Abstract
Purpose: Heat stress induces complex cellular responses, and its detailed molecular mechanisms still remain to be clarified. The objective of this study was to investigate the molecular mechanisms underlying cellular responses to mild hyperthermia (MHT) in normal human fibroblastic (NHF) cells.
Materials and methods: Cells were treated with MHT (41°C, 30 min) and then cultured at 37°C. Gene expression was determined by the GeneChip® system and bioinformatics tools.
Results: Treatment of the NHF cell lines, Hs68 and OUMS-36, with MHT did not affect the cell viability or cell cycle. In contrast, many probe sets were differentially expressed by >1.5-fold in both cell lines after MHT treatment. Of the 1,196 commonly and differentially expressed probe sets analysed by k-means clustering, three gene clusters, Up-I, Down-I and Down-II, were observed. Interestingly, two gene networks were obtained from the up-regulated genes in cluster Up-I. The gene network E contained DDIT3 and HSPA5 and was mainly associated with the biological process of endoplasmic reticulum stress, while the network S contained HBEGF and LIF and was associated with the biological process of cell survival. Eighteen genes were validated by quantitative real-time polymerase chain reaction, consistent with the microarray data, in four kinds of NHF cells.
Conclusions: Common genes that were differentially expressed and/or acted within a gene network in response to MHT in NHF cells were identified. These findings provide the molecular basis for a further understanding of the mechanisms of the MHT response in NHF cells.
Introduction
Hyperthermia (HT) induced by heat stress in the range of 40–45°C has been used as a possible mode of cancer treatment and its pleiotropic effects argue for its combined use with already established strategies, especially radiotherapy and/or chemotherapy Citation[1–6]. In general, cancer cells exposed to temperatures of more than 42.5°C, the breakpoint of HT, will undergo cell death with increasing temperature, whereas temperatures up to 42.5°C, in the mild hyperthermia (MHT) range, induce only slight or no cytotoxicity Citation[7]. However, it is very difficult to restrict the application of heat to the cancer itself and avoid damaging the surrounding normal tissue Citation[8]. It has also been suggested that HT monotherapy maintaining a cancer temperature above 42.5°C for direct cytotoxicity has been associated with higher toxicity and less efficacy against cancer. Moreover, in accordance with the close relationship established between cancer and immune reactions, recent findings have suggested that an anti-tumour immune system including heat shock protein (HSP) chaperone tumour antigens might be effective as an HT-based cancer immunotherapy Citation[9], Citation[10]. Therefore, MHT in combination with radiotherapy and/or chemotherapy have been used for various cancers, and the anticancer effects of such combinations have been verified in many clinical trials Citation[1–6].
The heat shock response is an ancient and evolutionally conserved protective reaction to elevated temperatures. Heat induces a wide spectrum of stress responses, such as an induction of HSPs, protein aggregation, DNA and RNA damage, an imbalance of protein homeostasis, reactive oxygen species production, cell growth arrest and different types of cell death, including apoptosis Citation[11], Citation[12]. HSPs, which are especially induced by heat, behave as molecular chaperones for other cellular proteins Citation[11]. From the point of view of cancer cell death, HSPs function as strong cytoprotective molecules preventing apoptosis and other types of cell death and participate in thermotolerance Citation[13–15]. HSPs achieve these effects by interacting with components of the cell signalling pathway, such as caspase-dependent apoptosis, upstream and downstream of the mitochondrial level Citation[16]. Furthermore, heat activates extracellular signal-regulated kinases, and this activation provides cytoprotection from cell death by heating Citation[17]. On the other hand, HT has been reported to induce apoptosis via activation of the protein kinase c-Jun N-terminal kinase Citation[18], Citation[19]. More recently, MHT was reported to activate the endoplasmic reticulum (ER) stress pathway in mammalian cells Citation[20], Citation[21].
Recent microarray technology coupled with bioinformatics tools has provided a view of the genome-wide expression profiles, and the relevant biological functions and gene networks based on the gene-expression data. It has been reported that heat stresses including MHT and HT affect the expression pattern of genes and a variety of biological functions in normal cells Citation[14], Citation[22–26] and cancer cells Citation[24], Citation[27–34]. Because heat stress induces complex cellular responses and changes in signal transduction, the precise mechanisms of heat response have not been elucidated.
As described above, MHT-based cancer therapy has been regarded as an effective cancer treatment with relatively few side effects. However, the temperature of the surrounding normal tissue of the tumour is also elevated to within the MHT range. Therefore, it is very important to understand the heat responses to normal cells in addition to cancer cells. Previously, we reported differentially expressed genes and gene networks in MHT-treated human leukaemia U937 cells Citation[32], Citation[33] or human oral squamous cell carcinoma HSC-3 cells Citation[34]. The objective of this study was to investigate the molecular mechanisms underlying cellular responses to MHT in normal human fibroblastic (NHF) cells. To assess the global-scale gene expression profiles we employed high-density oligonucleotide microarrays and computational gene expression analysis tools in two kinds of NHF cells, Hs68 and OUMS-36, responsive to MHT at 41°C. Moreover, a real-time quantitative polymerase chain reaction (qPCR) assay was performed to identify the common expression patterns responsive to MHT in two other NHF cell types, KD and NTI-4, as well as Hs68 and OUMS-36 cells.
