Abstract
Purpose: For the potential application of platinum nanoparticles (PtNPs) in hyperthermia therapy, the heating efficiency of PtNPs in the presence of radiofrequency (RF) current generated by a capacitive electric transfer (CET) system was compared with that of gold nanoparticles (AuNPs).
Materials and methods: PtNPs and AuNPs synthesised by citrate capping (5 nm) were exposed to an RF current of 0.35 ± 0.05 MHz in a CET system. The temperature of the solution containing various concentrations of platinum or gold NPs was monitored for 5 min at various power ranges.
Results: When both NP solutions were exposed to an RF field at a fixed power, the temperature of the NP solution increased continuously over the 5 min of measurement. In contrast, the NP-free solutions did not show any temperature change. Both PtNPs and AuNPs can be heated in a concentration- and power-dependent manner. However, PtNPs showed a higher efficiency in generating heat compared with AuNPs in both water and the physiological buffer.
Conclusions: The heat generating efficiency of 5-nm PtNPs was about 50% higher than that of AuNPs when they were exposed to electric current through RF. This result suggests that PtNPs are promising nanomaterials for RF-induced hyperthermia therapy.
Introduction
During the past decade, nanomedicine, the medical application of nanotechnology, has emerged as a new field in the medical sciences and has been providing new opportunities for diagnosis and therapy Citation[1]. Various nanomaterials have been developed and tested to explore possible biomedical applications Citation[1]. Among the medical treatments investigated, nanoparticle (NP)-based hyperthermia has been developed as a promising therapeutic approach for cancer treatment Citation[2–4]. In this technique, the heat released from NPs upon the exposure to electromagnetic waves damages cancer cells and renders them more sensitive to the effects of radiation, anti-cancer drugs, or a combination thereof.
Near-infrared radiation (NIR) has been widely used for stimulating NPs for NP-based cancer thermotherapy because many NPs absorb energy and release heat at this wavelength Citation[5], Citation[6]. However, NIR hyperthermia therapy has some limitations in cancer treatment because of the low penetration depth of NIR waves into tissues Citation[7]. In terms of tissue penetration, magnetic fields (MFs) have advantages in medical applications Citation[4], Citation[8], Citation[9]. Even though magnetic iron NPs have been commonly used, their heat generation in MFs is relatively inefficient. Therefore, a high concentration of magnetic NPs is required to achieve therapeutic heating in MF-induced hyperthermia treatment, which is a major flaw in the clinical application of MF Citation[10]. Radiofrequency (RF) fields have drawn attention as an alternative energy source for inducing NP-based hyperthermia, since RF fields can easily penetrate through human tissues and generate heat with high efficiency. Furthermore, it has been confirmed that RF waves are safe and have no harmful effects in human tissue Citation[11], Citation[12].
In order to improve heating efficiency of NPs for use in hyperthermia, the physical properties of NPs, such as their electrical, magnetic, optical or thermal properties, must be considered, since heat release can be induced by various means. Among the metallic NPs, there has been a great deal of research of gold-based nanomaterials for cancer therapy due to their advantages in terms of biocompatibility and their ability to conjugate with biological molecules Citation[13–17]. Therefore, RF-induced heat generation using gold NPs (AuNPs) has been widely investigated to develop a non-invasive thermotherapy for cancer Citation[18], Citation[19]. Furthermore, the recent success of the specific labelling of AuNPs to target cancer cells enables the selective thermal ablation of tumours Citation[20]. To accelerate the clinical application of the NP-based RF thermotherapy, it is necessary to improve the selectivity of its cytotoxic effect on cancer cells by reducing the thermal damage to normal cells and by increasing the heat generating efficiency of NPs bound to cancer cells, which eventually reduces the concentration of NPs needed for hyperthermia cancer therapy. Therefore, the heat responses of various NPs subjected to an RF field should be investigated.
