Abstract
Purpose: A synergistic cancer cell killing effect of sub-lethal hyperthermia and chemotherapy has been reported extensively. In this study, in vitro cell culture experiments with a uterine cancer cell line (MES-SA) and its multidrug resistant (MDR) variant MES-SA/Dx5 were conducted in order to investigate the role of heating rate in achieving a synergistic effect. The mode of cell death, induction of thermal tolerance and P-glycoprotein (P-gp) mediated MDR following two different rates of heating were studied.
Materials and methods: Doxorubicin (DOX) was used as the chemotherapy drug. A rapid rate hyperthermia was achieved by near infrared laser (NIR) excited indocyanine green (ICG) dye (absorption maximum at 808 nm, ideal for tissue penetration). A slow rate hyperthermia was provided by a cell culture incubator.
Results: The potentiating effect of hyperthermia to chemotherapy can be maximised by increasing the rate of heating. When delivered at the same thermal dose, a rapid increase in temperature from 37°C to 43°C caused more cell membrane damage than gradually heating the cells from 37°C to 43°C and thus allowed for more intracellular accumulation of DOX. Meanwhile, the rapid rate laser-ICG hyperthermia at 43°C caused cell necrosis whereas the slow rate incubator hyperthermia at 43°C induced mild apoptosis. At 43°C a positive correlation between thermal tolerance and the length of hyperthermia exposure is identified.
Conclusions: This study shows that by increasing the rate of heating, less thermal dose is needed in order to overcome P-gp mediated MDR.
Introduction
Hyperthermia in combination with chemotherapy has been widely used in cancer treatment. The most obvious benefit of hyperthermia to chemotherapy is the fact that hyperthermia shows synergy with some drugs against cancer cells Citation[1–4]. This may make it helpful in treating tumours that are resistant or refractory to chemotherapeutic agents. Cancer cells can become resistant to chemotherapeutic agents over time. Research has shown that the exposure to anticancer drugs such as doxorubicin (DOX) can initially kill the majority of the cancer cells, but may cause the remainder to become insensitive not only to DOX but also to a variety of other chemotherapeutic agents Citation[5]. This effect is called multidrug resistance (MDR). A plasma membrane P-glycoprotein (P-gp) is consistently found to be over-expressed in a variety of MDR cell lines Citation[6]. P-gp can export functionally and structurally unrelated hydrophobic molecules out of the cell membrane using the energy of ATP hydrolysis Citation[7]. As a result, a decreased net cellular accumulation of the chemotherapy agent is observed in MDR cells.
Various pre-clinical as well as clinical reports have suggested the role of hyperthermia in overcoming P-gp regulated MDR Citation[2], Citation[7–12]. The exact mechanism of how hyperthermia can overcome MDR is still unclear. The cause may be multifactorial: transmembrane conductivity, sodium/potassium-ATPase activity, glutathione metabolism and P-gp activity could all be affected by hyperthermia Citation[13]. At any rate, it is widely accepted that the fluidity and permeability of the cell membrane is modified upon heat exposure Citation[14]. If DOX is applied together with hyperthermia, an increase in net DOX accumulation is expected due to the increased membrane permeability compared to normal temperature (37°C), which is the basis of hyperthermia synergy to chemotherapy.