Materials and methods
Cell culture
Human fibroblastic cell lines, Hs68 (derived from newborn foreskin), KD (lip), NTI-4 (foetus body) and OUMS-36 (embryo), were obtained from the Human Science Research Resources Bank, Japan Health Sciences Foundation (Tokyo, Japan). These cells were cultured in Dulbecco's modified Eagle medium (Life Technologies, Grand Island, NY, USA) supplemented with 10% foetal bovine serum (Equitech-Bio, Kerrville, TX, USA) at 37°C in humidified air with 5% CO2 and 95% air.
Heat treatment
Heat treatment was performed by immersing plastic vessels containing the attached cells in a water bath at 41°C (±0.05°C) or 44°C (±0.05°C) for 30 min, respectively. The temperature was monitored with a digital thermometer (No. 7563, Yokogawa, Tokyo) during heating. After heat treatment, the cells were incubated for 0–24 h at 37°C.
Analyses of cell viability and cell cycle
For cell viability, the trypan blue dye exclusion test was performed. The number of cells excluding the dye was counted by using a haemocytometer. The cell cycle was determined by flow cytometry as described previously Citation[35]. In brief, for detection of the cell cycle, cells were fixed with 70% ice-cold ethanol, and then treated with RNase A (Nacalai Tesque, Kyoto, Japan) and propidium iodide (Wako Pure Chemical Industries, Osaka, Japan). The samples were run on an Epics XL flow cytometer (Beckman Coulter, Fullerton, CA, USA).
RNA isolation
Total RNA was extracted from cells using an RNeasy Total RNA Extraction Kit (Qiagen, Valencia, CA) along with on-column DNase I treatment (RNase-free DNase kit, Qiagen). RNA quality was analysed using a Bioanalyzer 2100 (Agilent Technologies, Santa Clara, CA). RNA samples that had RIN (RNA integrity number) values above 9.5 were considered acceptable.
Microarray gene expression analysis
Microarray gene expression analysis was performed using a GeneChip® system with a Human Genome U133-plus 2.0 array, which was spotted with 54,675 probe sets (Affymetrix, Santa Clara) according to the manufacturer's instructions. In short, 500 ng of total RNA was used to synthesise cRNA with a GeneChip® 3’ IVT Express Kit (Affymetrix). After fragmentation, biotin-labelled cRNA was hybridised to the array at 45°C for 16 h. The arrays were washed, stained with streptavidin-phycoerythrin, and scanned using a probe array scanner. The obtained hybridisation intensity data were analysed using GeneSpring® GX (Agilent) to extract the significant genes. To examine gene ontology, including biological processes, cellular components, molecular functions, and gene networks, the obtained data were analysed using Ingenuity® Pathway Analysis tools (Ingenuity Systems, Mountain View, CA), a web-delivered application that enables the identification, visualisation, and exploration of molecular interaction networks in gene expression data Citation[36], Citation[37].
Real-time qPCR assay
Real-time qPCR was performed on an Mx3005P real-time PCR system (Agilent) using SYBR PreMix ExTaq (Takara Bio, Shiga, Japan) or Premix Ex Taq (for the use of TaqMan probes, Takara Bio) according to the manufacturer's protocols. The reverse transcriptase reaction was carried out with total RNA by using a random 6 mers and an oligo dT primer (PrimeScript RT Reagent Kit; Takara Bio). Real-time qPCR was performed by using the specific primers and probes listed in and of the supplementary materials. Each mRNA expression level was normalised with respect to the mRNA expression of glyceraldehyde 3-phosphate dehydrogenase (GAPDH).
Table I. The top 40 genes in the cluster Up-I.
Table II. The top 20 genes in the cluster Down-I.
Statistical analysis
Data are shown as the means ± SD. Student's t-test was used for statistical analysis and significance was assumed for p values <0.05.
Results
Effects of heat on cell viability and cell cycle in NHF Hs68 and OUMS-36 cells
We used a trypan blue dye exclusion test for the detection of cell viability. When Hs68 and OUMS-36 cells were treated with MHT at 41°C for 30 min followed by culture at 37°C for 24 h, the percentage of viable cells did not change compared to the control (37°C treatment) (). On the other hand, HT at 44°C for 30 min significantly decreased cell viability, to levels of 78.4 ± 11.2% and 74.8 ± 11.3% (mean ± SD; each control = 100%) for Hs68 and OUMS-36 cells, respectively (). In addition, the cell cycle distribution was monitored by propidium iodide staining with flow cytometry. As shown in , the percentages of control Hs68 or OUMS-36 cells in the G0/G1, S, and G2/M phases were 78.1 ± 0.6%, 9.3 ± 0.3%, and 12.6 ± 0.7% or 63.0 ± 8.3%, 19.3 ± 4.3%, and 17.7 ± 4.0% (mean ± SD), respectively. Although changes in the distribution of cell cycle phases were not observed in the Hs68 or OUMS-36 cells treated with MHT at 41°C for 30 min, HT at 44°C for 30 min could arrest cells of either type in the G2/M phase (). These data indicated that no cell damage and no cell cycle arrest was present in the cells treated with MHT at 41°C for 30 min.