In this study we measured the heat generating efficiency of platinum nanoparticles (PtNPs) in the presence of an RF current generated via a capacitive electric transfer (CET) system for the first time, and compared the results with those of AuNPs. CET has been developed for non-invasive thermotherapy to treat pain symptoms Citation[21], Citation[22]. This method is based on the application of electric currents in the frequency range of 0.3 to 0.6 MHz, which has been proven not to be harmful to human tissue Citation[23], Citation[24]. PtNPs have been extensively investigated for their applications in various fields such as fine chemical synthesis Citation[25], hydrogen production Citation[26], and biomedicine Citation[27–29]. It was demonstrated that the toxicity of PtNPs is as low as AuNPs at concentrations up to 25 ppm when zebrafish embryos were used as a model organism Citation[30]. Moreover, the toxicity of PtNPs to the cultured cells was dramatically reduced when they were encapsulated by protein shells Citation[31]. In addition, PtNPs show antioxidant activity Citation[27–29] and can be functionalised by surface modification Citation[32] or encapsulation using protein shells Citation[31], Citation[33]. Taken together, PtNPs possess superior properties as a candidate material for biomedical application with low cell toxicity.
Here we compared the heat generating efficiency of 5-nm PtNPs with AuNPs of the same size using CET as an RF source. The 5-nm AuNPs were chosen since they have been widely studied for application to RF-induced thermotherapy Citation[34–37].
Experimental section
Materials
All chemicals were purchased from Sigma-Aldrich (Saint Louis, MO, USA) and used as received without further purification. Citrate-capped AuNP solution was purchased from Ted Pella (Redding, CA, USA). Dulbecco's phosphate buffered saline (DPBS) was purchased from Life Technologies (Carlsbad, CA); 1 × DPBS contains 2.68 mM KCl, 1.47 mM KH2PO4, 136.99 mM NaCl, and 8.10 mM Na2HPO4, with a pH of 7.4.
Sample preparation
With some modifications, 5-nm PtNP was synthesised according to the reported procedures Citation[38]. Briefly, PtNP was obtained by reducing 1 mM chloroplatinic acid (H2PtCl6) with 10 mM sodium borohydride (NaBH4) in the presence of 1 mM trisodium citrate (Na3C6H5O7) in a 50-mL reaction mixture. The amounts of each component listed above are their final concentrations in the reaction mixture. The NaBH4 solution was added dropwise to the reaction mixture with constant stirring for 1 h. The final product was kept at 4°C for further use.
Material characterisation
Transmission electron microscopy (TEM) images were taken using JEOL JEM-3010 microscopes (Tokyo, Japan) operated at 300 kV. TEM samples were prepared by applying the citrate-PtNP or citrate-AuNP solution to a copper grid covered with a thin carbon film (JEOL) for 30 s, and dehydrating it overnight at 25°C. The sizes of the NPs were estimated by averaging 200 individual particles using the Gatan Digital Micrograph software (Pleasanton, CA).
The concentrations of PtNPs and AuNPs were determined by inductively coupled plasma mass spectroscopy with an Agilent 7500 system (Santa Clara, CA).
Heating of NP solutions using an RF current
A CET system was composed of an RF generator and an incubation chamber. The RF generator was modified from an E-motion Plus TM303 system (Plus, Seoul, Korea) with a frequency range of 0.05–0.50 MHz and a power range of 10–50 W. This RF generator was basically similar to Indiba electronic devices that are commonly used in medical treatments Citation[21], Citation[22]. The frequency and output power of the RF generator were measured by a Tektronix oscilloscope TDS 210 (Beaverton, OR, USA). Two electrodes connected to the RF generator block each end of the incubation chamber, a glass cylinder 8 cm in length and 2 cm in diameter. NP solution or control buffer are exposed to RF in the incubation chamber (). Active and return electrodes were made of insulated metal plates with a diameter of 2 cm (). To prevent thermal loss during the experiment, thick-walled glass with a thickness of 0.5 cm and low thermal conductive polymer were used for the reaction chamber and the electrode cover, respectively. In addition, the entire reaction chamber was put in an acyl box to minimise the temperature fluctuation. An RF of 0.35 ± 0.05 MHz with a power of 40 W was applied for most measurements unless otherwise specified. The temperature of the reaction solution containing various concentrations of platinum or gold NPs in the reaction chamber was monitored for 5 min using a fibre-optic temperature sensor system with an accuracy of ±0.1°C, The fibre-optic temperature sensor was used to avoid artefact which may arise from the interference of RF. The temperature sensor was vertically placed in the centre of the reaction chamber in all the measurements ().
