expression and cell viability in three tumor cell lines. Acad Radiol 2007; 14: 859–870
- Liu Y, Kon T, Li C, Zhong P. High intensity focused ultrasound-induced gene activation in solid tumors. J Acoust Soc Am 2006; 120: 492–501
- Harris M. Temperature-resistant variants in clonal populations of pig kidney cells. Exp Cell Res 1967; 46: 301–314
- Westra A, Dewey WC. Variation in sensitivity to heat shock during the cell-cycle of Chinese hamster cells in vitro. Int J Radiat Biol Relat Stud Phys Chem Med 1971; 19: 467–477
- Crile G, Jr. The effects of heat and radiation on cancers implanted on the feet of mice. Cancer Res 1963; 23: 372–380
- Morris CC, Field SB. The relationship between heating time and temperature for rat tail necrosis with and without occlusion of the blood supply. Int J Radiat Biol Relat Stud Phys Chem Med 1985; 47: 41–48
- Okumura Y, Reinhold HS. Heat sensitivity of rat skin. Eur J Cancer 1978; 14: 1161–1166
- Overgaard J, Suit HD. Time–temperature relationship th hyperthermic treatment of malignant and normal tissue in vivo. Cancer Res 1979; 39: 3248–3253
- Dewey WC, Freeman ML, Raaphorst GP, Clark EP, Wong RSL, Highfield DP, . Cell biology of hyperthermia and radiation. Radiation Biology in Cancer Research, RMaHR Withers, et al. Raven Press, New York 1980
- Dewey WC, Hopwood LE, Sapareto SA, Gerweck LE. Cellular responses to combinations of hyperthermia and radiation. Radiology 1977; 123: 463–474
- Li GC, Fisher GA, Hahn GM. Induction of thermotolerance and evidence for a well-defined, thermotropic cooperative process. Radiat Res 1982; 89: 361–368
- Freeman BC, Michels A, Song J, Kampinga HH, Morimoto RI. Analysis of molecular chaperone activities using in vitro and in vivo approaches. Methods Mol Biol 2000; 99: 393–419
- Kiang JG, Tsokos GC. Heat shock protein 70 kDa: Molecular biology, biochemistry, and physiology. Pharmacol Ther 1998; 80: 183–201
- Dewhirst MW, Viglianti BL, Lora-Michiels M, Hanson M, Hoopes PJ. Basic principles of thermal dosimetry and thermal thresholds for tissue damage from hyperthermia. Int J Hyperthermia 2003; 19: 267–294
- Piano A, Asirelli C, Caselli F, Fabbri E. Hsp70 expression in thermally stressed Ostrea edulis, a commercially important oyster in Europe. Cell Stress Chaperones 2002; 7: 250–257
- Winkeler A, Sena-Esteves M, Paulis LE, Li H, Waerzeggers Y, Ruckriem B, Himmelreich U, et al. Switching on the lights for gene therapy. PLoS One 2007; 2: e528