Figure 1. The effects of heat on cell viability and the cell cycle in two NHF cell lines, Hs68 and OUMS-36. Cells were exposed to MHT at 41°C for 30 min or HT at 44°C for 30 min. After heat treatment, the cells were incubated for 24 h at 37°C. The cell viability (A, B) and cell cycle (C, D) were measured. (A, C) Hs68 cells. (B, D) OUMS-36 cells. Data indicate the means ± SD for four different experiments. *P < 0.05 versus the control (37°C treatment).
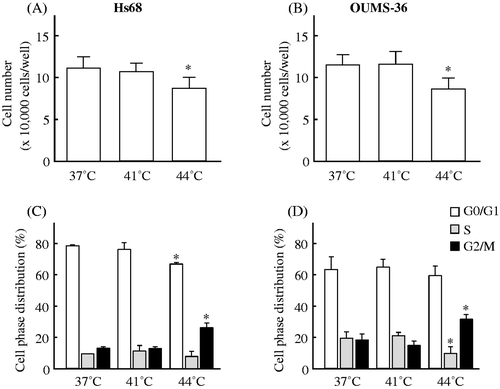
Global gene expression and cluster analyses
Global-scale gene expression analysis was carried out using a GeneChip® system with a Human Genome U133-plus 2.0 array, which was s potted with 54,675 probe sets. We detected 15,704 (28.7%) and 15,436 (28.2%) probe sets that were expressed in Hs68 and OUMS-36 cells subjected to MHT treatment, respectively. The complete lists of genes from Hs68 and OUMS-36 cell samples have been deposited in the Gene Expression Omnibus (GEO), a public database (accession numbers: GSE39177 and GSE39178). Expression analysis using GeneSpring® software of cells treated with MHT demonstrated many probe sets that were differentially regulated by a factor of 1.5 or greater. The Venn diagram in summarises the numbers of specifically and commonly expressed probe sets affected by MHT at 41°C for 30 min in Hs68 and OUMS-36 cells. In all, 2,567 and 2,169 probe sets were found to be differentially expressed in Hs68 and OUMS-36 cells, respectively, and 1,196 probe sets were differentially expressed in both cell lines (). Moreover, k-means clustering, a non-hierarchical gene clustering algorithm included in the GeneSpring® software package, was performed to generate the major patterns of gene expression during MHT treatment. In both cell lines, probe sets were grouped in three clusters with distinct expression profiles, designated as Up-I, Down-I and Down-II. The expression levels of probe sets in the Up-I cluster were gradually increased and this cluster also contained increased probe sets whose peak expressions occurred at 0 and 1 h; the expression levels of probe sets in the Down-I cluster were gradually decreased and this cluster also contained decreased probe sets whose lowest expression was at 1 h; the Down-II cluster contained transiently decreased probe sets whose lowest expression was at 1 h. The numbers of commonly expressed probe sets and genes in the Up-I, Down-I or Down-II cluster were 201 and 168, 493 and 443 or 283 and 238, respectively (). The top 40, 20 and 20 of genes in the Up-I, Down-I and Down-II clusters are shown in , and , respectively.
Figure 2. Gene expression analysis. Gene expression analysis of the probe sets that were differentially expressed by a factor of 1.5 or greater in Hs68 and OUMS-36 cells treated with MHT at 41°C for 30 min was performed using bioinformatics analysis tools. (A) Venn diagram of the probe sets that were differentially expressed in Hs68 and OUMS-36 cells. The numbers of probe sets are shown. (B) K-means clustering of commonly and differentially expressed probe sets (1,196 probe sets). Probe sets were grouped in three clusters with distinct expression profiles, Up-I, Down-I and Down-II. The numbers of commonly expressed probe sets and genes are shown. C, control.
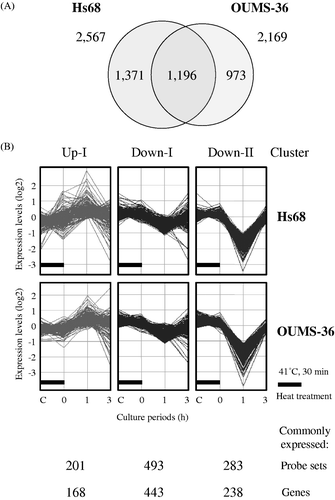
Table III. The top 20 of genes in the cluster Down-II.