Figure 1. The schematic of the CET system. An RF current was applied from the active electrode (red) to the return electrode (black) through the incubation chamber. Each electrode was coated with an insulated material. An oscilloscope and a temperature sensor measured the electric parameters applied to the active electrode and the temperatures in the incubation chamber, respectively. Various concentrations of NPs (spherical black dots) in deionised water or buffer can be placed in the incubation chamber.
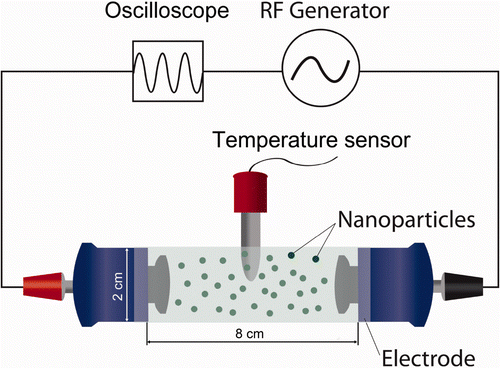
Results and discussion
PtNP was synthesised using trisodium citrate as a stabiliser and reducing agent. The TEM images showed the monodispersed spherical PtNPs and AuNPs with sizes of approximately 5 nm: 5.1 ± 0.6 nm for the PtNPs and 5.5 ± 0.6 nm for the AuNPs (). When either PtNPs or AuNPs in the deionised water were exposed to the RF field at a constant power of 40 W, the temperature was continuously increased over 5 min of measurement (). In contrast, under the same reaction conditions, a temperature increment was not observed when the NP-free solution was used (). Over this period, the heating rate was proportional to the concentration of NPs and increased almost linearly for concentrations higher than 1 ppm (). Intriguingly, PtNPs showed a higher efficiency in heat generation than AuNPs in all concentration ranges, and the heating rate of the PtNPs was about 50% higher than that of AuNPs. For example, the temperatures of the incubation chamber were raised by 24.0 and 15.5 degrees in the presence of 10 ppm PtNPs and AuNPs, respectively, over 5 min of exposure to the RF current (). At this concentration, the RF heating rate was 4.8°C/min for the PtNPs, but only 3.1°C/min for the AuNPs ().
Figure 2. TEM images of PtNPs (A) and AuNPs (B). The size distribution diagrams are shown in the bottom panels. The scale bar in the TEM picture is 50 nm.
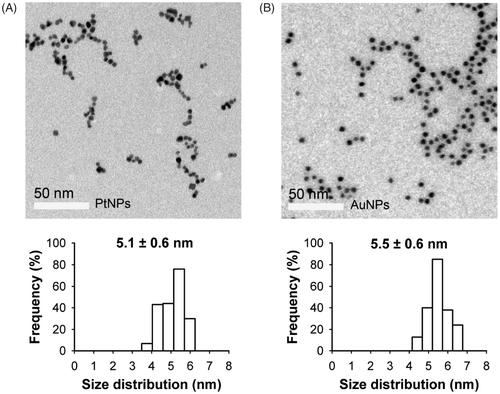
Figure 3. Heating rate of the PtNPs (A) and AuNPs (B) at various concentrations. The temperatures of the PtNPs and AuNPs in the deionised water were monitored every 5 s for 5 min. The largest temperature increment was observed in the PtNP solution.
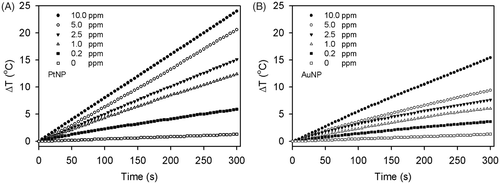
Figure 4. Concentration dependence of the RF heating rates of the PtNPs (black circles) and AuNPs (white circles). The heating rates of NPs at each concentration were the temperature changes over 5 min from . The solid lines were obtained using least squares regression analysis. The heating rate of the PtNPs was about two-fold higher than that of the AuNPs.