In our previous study we observed different hyperthermia chemotherapy interaction in a non-MDR ovarian cancer cell line following different rates of heating Citation[15]. A rapid rate hyperthermia shows synergy to chemotherapy whereas a sub-additive effect was found when a slow rate hyperthermia was applied. The rapid rate heating was achieved by a near infrared (NIR) laser exciting indocyanine green (ICG) dye that had been pre-loaded into the cells. This system is capable of raising the temperature from the baseline 37°C to 43°C within 1 min. As a comparison, the slow rate heating was produced by a cell culture incubator. In contrast, it took about 50 min for a single well of a 96-well plate containing 200 µL media to reach 43°C in the incubator. Since the heating rate was slow and the duration of hyperthermia treatment was prolonged in the incubator hyperthermia, one could easily speculate that thermotolerance may have occurred and diminished the effect of hyperthermia. Cancer cells may confer thermotolerance as a result of continuous heat exposure within as little as 30 min at 43°C Citation[14]. The development of thermotolerance is mainly due to heat shock protein (HSP) over-expression to repair misfolded proteins during heat stress Citation[16], although some reports indicated an uncoupling of thermotolerance and Hsp70 expression under slow rate heating conditions Citation[17]. It has also been reported that the synergy between chemotherapy (DOX) and hyperthermia is decreased if the heating is applied before the DOX treatment Citation[18], Citation[19] which may be a direct result of cancer cells obtaining resistance through prior heat exposure. Although delivering hyperthermia and chemotherapy simultaneously may not allow for the most appropriate timing, drug release from the carrier can be controlled, so it may be possible to induce hyperthermia after chemotherapy. There are already a few such ‘smart’ nanoparticle designs Citation[20–22]. Another possible reason for the sub-additive effect found in incubator hyperthermia is the induction of MDR activity leading to DOX tolerance. It is not clear whether there is a causation relationship between MDR and thermotolerance, however, the simultaneous induction of MDR and thermotolerance under hyperthermia has been observed by several groups Citation[16], Citation[23–26].
It has been reported that the rate of heating is a critical factor in determining the synergy of hyperthermia and chemotherapy Citation[18]. The purpose of this study is to investigate the reason for the different response to the rapid/slow rate heating and thus provide a rationale for hyperthermia delivered by intracellularly localised excitable compounds. We try to explore the molecular basis of these phenomena that we had observed. As a general concept, the effect of hyperthermia is thermally dose dependent. In most thermal dose calculation models, such as the widely used CEM43°C (CEM43) model where thermal dose is normalised to cumulative equivalent minutes at 43°C Citation[27], pure thermal damage could be described by two parameters, temperature and duration of heating. In the case of combined therapy involving hyperthermia, these two parameters might not be enough to describe the combined effect. We hypothesise that the rate of temperature increase in a hyperthermia treatment can affect the internalisation of chemotherapeutic agents. More severe cell membrane damage may have occurred after the rapid rate heating, allowing more DOX to be accumulated in the cell compared to that which occurred during slow rate hearing. We also expect to see more necrotic cell death after rapid rate heating since we hypothesise more severe cell membrane damage, whereas apoptotic cell death is more likely after slow rate hyperthermia. In order to test this hypothesis we have included a MDR positive cell line in this study. Since MDR transporter protein P-gp is embedded in the cell membrane, damage to the cell membrane will lead to P-gp malfunction. If DOX is applied together with this type of rapid rate heating, DOX accumulation in the cell nucleus is expected.
Materials and methods
Cell culture and chemical preparation
A MDR uterine sarcoma cell line MES-SA/Dx5 (Dx5) and its parental drug-sensitive cell line MES-SA obtained from the America Type Culture Collection (ATCC, Manassas, VA) were cultured with McCoy's 5A media (ATCC) supplemented with 10% foetal bovine serum (ATCC) and 1% penicillin-streptomycin (GIBCO, Carlsbad, CA) and maintained in a Heracell (Thermo Scientific, Waltham, MA) cell culture incubator containing 5% carbon dioxide at 37°C. Cells were harvested using 0.25% trypsin-EDTA (GIBCO) and subcultivated at a 1:10 ratio upon confluence. DOX (Sigma-Aldrich, Saint Louis, MI) solution was prepared by dissolving 10 mg powder in 1 mL of DMSO (Sigma-Aldrich). Dulbecco's phosphate buffered saline (DPBS) (GIBCO) was used to further dilute DOX to desired concentrations. According to the manufacturer's instruction, ICG (Sigma-Aldrich) in its solution form is stable only for 8 h. Therefore, fresh ICG solution was prepared before each experiment by dissolving 1 mg of ICG powder in 1 mL of de-ionised water. Further dilutions were prepared using DPBS to desired concentrations.
Thermal dose calculation
We use the Arrhenius model Citation[27] to calculate thermal dose. Details of the calculation can be seen in the supplementary material.
Hyperthermia treatment
The heating system used here, including both the incubator and laser-ICG hyperthermia delivery system, is the same as has been described previously Citation[15]. The temperature calibration study, as well as hyperthermia treatment by both heating systems, followed the same protocol as we have established in our previous paper.