Identification of biological functions and gene networks
Functional category and gene network analyses of commonly and differentially expressed genes induced by MHT were conducted by using the Ingenuity® Pathways Knowledge Base. The top three biological functions at each cluster are summarised in of the supplementary materials. Three biological functions, i.e. cellular compromise, cellular function and maintenance, and cellular growth and proliferation, were observed in the up-regulated gene cluster Up-I. In the down-regulated gene cluster Down-I, the biological functions were determined to be gene expression, cell death, and cellular compromise. In addition, three biological functions, i.e. cell cycle, gene expression, and DNA replication, recombination and repair, were observed in gene cluster Down-II. In addition, two significant gene networks, E and S, that were obtained from up-regulated genes in the Up-I cluster were identified (). The detailed time-course of changes in the expression levels of genes for each gene network in Hs68 and OUMS-36 cells is shown in and of the supplementary materials. The gene network E contained many HSPs, DNA-damage-inducible transcript 3 (DDIT3), homocysteine-inducible, endoplasmic reticulum stress-inducible, ubiquitin-like domain member 1 (HERPUD1), and Hsp70 kDa protein 5 (glucose-regulated protein, 78 kDa) (HSPA5), and was mainly associated with ER stress (functions annotation) in the category of biological functions (cellular compromise or cellular function and maintenance). The gene network S contained endothelin 1 (EDN1), heparin-binding EGF-like growth factor (HBEGF), and leukaemia inhibitory factor (LIF), and was mainly associated with cell survival (functions annotation) in the category of biological functions (cell death) ().
Figure 3. Gene networks. Up-regulated genes in the cluster Up-I were analysed by Ingenuity® Pathways Analysis tools. Gene networks E (A) and S (B) associated with ER stress and cell survival, respectively, were identified. The network is displayed graphically as nodes (genes) and edges (the biological relationships between the nodes). The node colour of genes indicates the expression level of genes. Nodes and edges are displayed using various shapes and labels that present the functional class of genes and the nature of the relationship between the nodes, respectively.
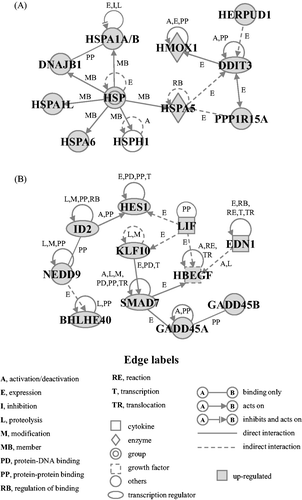
Quantitative analysis of differentially expressed genes
To verify the alterations observed by the microarray analysis, we used two NHF cell lines, KD and NTI-4, in addition to Hs68 and OUMS-36 cells. Time-dependent changes in the gene expression levels of selected genes that belonged to the gene networks E and S were monitored by using a real-time qPCR. The expression levels of all 10 genes in the gene network E, DDIT3, DnaJ (Hsp40) homolog, subfamily B, member 1 (DNAJB1), HERPUD1, haem oxygenase (decycling) 1 (HMOX1), Hsp70 kDa protein 1 A/B (HSPA1A/B), Hsp70 kDa protein 1-like (HSPA1L), HSPA5, Hsp70 kDa protein 6 (Hsp70B') (HSPA6), heat shock 105 kDa/110 kDa protein 1 (HSPH1), and protein phosphatase 1, regulatory subunit 15 A (PPP1R15A), were significantly increased in the Hs68 and OUMS-36 cells treated with MHT. These elevations were observed in the KD and NTI-4 cells treated with MHT (). The expression levels of all 11 genes in the gene network S, basic helix-loop-helix family, member e40 (BHLHE40), EDN1, growth arrest and DNA-damage-inducible, alpha (GADD45A), growth arrest and DNA-damage-inducible, beta (GADD45B), HBEGF, hairy and enhancer of split 1, (Drosophila) (HES1), inhibitor of DNA binding 2, dominant negative helix-loop-helix protein (ID2), Kruppel-like factor 10 (KLF10), LIF, neural precursor cell expressed, developmentally down-regulated 9 (NEDD9), and SMAD family member 7 (SMAD7), were significantly increased in the Hs68 and OUMS-36 cells treated with MHT. The expression levels of eight of the 11 genes, with the exception being HES1, ID2 and KLF10, were significantly elevated in both KD and NTI-4 cells undergoing MHT treatment. On the other hand, increases in the expression of ID2 and KLF10 were detected in the NTI-4 and KD cells, respectively. A significant elevation of the expression of HES1 did not observed in the KD and NTI-4 cells (). Taken together, the microarray results for all 21 genes in these networks were comparable to those from real-time qPCR analysis.
Figure 4. Effects of MHT on the time-course of changes in the expression levels of genes in the gene network E. Cells of the NHF lines Hs68 (Hs), KD, NTI-4 (NTI) and OUMS-36 (OUMS) were incubated at 41°C for 30 min and then cultured at 37°C for 0, 1 and 3 h. Real-time qPCR was carried out. DDIT3 (A), DNAJB1 (B), HERPUD1 (C), HMOX1 (D), HSPA1A/B (E), HSPA1L (F), HSPA5 (G), HSPA6 (H), HSPH1 (I) and PPP1R15A (J) were included in the gene network E. The expression level of each mRNA was normalised to the GAPDH expression level. Data are presented as the mean ± SD (n = 4). *P < 0.05 versus the control.