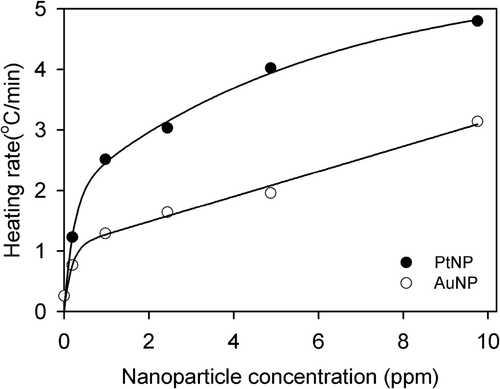
It is crucial that the smallest amount of NPs be used for therapeutic hyperthermia since the safety of NPs has not been completely evaluated Citation[39], Citation[40]. In this respect our current results are very encouraging and support that both PtNPs and AuNPs can release heat and increase temperature with exposure to the RF current generated from a CET system even at a very low concentration of NPs; 0.2 ppm of PtNPs and AuNPs increased temperatures by 6.0° and 3.5°C, respectively, after just 5 min of exposure at 40 W ().
To determine the effect of the RF field power on heating, solutions containing PtNPs and AuNPs with concentrations of 2.5 ppm were exposed to the RF field at power ranges from 20 to 50 W for 5 min. We confirmed that temperature increased in a power-dependent manner in the solutions containing PtNPs or AuNPs (). Consistently, when the same power was applied, the PtNPs also showed a higher temperature change than the AuNPs.
Figure 5. Heating rates of the PtNPs (A) and AuNPs (B) at the power ranges of 20 to 50 W. Under the exposure of the NP solution to the RF current at a given power, the temperatures of the PtNPs and AuNPs at the concentration of 2.5 ppm in the deionised water were monitored every 5 s for 5 min.
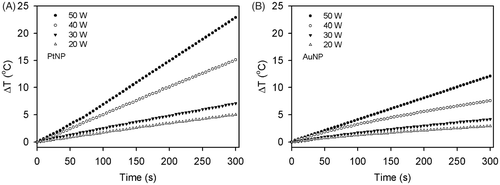
We further confirmed the temperature changes of PtNPs and AuNPs dissolved in DPBS solution, a physiological buffer used in animal cell cultures. The results indicated that PtNPs and AuNPs at a concentration of 2.5 ppm dissolved in 1 × DPBS showed approximately the same temperature changes as those dissolved in deionised water ( and ). Interestingly, 1 × DPBS alone exhibited a slight temperature change (), while deionised water did not show any temperature change under the same reaction conditions and time interval (). This result was expected, since the high frequency current is considered to raise the solution temperature in part by the Joule effect on the electrolytes Citation[41]. To see the sole contribution of NPs to heat release, the degree of temperature rise in NP-free DPBS was subtracted from that in NP-containing DPBS (inset in ). This revealed that PtNPs consistently showed a higher heating efficiency than AuNPs even in a physiological buffer.
Figure 6. Heating rates of PtNPs (black circles) and AuNPs (white circles) in DPBS solution. PtNPs and AuNPs prepared in 1 × DPBS solution at a concentration of 2.5 ppm were exposed to a 40 W RF current and their temperatures were monitored for 5 min. The temperature changes of the DPBS solution were also measured under the same conditions as a control (black triangles). The temperature changes induced by PtNP and AuNP, calculated by subtracting the temperatures of the control from those of the NP solutions, are also displayed in the inset.
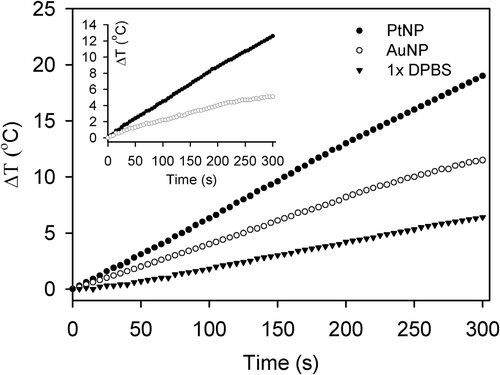
The use of RF wave-irradiated NPs for non-invasive thermal therapies has been proposed for cancer treatment. Accordingly, electromagnetic absorption in metallic NPs and the following heat production have been intensively studied in theoretical and experimental aspects Citation[20], Citation[34], Citation[42–44]. However, the mechanism of how NPs generate heat against RF field is still a controversial topic Citation[20], Citation[41–45]. Initially, the Joule-heating model was proposed to explain the heating mechanism of gold NPs under RF fields Citation[34]. However, recent studies found that the heating effect is not simply interpreted by the resistivity in the Joule-heating model, but influenced by many variables such as conductivity, dielectric property, surface plasmon, size and shape of NPs, impurities of NPs, frequency of wave, ionic strength in the solution and inter-particle interactions Citation[34], Citation[42–44], Citation[46]. Considering these disputes, the difference in the RF-induced heating rates between PtNPs and AuNPs would not be explained without more elaborate experiments. The main purpose of the current study was to evaluate the potential of platinum particles as a candidate material for RF-based non-invasive thermal therapies, and we demonstrated that PtNPs have a higher heating rate than AuNPs in a CET system. The efficiency of heat generation from PtNPs under an RF field will need to be optimised for therapeutic applications, and mechanistic understanding requires further experimental and theoretical investigations.