Thermotolerance detection
The 70 kDa heat shock proteins (Hsp70s) were chosen to study the induction of thermotolerance after hyperthermia exposure because they provide thermotolerance to cells on exposure to heat stress. Enzyme-linked immunosorbent assay (ELISA) was used to detect the expression of Hsp70 using its corresponding antigen.
Mode of cell death
Two different assays were used to detect apoptotic and necrotic cell death after either chemotherapy/hyperthermia treatment alone or in combination. Cellular caspase-3 level was monitored as an indicator of apoptosis because the activation of caspase-3 is a unique and universal event for the initiation of all intracellular events in cell apoptosis. A caspase-3 activity assay kit (Roche Applied Science, Indianapolis, IN) was used for this purpose. This is a fluorometric immunosorbent enzyme assay (FIENA) for the quantitative determination of Caspase-3 activity in 96-well plate pre-coated with anti-caspase-3 agent. Caspase-3 levels were quantified 1, 3, 6, 12 and 24 h after the hyperthermia treatment and then normalised to their respective control which is the caspase-3 value from cells that did not receive any treatment.
Apoptotic/necrotic cell death was distinguished by a method using fluorescence microscopy with Hoechst 33342 and propidium iodide (PI) (Invitrogen, Carlsbad) when DOX is not used or used at low concentration. The simultaneous use of ICG and Hoechst 33342/PI without cross-interference is possible based reports by several groups Citation[28], Citation[29]. In practice, we have not seen any overlap between their absorbance and emission spectra. After hyperthermia treatment, cells were stained with 10 µg/mL of Hoechst 33342 and 10 µg/mL of PI for 5 min and analysed under an Olympus IX81 fluorescent microscope at 20× magnification (Olympus America, Center Valley, PA). Hoechst 33342 stained all nuclei and PI stained the nuclei of cells with a disrupted plasma membrane. Nuclei of viable, necrotic and apoptotic cells were observed as round blue nuclei, round pink nuclei and fragmented blue or pink nuclei, respectively.
P-gp activity
Calcein-AM test was used to monitor the function of the P-gp drug efflux pump during and after hyperthermia treatment. Similar to DOX, calcein-AM is a lipophilic non-fluorescent dye that can penetrate the cell membrane by free diffusion. After entering the cell, the ester bonds of calcein-AM are cleaved. This transforms lipophilic non-fluorescent calcein-AM into hydrophilic fluorescent calcein, which can no longer diffuse out of the cell. P-gp can actively transport calcein-AM outside the cell membrane. Therefore, P-gp activity can be quantified by calcein fluorescence intensity: non-MDR cells will show strong fluorescence while MDR cells will show little fluorescence. In this study, the calcein-AM assay was performed immediately after incubator hyperthermia or hyperthermia induced by NIR dye (ICG) radiation. The calcein-AM assay was also performed in non-heated drug-sensitive cells to serve as a positive control and in non-heated MDR cells to serve as a negative control.
Results
Thermal dose calculation
The temperature increase rate in the incubator hyperthermia is much slower than the temperature increase observed during ICG/laser exposure. As described in our pervious paper, 43°C incubator hyperthermia treatment for 1 h produced similar cytotoxicity as laser-ICG hyperthermia for 3 min with 5 µM ICG. The calculated CEM43 was 23.5 and 6.2 min in the two types of heat treatment evaluated (slow rate 1 h incubator and fast rate 3 min ICG/NIR laser exposure respectively). The fact that the thermal dose produced in the incubator hyperthermia is almost 4 times the laser-ICG hyperthermia, and that the cytotoxicity is similar to the laser-ICG hyperthermia confirmed the importance of the rate of heating. In order to further study the effect of the rate of heating on cell killing and/or growth inhibition, two other types of heating regime at their isoeffect dose, namely 10 µM ICG + 3 min laser and 50°C incubator for 30 min were also included in this study. The temperature profile during heating and the calculated thermal dose can be found in the supplementary material.