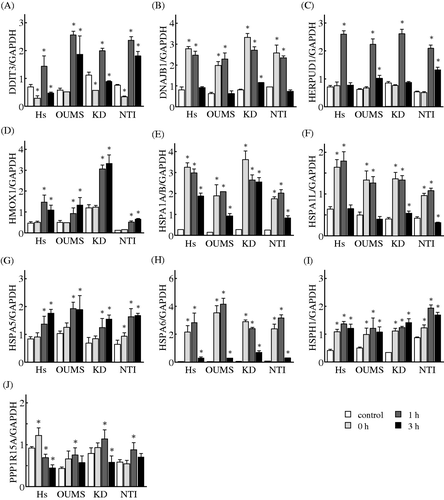
Figure 5. Effects of MHT on the time-course of changes in the expression levels of genes in the gene network S. Cells of the NHF cells Hs68 (Hs), KD, NTI-4 (NTI) and OUMS-36 (OUMS) were incubated at 41°C for 30 min and then cultured at 37°C for 0, 1 and 3 h. Real-time qPCR was carried out. BHLHE40 (A), EDN1 (B), GADD45A (C), GADD45B (D), HBEGF (E), HES1 (F), ID2 (G), KLF10 (H), LIF (I), NEDD9 (J) and SMAD7 (K) were included in the gene network S. The expression level of each mRNA was normalised to the GAPDH expression level. Data are presented as the mean ± SD (n = 4). *P < 0.05 versus the control.
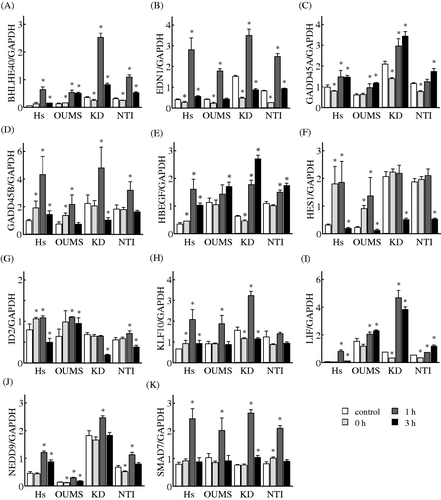
Discussion
The heat shock response, which is a universal cellular response to heat, is a very important cellular event for cell adaptation. Because of the complex cellular response elicited by heat, we hypothesised that the most powerful technologies currently available for elucidating heat responses were global-scale microarrays and bioinformatics tools. Our approach with these technologies is the first to demonstrate the common genes and gene networks in response to MHT in NHF cells.
In the present study, MHT at 41°C did not affect cell viability or the cell cycle. However, a global-scale microarray clearly showed that many genes were differentially expressed by >1.5-fold in both cell lines, Hs68 and OUMS-36, after MHT treatment. This finding was in good agreement with previous results in MHT conditions Citation[24], Citation[31–34] and HT at relatively high temperature Citation[14], Citation[22], Citation[23], Citation[25–31], Citation[33], Citation[34] in normal cells Citation[14], Citation[22–26] and cancer cells Citation[24], Citation[27–34]. Here, computational bioinformatics tools were used to explore common responses to MHT. Approximately 50% of the probe sets were found to be commonly and differentially expressed probe sets between Hs68 and OUMS-36 cells, and these could be grouped in three clusters with distinct expression profiles, Up-I, Down-I and Down-II. Numerous genes in the clusters of Down-I and Down-II were transiently decreased at 1 h post-MHT treatment, but their expression levels were recovered immediately (). In mammalian cells, heat activates transcription of heat responsive genes, including HSPs, coincident with a bulk decrease in mRNA and protein syntheses, and this overall reprogramming of gene expression permits the selective synthesis of HSPs Citation[38–40].
Next, we focused on the common and up-regulated genes induced by MHT. In this study it was of particular interest that the significant gene networks E and S derived from up-regulated genes in the cluster Up-I were mainly associated with the biological processes of ER stress and cell survival, respectively. Moreover, real-time qPCR demonstrated that the expression of almost all the genes (18 out of 21 genes) selected was induced by MHT in four kinds of NHF cells, indicating that these genes would be common MHT-responsive molecules in NHF cells. In network E, four genes, HSPA5 Citation[41], Citation[42], DDIT3 Citation[41], Citation[42], HERPUD1 Citation[43], and PPP1R15A Citation[42], which are very closely associated with the ER stress response, were present, and relationships between DDIT3 and HERPUD1 Citation[44], HSPA5 Citation[45] or PPP1R15A Citation[46] were identified. More recently, Xu et al. Citation[20] demonstrated that MHT (40°C) but not HT (43°C) induced a partial or full ER stress pathway in parallel with the induction of Hsp70 protein in five mammalian cell types. Pallepati and Averill-Bates Citation[21] reported that MHT (40°C) enhanced the pro-survival effects of the PERK (prot ein kinase RNA-like endoplasmic reticulum kinase)/eIF2α (alpha subunit of eukaryotic initiation factor 2) branch of ER stress. In addition, ER stress-related genes including HERPUD1 and DNAJB9 were up-regulated in normal human fibroblastic cells treated with MHT at 42°C using cDNA microarrays Citation[24]. Based on the present results and the previous reports Citation[20], Citation[21], Citation[24], we suggested that heat stresses within an MHT range can activate ER stress in our NHF cell systems. Although DDIT3 Citation[47], Citation[48] and PPP1R15A Citation[47], which are reported to be inducers of cell death during ER stress, were elevated in normal fibroblastic cells treated with MHT, no cell death was observed (). It is presumed that HSPA5 Citation[49], HERPUD1 Citation[44] and many HSPs Citation[16], which have cytoprotective activity, participate in the prevention of cell death induced by MHT. In the present study we uncovered a unique cell survival associated gene network S. In network S, most of the genes, including LIF Citation[50], HBEGF Citation[51], GADD45A Citation[52] and GADD45B Citation[52], are related to cell survival. In this network, HBEGF was reported to be positively regulated by SMAD7 Citation[53] and functionally activated by EDN1 Citation[54]. These genes in network S seemed to behave as anti-cell death molecules under the present experimental conditions.