Conclusions
In this study we explored the heat-generating property of PtNPs when exposed to a CET-generated RF current with a frequency of 0.35 MHz. The temperature of the NP solution in the incubation chamber was raised by the heat released from NPs. We found that the heat released from the PtNPs or the temperature increment in the incubation chamber was proportional to the exposure time, the concentration of PtNPs, and the power of the RF field. Most importantly, we proved that PtNPs showed superior heating efficiency to AuNPs. Considering that their toxicities are comparable, PtNPs could be developed for the application to RF-induced hyperthermia therapy and widen the range of choices in developing NPs for this purpose. Moreover, the feasibility of the surface modification of PtNPs Citation[32] makes them biocompatible and multifunctional. Therefore, the prospects of PtNP-based multifunctional NPs are promising for future biomedical applications.
Acknowledgements
We thank Seung Woo Kim and Mun Seong Heo for helping the modification of the RF machine, and Eun Ae Kim for preparing the illustration.
Declaration of interest: This work was supported by the Next-Generation BioGreen 21 Program (SSAC PJ008107), Korea Healthcare Technology R&D Project (A092006), National Research Foundation of Korea grant number 2011-0028878, and Samsung Biomedical Research Institute. B.H.S., S.H.M. and K.K.K. designed the research, analysed the data and wrote the paper; B.H.S. and S.H.M. performed the research. The authors alone are responsible for the content and writing of the paper.
References
- Barreto JA, O’Malley W, Kubeil M, Graham B, Stephan H, Spiccia L. Nanomaterials: Applications in cancer imaging and therapy. Adv Mater 2011; 23: H18–40
- Day ES, Morton JG, West JL. Nanoparticles for thermal cancer therapy. J Biomech Eng 2009; 131: 074001
- Krishnan S, Diagaradjane P, Cho SH. Nanoparticle-mediated thermal therapy: Evolving strategies for prostate cancer therapy. Int J Hyperthermia 2010; 26: 775–789
- Johannsen M, Thiesen B, Wust P, Jordan A. Magnetic nanoparticle hyperthermia for prostate cancer. Int J Hyperthermia 2010; 26: 790–795
- Kam NWS, O’Connell M, Wisdom JA, Dai HJ. Carbon nanotubes as multifunctional biological transporters and near-infrared agents for selective cancer cell destruction. P Natl Acad Sci USA 2005; 102: 11600–11605
- El-Sayed IH, Huang XH, El-Sayed MA. Selective laser photo-thermal therapy of epithelial carcinoma using anti-EGFR antibody conjugated gold nanoparticles. Cancer Lett 2006; 239: 129–135
- Huang X, El-Sayed IH, Qian W, El-Sayed MA. Cancer cell imaging and photothermal therapy in the near-infrared region by using gold nanorods. J Am Chem Soc 2006; 128: 2115–2120
- Jordan A, Wust P, Fahling H, John W, Hinz A, Felix R. Inductive heating of ferrimagnetic particles and magnetic fluids: Physical evaluation of their potential for hyperthermia. Int J Hyperthermia 1993; 9: 51–68
- Johannsen M, Gneveckow U, Eckelt L, Feussner A, Waldofner N, Scholz R, et al. Clinical hyperthermia of prostate cancer using magnetic nanoparticles: Presentation of a new interstitial technique. Int J Hyperthermia 2005; 21: 637–647
- Pankhurst QA, Connolly J, Jones SK, Dobson J. Applications of magnetic nanoparticles in biomedicine. J Phys D Appl Phys 2003; 36: R167–R181