Cell death caused by DOX chemotherapy
MES-SA and Dx5 cells were treated with 1 µM or 5 µM DOX for up to 24 h. Results from the caspase-3 activity assay () suggested that there is apoptotic cell death after DOX treatment in both MES-SA and Dx5 cells. No difference in caspase-3 expression was observed between MES-SA and Dx5 cells under controlled conditions (no DOX); therefore, each treatment value was presented as the percentage increase/decrease compared to control values. An ANOVA test at each time point among the treatment groups indicated that 5 µM DOX-treated MES-SA cells had higher caspase-3 expression than the other three groups at 12-, 18- and 24-h time points. Meanwhile, in both cell lines, 1 µM DOX treatment had consistently lower caspase-3 level compared to 5 µM DOX treatment at 12-, 18- and 24-h time points.
Figure 1. Caspase-3 level after DOX treatment. n = 3 experiments. Data were presented as mean ± SD. *Statistical significance comparing each treatment to control cells (cells without treatment), with one-sided Student's t-test (α = 0.05). ★Statistical significance among the groups by ANOVA with Bonferroni post-hoc correction (α = 0.05).
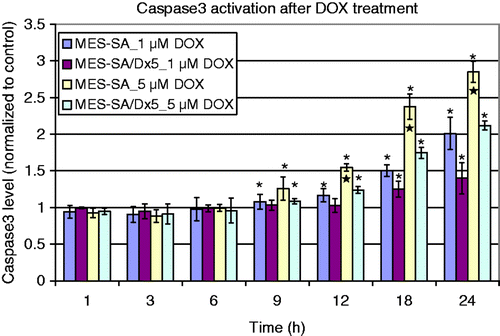
The result indicated that the induction of apoptosis is DOX concentration dependent; higher concentration of DOX caused more caspase-3 production. 1 µM of DOX did not change caspase-3 expression in Dx5 cells. Results from the DOX uptake assay (shown below) indicated that the low caspase-3 activation is due to MDR decreasing DOX uptake in Dx5 cells. Furthermore, the activation of caspase-3 depends on the DOX incubation time: longer incubation induced higher caspase-3 expression for up to 24 h.
Cell death caused by hyperthermia treatment
Incubator hyperthermia
As shown in , MES-SA and Dx5 cells were stained with Hoechst 33342 and PI either immediately after the hyperthermia treatment or 24 h post-treatment in order to distinguish normal, apoptotic and necrotic cells. Different modes of cell death were observed at the two thermal doses. Mild apoptosis was observed 24 h after the low thermal dose 1 h 43°C incubator hyperthermia; whereas the high thermal dose treatment, incubating cells for 30 min at 50°C caused necrosis. As shown in , control MES-SA and Dx5 cells showed no PI staining, indicating the integrity of cell membranes. After 1 h 43°C incubation, no obvious change could be observed as shown by , except maybe one or two dead cells. 24 h after the treatment, fragmented blue or pink nuclei could be seen in some cells (), indicating these cells were undergoing apoptosis.
Figure 2. Hoechst/PI staining of MES-SA cells (upper panel) and Dx5 cells (lower panel) treated by incubator hyperthermia. Images were taken either immediately, or 24 h after the treatment. The scale bar represents 8 µm. (A) Control MES-SA; (B) MES-SA immediately after 1 h 43°C incubation; (C) MES-SA 24 h after 1 h 43°C incubation; (D) MES-SA immediately after 30 min 50°C incubation; (E) MES-SA 24 h after 30 min 50°C incubation; (F) control Dx5; (G) Dx5 immediately after 1 h 43°C incubation; (H) Dx5 24 h after 1 h 43°C incubation; (I) Dx5 immediately after 30 min 50°C incubation; (J) Dx5 24 h after 30 min 50°C incubation.
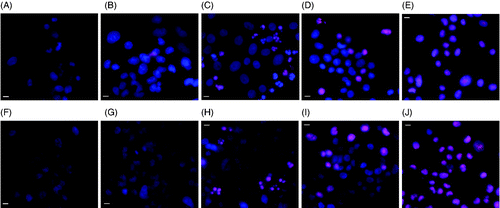
Compared to 1 h 43°C incubation, 30 min 50°C incubation caused more severe damage to the cells. Necrotic cell death could be seen immediately after the treatment (). Although a lot of the cells were not stained by PI at that time, they were severely damaged by the heat stress and eventually died through necrosis as evident by the images taken 24 h after treatment ().