Our previous study with the GeneChip® microarray system showed that heat stresses affect the expression of a large number of genes in human lymphoma U937 cells Citation[32], Citation[33] and human oral squamous cell carcinoma HSC-3 cells Citation[34]. Heat maps of gene expression patterns in the gene networks E and S among NHF cells in the present study and in human cancer cells Citation[32–34] are demonstrated in and of the supplementary materials. Although up-regulations of HSPA1L and DDIT3 were not detected in cancer cells, the majority of genes in network E, including many HSPs, were observed in both the HNF cells and cancer cells (). On the other hand, a remarkable difference in the gene expression patterns of genes in network S was observed among NHF cells and cancer cells (). It has also been indicated that there are differences between cancer cells and normal fibroblast cells in terms of their response to heat stress Citation[24]. These findings demonstrated that induction of HSPs is a common response to heat stresses even under different experimental conditions, including differences in heating temperature, duration of heat treatment and cell origin Citation[14], Citation[22–34]. However, at present, the specific gene expression patterns affected by heat stresses in normal cells and cancer cells are not fully understand. Further studies are required to clarify this issue.
In conclusion, common genes that were differentially expressed and/or acted within a gene network in response to MHT in NHF cells were identified. These findings provide the molecular basis for a further understanding of the mechanisms of the biological changes that are responsive to MHT in NHF cells. MHT has the advantage of being associated with fewer side effects than HT at a relatively high temperature range, and the combination of MHT with radiotherapy and/or chemotherapy is recognised as a more promising therapeutic approach than HT monotherapy in many cancers Citation[1–6]. It is thus expected that the findings obtained herein will facilitate the design of a more effective strategy for combination therapy with MHT.
Declaration of interest: This study was supported in part by a Grant-in-Aid from the Japanese Ministry of Education, Culture, Sports, Science and Technology. The authors alone are responsible for the content and writing of the paper.
References
- van der Zee J, González González D, van Rhoon GC, van Dijk JD, van Putten WL, Hart AA. Comparison of radiotherapy alone with radiotherapy plus hyperthermia in locally advanced pelvic tumours: A prospective, randomised, multicentre trial. Dutch Deep Hyperthermia Group. Lancet 2000; 355: 1119–1125
- Wust P, Hildebrandt B, Sreenivasa G, Rau B, Gellermann J, Riess H, et al. Hyperthermia in combined treatment of cancer. Lancet Oncol 2002; 3: 487–497
- Issels RD. Hyperthermia adds to chemotherapy. Eur J Cancer 2008; 44: 2546–2554
- Zagar TM, Oleson JR, Vujaskovic Z, Dewhirst MW, Craciunescu OI, Blackwell KL, et al. Hyperthermia combined with radiation therapy for superficial breast cancer and chest wall recurrence: A review of the randomised data. Int J Hyperthermia 2010; 26: 612–617
- Franckena M. Review of radiotherapy and hyperthermia in primary cervical cancer. Int J Hyperthermia 2012; 28: 543–548
- Westermann A, Mella O, Van Der Zee J, Jones EL, Van Der Steen-Banasik E, Koper P, et al. Long-term survival data of triple modality treatment of stage IIB-III-IVA cervical cancer with the combination of radiotherapy, chemotherapy and hyperthermia – An update. Int J Hyperthermia 2012; 28: 549–553
- Dewey WC. Arrhenius relationships from the molecule and cell to the clinic. Int J Hyperthermia 2009; 25: 3–20
- Yarmolenko PS, Moon EJ, Landon C, Manzoor A, Hochman DW, Viglianti BL, et al. Thresholds for thermal damage to normal tissues: An update. Int J Hyperthermia 2011; 27: 320–343
- Beachy SH, Repasky EA. Toward establishment of temperature thresholds for immunological impact of heat exposure in humans. Int J Hyperthermia 2011; 27: 344–352
- Frey B, Weiss EM, Rubner Y, Wunderlich R, Ott OJ, Sauer R, et al. Old and new facts about hyperthermia-induced modulations of the immune system. Int J Hyperthermia 2012; 28: 528–542
- Lindquist S. The heat-shock response. Annu Rev Biochem 1986; 55: 1151–1191