- Erdreich LS, Klauenberg BJ. Radio frequency radiation exposure standards: Considerations for harmonization. Health Phys 2001; 80: 430–439
- Adair ER, Kelleher SA, Mack GW, Morocco TS. Thermophysiological responses of human volunteers during controlled whole-body radio frequency exposure at 450 MHz. Bioelectromagnetics 1998; 19: 232–245
- Jelveh S, Chithrani DB. Gold nanostructures as a platform for combinational therapy in future cancer therapeutics. Cancers 2011; 3: 1081–1110
- Connor EE, Mwamuka J, Gole A, Murphy CJ, Wyatt MD. Gold nanoparticles are taken up by human cells but do not cause acute cytotoxicity. Small 2005; 1: 325–327
- Raoof M, Zhu C, Kaluarachchi WD, Curley SA. Luciferase-based protein denaturation assay for quantification of radiofrequency field-induced targeted hyperthermia: Developing an intracellular thermometer. Int J Hyperthermia 2012; 28: 202–209
- Stafford RJ, Shetty A, Elliott AM, Schwartz JA, Goodrich GP, Hazle JD. MR temperature imaging of nanoshell mediated laser ablation. Int J Hyperthermia 2011; 27: 782–790
- Elliott AM, Shetty AM, Wang J, Hazle JD, Jason Stafford R. Use of gold nanoshells to constrain and enhance laser thermal therapy of metastatic liver tumours. Int J Hyperthermia 2010; 26: 434–440
- Cardinal J, Klune JR, Chory E, Jeyabalan G, Kanzius JS, Nalesnik M, et al. Noninvasive radiofrequency ablation of cancer targeted by gold nanoparticles. Surgery 2008; 144: 125–132
- Glazer ES, Zhu C, Massey KL, Thompson CS, Kaluarachchi WD, Hamir AN, et al. Noninvasive radiofrequency field destruction of pancreatic adenocarcinoma xenografts treated with targeted gold nanoparticles. Clin Cancer Res 2010; 16: 5712–5721
- Raoof M, Corr SJ, Kaluarachchi WD, Massey KL, Briggs K, Zhu C, et al. Stability of antibody-conjugated gold nanoparticles in the endolysosomal nanoenvironment: Implications for noninvasive radiofrequency-based cancer therapy. Nanomedicine 2012; 8: 1096–1105
- Takahashi K, Suyama T, Onodera M, Hirabayashi S, Tsuzuki N, Li Z-S. Clinical effects of capacitive electric transfer hyperthermia therapy for lumbago. J Phys Ther Sci 1999; 11: 7
- Takahashi K, Suyama T, Takakura Y, Hirabayashi S, Tsuzuki N. Clinical effect of capacitive electric transfer hyperthermia therapy for cervico-omo-branchial pain. J Phys Ther Sci 2000; 12: 6
- Hernandez-Bule ML, Trillo MA, Cid MA, Leal J, Ubeda A. In vitro exposure to 0.57-MHz electric currents exerts cytostatic effects in HepG2 human hepatocarcinoma cells. Int J Oncol 2007; 30: 583–592
- Hernandez-Bule ML, Cid MA, Trillo MA, Leal J, Ubeda A. Cytostatic response of HepG2 to 0.57 MHz electric currents mediated by changes in cell cycle control proteins. Int J Oncol 2010; 37: 1399–1405
- Stamenkovic VR, Fowler B, Mun BS, Wang G, Ross PN, Lucas CA, et al. Improved oxygen reduction activity on Pt3Ni(111) via Increased Surface Site availability. Science 2007; 315: 493–497
- Varpness Z, Peters JW, Young M, Douglas T. Biomimetic synthesis of a H2 catalyst using a protein cage architecture. Nano Lett 2005; 5: 2306–2309
- Kajita M, Hikosaka K, Iitsuka M, Kanayama A, Toshima N, Miyamoto Y. Platinum nanoparticle is a useful scavenger of superoxide anion and hydrogen peroxide. Free Radical Res 2007; 41: 615–626
- Hikosakaa K, Kima J, Kajitaa M, Kanayamaa A, Miyamoto Y. Platinum nanoparticles have an activity similar to mitochondrial NADH:ubiquinone oxidoreductase. Colloids Surf B 2008; 66: 195–200