Since Hoechst/PI staining suggested apoptosis in 1 h 43°C incubator hyperthermia-treated cells while necrosis in 50°C hyperthermia-treated cells, quantitative caspase-3 assay was performed on 1 h 43°C incubator hyperthermia-treated cells only ( of the supplementary material) Since cell growth was known to be inhibited 24 h after 1 h 43°C incubator hyperthermia, the caspase-3 fluorescence values at the 24-h time point were normalised to the SRB value to account for changes in cell number. Briefly, caspase-3 data after the treatment were normalised to the ratio of the SRB toxicity values of the treated cells to the SRB toxicity value of the control, which did not receive treatment. Statistically significant difference could be detected at 6 h post treatment in Dx5 cells and 24 h post treatment in MES-SA cells, but the percentage increase in caspase-3 level is only about 25–30%, indicating the extent of apoptosis is only mild, which confirmed the observation in the Hoechst/PI staining assay.
Laser-ICG hyperthermia
The ICG treatment itself (10 µM) without NIR laser had no effect on the cells in terms of cytotoxicity (data not shown). Meanwhile, incubating cells with 10 µM ICG for 1 h and then removing ICG did not cause any temperature increase when the laser was applied (data not included).
In both MES-SA and Dx5 cells, laser-ICG hyperthermia induced necrosis at both 5 µM and 10 µM ICG concentration as shown in . Cells were treated with 5 µM or 10 µM of ICG and excited with NIR laser for 3 min, which produced 43°C and 50°C hyperthermia respectively. The images were taken either 1 h or 24 h after the NIR irradiation. With the increase of thermal dose (more ICG) the fluorescence of PI increased, indicating more severe cell membrane damage.
Figure 3. Hoechst/PI staining of MES-SA cells (upper panel) and Dx5 cells (lower panel) treated by laser excited (3 min) ICG (10 µM). Images were taken either 1 h after, or 24 h after the treatment. The scale bar represents 8 µm. (A) Control MES-SA; (B) MES-SA 1 h after laser irradiation; (C) MES-SA 24 h after laser irradiation; (D) MES-SA 1 h after laser irradiation; (E) MES-SA 24 h after laser irradiation ; (F) control Dx5; (G) Dx5 1 h after laser irradiation; (H) Dx5 24 h after laser irradiation; (I) Dx5 1 h after laser irradiation; (J) Dx5 24 h after laser irradiation.
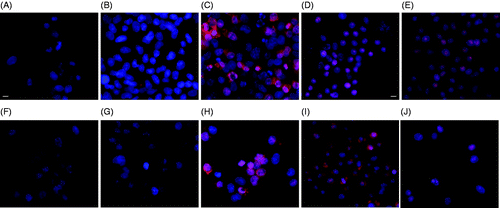
In the previous section, mild apoptosis is observed in both cells 24 h after the slow rate 1 h 43°C incubator hyperthermia. Here it is shown that when the heating is rapid the same thermal dose (5 µM ICG + 3 min laser) caused cell necrosis instead of apoptosis in 24 h, as evident by . Similar to the 1 h 43°C incubation treatment, there is no significant morphological change immediately after 5 µM laser-ICG hyperthermia.
When comparing to the lower thermal dose (), at higher dose both slow rate (incubator) and rapid rate (3 min laser + 10 µM ICG) hyperthermia led to necrosis in almost all the cells 24 h after treatment (). However, the effect of the rapid rate laser-ICG hyperthermia is also more rapid because it caused complete cell necrosis immediately after the treatment () whereas there are still some cells possessing intact cell membrane immediately after the 30 min 50°C incubation. No significant apoptosis could be detected in either MES-SA or Dx5 cells up to 24 h post treatment using the quantitative caspase-3 assay (shown in the supplementary material, Figure 3), which confirmed the observation made in the Hoechst/PI test.