- Richter K, Haslbeck M, Buchner J. The heat shock response: Life on the verge of death. Mol Cell 2010; 40: 253–266
- Nollen EA, Brunsting JF, Roelofsen H, Weber LA, Kampinga HH. In vivo chaperone activity of heat shock protein 70 and thermotolerance. Mol Cell Biol 1999; 19: 2069–2079
- Beckham JT, Wilmink GJ, Opalenik SR, Mackanos MA, Abraham AA, Takahashi K, et al. Microarray analysis of cellular thermotolerance. Lasers Surg Med 2010; 42: 752–765
- Cheng L, Smith DJ, Anderson RL, Nagley P. Human neuroblastoma SH-SY5Y cells show increased resistance to hyperthermic stress after differentiation, associated with elevated levels of Hsp72. Int J Hyperthermia 2011; 27: 415–426
- Lanneau D, Brunet M, Frisan E, Solary E, Fontenay M, Garrido C. Heat shock proteins: Essential proteins for apoptosis regulation. J Cell Mol Med 2008; 12: 743–761
- Woessmann W, Meng YH, Mivechi NF. An essential role for mitogen-activated protein kinases, ERKs, in preventing heat-induced cell death. J Cell Biochem 1999; 74: 648–662
- Verheij M, Bose R, Lin XH, Yao B, Jarvis WD, Grant S, et al. Requirement for ceramide-initiated SAPK/JNK signaling in stress-induced apoptosis. Nature 1996; 380: 75–79
- Gabai VL, Yaglom JA, Volloch V, Meriin AB, Force T, Koutroumanis M, et al. Hsp72-mediated suppression of c-Jun N-terminal kinase is implicated in development of tolerance to caspase-independent cell death. Mol Cell Biol 2000; 20: 6826–6836
- Xu X, Gupta S, Hu W, McGrath BC, Cavener DR. Hyperthermia induces the ER stress pathway. PLoS One 2011; 6: e23740
- Pallepati P, Averill-Bates DA. Activation of ER stress and apoptosis by hydrogen peroxide in HeLa cells: Protective role of mild heat preconditioning at 40°C. Biochim Biophys Acta 2011; 1813: 1987–1999
- Dinh HK, Zhao B, Schuschereba ST, Merrill G, Bowman PD. Gene expression profiling of the response to thermal injury in human cells. Physiol Genomics 2001; 7: 3–13
- Sonna LA, Gaffin SL, Pratt RE, Cullivan ML, Angel KC, Lilly CM. Effect of acute heat shock on gene expression by human peripheral blood mononuclear cells. J Appl Physiol 2002; 92: 2208–2220
- Murray JI, Whitfield ML, Trinklein ND, Myers RM, Brown PO, Botstein D. Diverse and specific gene expression responses to stresses in cultured human cells. Mol Biol Cell 2004; 15: 2361–2374
- Zhou M, Zhang A, Lin B, Liu J, Xu LX. Study of heat shock response of human umbilical vein endothelial cells (HUVECs) using cDNA microarray. Int J Hyperthermia 2007; 23: 225–258
- Wong HR, Odoms K, Sakthivel B. Divergence of canonical danger signals: The genome-level expression patterns of human mononuclear cells subjected to heat shock or lipopolysaccharide. BMC Immunol 2008; 9: 24
- Schena M, Shalon D, Heller R, Chai A, Brown PO, Davis RW. Parallel human genome analysis: Microarray-based expression monitoring of 1000 genes. Proc Natl Acad Sci USA 1996; 93: 10614–10619
- Narita N, Noda I, Ohtsubo T, Fujieda S, Tok uriki M, Saito T, et al. Analysis of heat-shock related gene expression in head-and-neck cancer using cDNA arrays. Int J Radiat Oncol Biol Phys 2002; 53: 190–196
- Kato N, Kobayashi T, Honda H. Screening of stress enhancer based on analysis of gene expression profiles: Enhancement of hyperthermia-induced tumour necrosis by an MMP-3 inhibitor. Cancer Sci 2003; 94: 644–649
- Borkamo ED, Dahl O, Bruland O, Fluge O. Global gene expression analyses reveal changes in biological processes after hyperthermia in a rat glioma model. Int J Hyperthermia 2008; 24: 425–441
- Laramie JM, Chung TP, Brownstein B, Stormo GD, Cobb JP. Transcriptional profiles of human epithelial cells in response to heat: Computational evidence for novel heat shock proteins. Shock 2008; 29: 623–630
- Tabuchi Y, Takasaki I, Wada S, Zhao QL, Hori T, Nomura T, et al. Genes and genetic networks responsive to mild hyperthermia in human lymphoma U937 cells. Int J Hyperthermia 2008; 24: 613–622
- Furusawa Y, Tabuchi Y, Wada S, Takasaki I, Ohtsuka K, Kondo T. Identification of biological functions and gene networks regulated by heat stress in U937 human lymphoma cells. Int J Mol Med 2011; 28: 143–151
- Tabuchi Y, Wada S, Furusawa Y, Ohtsuka K, Kondo T. Gene networks related to the cell death elicited by hyperthermia in human oral squamous cell carcinoma HSC-3 cells. Int J Mol Med 2012; 29: 380–386