- Kim J, Takahashi M, Shimizu T, Shirasawa T, Kajita M, Kanayama A, et al. Effects of a potent antioxidant, platinum nanoparticle, on the lifespan of Caenorhabditis elegans. Mech Ageing Dev 2008; 129: 322–331
- Asharani PV, Lianwu Y, Gong Z, Valiyaveettil S. Comparison of the toxicity of silver, gold and platinum nanoparticles in developing zebrafish embryos. Nanotoxicology 2011; 5: 43–54
- San BH, Moh SH, Kim KK. The effect of protein shells on the antioxidant activity of protein-encapsulated platinum nanoparticles. J Mater Chem 2012; 22: 1774–1780
- Yee C, Scotti M, Ulman A, White H, Rafailovich M, Sokolov J. One-phase synthesis of thiol-functionalized platinum nanoparticles. Langmuir 1999; 15: 4314–4316
- San BH, Kim S, Moh SH, Lee H, Jung DY, Kim KK. Platinum nanoparticles encapsulated by aminopeptidase: A multifunctional bioinorganic nanohybrid catalyst. Angew Chem Int Ed 2011; 50: 11924–11929
- Moran CH, Wainerdi SM, Cherukuri TK, Kittrell C, Wiley BJ, Nicholas NW, et al. Size-dependent Joule heating of gold nanoparticles using capacitively coupled radiofrequency fields. Nano Res 2009; 2: 400–405
- Gannon CJ, Patra CR, Bhattacharya R, Mukherjee P, Curley SA. Intracellular gold nanoparticles enhance non-invasive radiofrequency thermal destruction of human gastrointestinal cancer cells. J Nanobiotechnology 2008; 6: 2
- Raoof M, Curley SA. Non-invasive radiofrequency-induced targeted hyperthermia for the treatment of hepatocellular carcinoma. Int J Hepatol 2011; 2011: 676957
- Kruse DE, Stephens DN, Lindfors HA, Ingham ES, Paoli EE, Ferrara KW. A radio-frequency coupling network for heating of citrate-coated gold nanoparticles for cancer therapy: Design and analysis. IEEE Trans Biomed Eng 2011; 58: 2002–2012
- Xiao XY, Bard AJ. Observing single nanoparticle collisions at an ultramicroelectrode by electrocatalytic amplification. J Am Chem Soc 2007; 129: 9610–9612
- Buzea C, Pacheco II, Robbie K. Nanomaterials and nanoparticles: Sources and toxicity. Biointerphases 2007; 2: Mr17–71
- Schrand AM, Rahman MF, Hussain SM, Schlager JJ, Smith DA, Syed AF. Metal-based nanoparticles and their toxicity assessment. Wiley Interdiscip Rev Nanomed Nanobiotechnol 2010; 2: 544–568
- Li D, Jung YS, Tan S, Kim HK, Chory E, Geller DA. Negligible absorption of radiofrequency radiation by colloidal gold nanoparticles. J Colloid Interface Sci 2011; 358: 47–53
- Liu X, Chen HJ, Chen X, Parini C, Wen D. Low frequency heating of gold nanoparticle dispersions for non-invasive thermal therapies. Nanoscale 2012; 4: 3945–3953
- Hanson GW, Patch SK. Optimum electromagnetic heating of nanoparticle thermal contrast agents at RF frequencies. J Appl Phys 2009; 106: 054309(1)–054309(10)
- Hanson GW, Monreal RC, Apell SP. Electromagnetic absorption mechanisms in metal nanospheres: Bulk and surface effects in radiofrequency-terahertz heating of nanoparticles. J Appl Phys 2011; 109: 124306(1)–124306(6)
- Pearce JA, Cook JR. Heating mechanisms in gold nanoparticles at radio frequencies. Conf Proc IEEE Eng Med Biol Soc 2011; 2011: 5577–5580
- Hanson G, Kim H, Geller D, Patch S, Shuba M, Maksimenko S. The effect of sample holder geometry on electromagnetic heating of nanoparticle and NaCl solutions at 13.56 MHz. IEEE Trans Biomed Eng 2012; 59: 3468–3474