DOX uptake
and show the DOX fluorescence intensity and subcellular localisation in MES-SA and Dx5 cells after the two hyperthermia treatments. Images were taken either 1 h after the hyperthermia treatment or 24 h post treatment. Generally, the DOX fluorescence intensity increased with increasing incubation time, as the images taken 24 h after the treatment were generally brighter than the ones taken immediately after the treatment.
Figure 4. DOX fluorescence in MES-SA cells treated by only DOX (A and F), DOX + 1 h 43°C incubation (B and G), DOX + 30 min 50°C incubation (C and H), DOX + 3 min laser/5 µM ICG (D and I), and DOX + 3 min laser/10 µM ICG incubation (E and J). Images were taken either 1 h after (A, B, C, D and E), or 24 h after the treatment (F, G, H, I and J). The scale bar represents 8 µm.
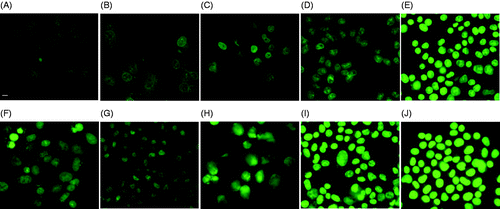
Figure 5. DOX fluorescence in Dx5 cells treated by only DOX (A and F), DOX + 1 h 43°C incubation (B and G), DOX + 30 min 50°C incubation (C and B), DOX + 3 min laser/5 µM ICG (D and I), and DOX + 3 min laser/10 µM ICG incubation (E and J). Images were taken either 1 h after (A, B, C, D and E), or 24 h after the treatment (F, G, H, I and J). The scale bar represents 8 µm.
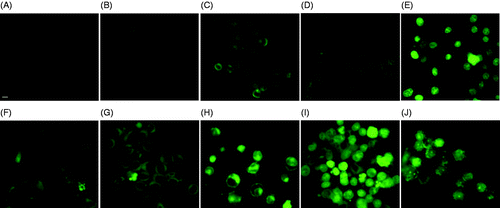
For MES-SA cells (), the fluorescence intensity and subcellular localisation of DOX did not change significantly after the hyperthermia treatment, which is reasonable because MES-SA is DOX-sensitive and DOX can diffuse freely into the cell, therefore the facilitating effect of hyperthermia to DOX is not obvious.
Compared to MES-SA, DX5 cells DOX showed a different subcellular localisation pattern. As shown in , the cell membrane was stained by DOX fluorescence while the nucleus compartment remained dark. This is because the P-gp pump anchored in the cell membrane of Dx5 cells pumps out any DOX molecule diffusing through the membrane. Meanwhile, enhanced DOX uptake by incubator hyperthermia was obvious in Dx5 cells (). Although more DOX accumulated in the Dx5 cells after 1 h 43°C incubation (), the subcellular localisation of DOX did not change compared to the DOX only treatment (), indicating the P-gp pump activity was not compromised. The increased DOX fluorescence intensity could be due to increased cell membrane permeability or increased DOX diffusion rate under hyperthermia condition. Different DOX subcellular localisation was observed in 30 min 50°C incubator hyperthermia-treated cells. As shown by , most cells showed nucleus staining, suggesting compromised P-gp pump activity immediately after the treatment.
Similar to the incubator hyperthermia-treated Dx5 cells, enhanced DOX uptake was obvious after the laser excited ICG (5 µM) hyperthermia (). DOX fluorescence intensity increased with the increase of thermal dose. It has been shown that 1 h 43°C hyperthermia did not change P-gp pump activity in Dx5 cells. However, at the same thermal dose, the rapid rate laser-ICG hyperthermia caused DOX accumulation in the cell nucleus. As shown in , only a few cells showed a dark cell nucleus. At the higher thermal dose (10 µM ICG), DOX fluorescence was present in almost all the cells, which is similar to the incubator hyperthermia at the same thermal dose.
When comparing the rapid rate laser-ICG hyperthermia to the slow rate incubator hyperthermia, both exhibited the ability to overcome P-gp at high thermal dose; however, at low thermal dose, the laser-ICG hyperthermia showed its superiority in helping DOX bypass P-gp mediated MDR as more DOX entered cell nuclei.