- Furusawa Y, Iizumi T, Fujiwara Y, Zhao QL, Tabuchi Y, Nomura T, et al. Inhibition of checkpoint kinase 1 abrogates G2/M checkpoint activation and promotes apoptosis under heat stress. Apoptosis 2012; 17: 102–112
- Tabuchi Y, Takasaki I, Doi T, Ishii Y, Sakai H, Kondo T. Genetic networks responsive to sodium butyrate in colonic epithelial cells. FEBS Lett 2006; 580: 3035–3041
- Ahmed K, Furusawa Y, Tabuchi Y, Emam HF, Piao JL, Hassan MA, et al. Chemical inducers of heat shock proteins derived from medicinal plants and cytoprotective genes response. Int J Hyperthermia 2012; 28: 1–8
- Westwood JT, Clos J, Wu C. Stress-induced oligomerization and chromosomal relocalization of heat-shock factor. Nature 1991; 353: 822–827
- Mariner PD, Walters RD, Espinoza CA, Drullinger LF, Wagner SD, Kugel JF, et al. Human Alu RNA is a modular transacting repressor of mRNA transcription during heat shock. Mol Cell 2008; 29: 499–509
- Spriggs KA, Bushell M, Willis AE. Translational regulation of gene expression during conditions of cell stress. Mol Cell 2010; 40: 228–237
- Wang XZ, Lawson B, Brewer JW, Zinszner H, Sanjay A, Mi LJ, et al. Signals from the stressed endoplasmic reticulum induce C/EBP-homologous protein (CHOP/GADD153). Mol Cell Biol 1996; 16: 4273–4280
- Marciniak SJ, Yun CY, Oyadomari S, Novoa I, Zhang Y, Jungreis R, et al. CHOP induces death by promoting protein synthesis and oxidation in the stressed endoplasmic reticulum. Genes Dev 2004; 18: 3066–3077
- Kokame K, Agarwala KL, Kato H, Miyata T. Herp, a new ubiquitin-like membrane protein induced by endoplasmic reticulum stress. J Biol Chem 2000; 275: 32846–32853
- Chigurupati S, Wei Z, Belal C, Vandermey M, Kyriazis GA, Arumugam TV, et al. The homocysteine-inducible endoplasmic reticulum stress protein counteracts calcium store depletion and induction of CCAAT enhancer-binding protein homologous protein in a neurotoxin model of Parkinson disease. J Biol Chem 2009; 284: 18323–18333
- Pyrko P, Schönthal AH, Hofman FM, Chen TC, Lee AS. The unfolded protein response regulator GRP78/BiP as a novel target for increasing chemosensitivity in malignant gliomas. Cancer Res 2007; 67: 9809–9816
- Birkenfeld AL, Lee HY, Majumdar S, Jurczak MJ, Camporez JP, Jornayvaz FR, et al. Influence of the hepatic eukaryotic initiation factor 2alpha (eIF2alpha) endoplasmic reticulum (ER) stress response pathway on insulin-mediated ER stress and hepatic and peripheral glucose metabolism. J Biol Chem 2011; 286: 36163–36170
- Sarkar D, Su ZZ, Lebedeva IV, Sauane M, Gopalkrishnan RV, Valerie K, et al. mda-7 (IL-24) Mediates selective apoptosis in human melanoma cells by inducing the coordinated overexpression of the GADD family of genes by means of p38 MAPK. Proc Natl Acad Sci USA 2002; 99: 10054–10059
- Kim SH, Hwang CI, Juhnn YS, Lee JH, Park WY, Song YS. GADD153 mediates celecoxib-induced apoptosis in cervical cancer cells. Carcinogenesis 2007; 28: 223–231
- Fu Y, Li J, Lee AS. GRP78/BiP inhibits endoplasmic reticulum BIK and protects human breast cancer cells against estrogen starvation-induced apoptosis. Cancer Res 2007; 67: 3734–3740
- Wysoczynski M, Miekus K, Jankowski K, Wanzeck J, Bertolone S, Janowska-Wieczorek A, et al. Leukemia inhibitory factor: A newly identified metastatic factor in rhabdomyosarcomas. Cancer Res 2007; 67: 2131–2140
- Yu WH, Woessner JF, Jr, McNeish JD, Stamenkovic I. CD44 anchors the assembly of matrilysin/MMP-7 with heparin-binding epidermal growth factor precursor and ErbB4 and regulates female reproductive organ remodeling. Genes Dev 2002; 16: 307–323
- Gupta M, Gupta SK, Hoffman B, Liebermann DA. Gadd45a and Gadd45b protect hematopoietic cells from UV-induced apoptosis via distinct signaling pathways, including p38 activation and JNK inhibition. J Biol Chem 2006; 281: 17552–17558
- Liu X, Lee J, Cooley M, Bhogte E, Hartley S, Glick A. Smad7 but not Smad6 cooperates with oncogenic ras to cause malignant conversion in a mouse model for squamous cell carcinoma. Cancer Res 2003; 63: 7760–7768
- Deacon K, Knox AJ. Endothelin-1 (ET-1) increases the expression of remodeling genes in vascular smooth muscle through linked calcium and cAMP pathways: Role of a phospholipase A(2)(cPLA(2))/cyclooxygenase-2 (COX-2)/prostacyclin receptor-dependent autocrine loop. J Biol Chem 2010; 285: 25913–25927