Hsp70 expression after hyperthermia treatment
Hsp70 expression after different types of hyperthermia was quantified 24 h after hyperthermia treatment (). ELISA was repeated three times for each data point shown in the figure; each assay contains two repetitions. According to the assay protocol, the measured absorbance value at 450 nm (Optical Density (OD) 450) represents the amount of Hsp70. Since the cell growth was inhibited after hyperthermia treatment, which may affect the total cellular HSP, the obtained Hsp70 absorption values were corrected using the SRB toxicity absorption value to account for changes in cellular protein for a particular treatment. Briefly, after obtaining cell SRB value, the Hsp70 values from each treatment group were normalised to the ratio of the SRB value from that treatment to the SRB value from the control, that is, from cells that did not receive any treatment. We observed that the baseline Hsp70 level in the MDR positive Dx5 cells is about twice as high as in the non-MDR MES-SA cells when both cells were cultured at normal temperature (37°C). Since the two cells were seeded at the same density and given that they have similar doubling times, this increased baseline Hsp70 expression in the Dx5 cells suggests a correlation between MDR and heat resistance.
Figure 6. Hsp70 expression after different types of hyperthermia treatment (n = 3). Data were normalised as described above. ★Significantly higher cellular Hsp70 level among the three groups by ANOVA test with Bonferroni post-hoc correction.
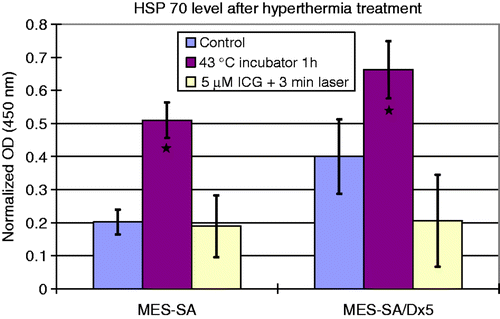
When comparing different hyperthermia treatments, increased Hsp70 expression was observed in 1 h 43°C incubator hyperthermia treatment. The treatment promoted Hsp70 expression in both MDR and non-MDR MES-SA cells. After the treatment the Hsp70 level in Dx5 cells was still higher than in MES-SA cells; however, the percentage increase is higher in MES-SA cells than in Dx5 cells. About a 70% increase was observed in Dx5 cells, whereas the Hsp70 level in MES-SA cells was more than doubled.
P-gp activity after hyperthermia treatment
From the results of previous experiments we can see an increased DOX fluorescence indicating enhanced uptake during hyperthermia conditions in both MES-SA and Dx5 cells. However, the reason for this increase in DOX uptake is not clear. We performed P-gp activity assay to find out whether this increase is due to the inhibition of P-gp drug efflux pump activity in the Dx5 cells. The calcein-AM assay was used to determine whether the increased permeability can help drugs bypass the P-gp MDR system.
MES-SA cells do not over-express P-gp, thus, calcein-AM can diffuse freely into the cell and be cleaved to form bright green fluorescence. As shown in , as expected the induction of P-gp inhibitor verapamil does not increase calcein fluorescence compared to the control. Meanwhile, 1 h 43°C incubator hyperthermia did not change P-gp activity as well, as evident by .
Figure 7. Calcein fluorescence after hyperthermia treatment. (A) MES-SA without any treatment; (B) MES-SA treated by 1 h 43°C incubator hyperthermia; (C) MES-SA treated with verapamil. Exposure time 1000 ms. The scale bar in (A) represents 24 µm.
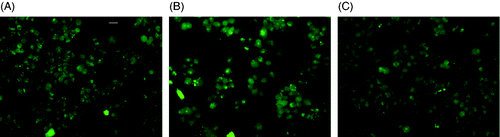
For the Dx5 cells, since it highly over-expresses P-gp, calcein-AM does not remain intracellular, as evident by the low fluorescence in . In , Dx5 cells were treated with the P-gp inhibitor verapamil; as expected, calcein-AM entered the cell and was cleaved to form fluorescent calcein. shows that 1 h 43°C incubator hyperthermia did not reduce P-gp activity as the fluorescence signal was weak and similar to the control cell which did not receive any treatment. The laser exposure to 5 µM ICG for 3 min, on the other hand, caused a slight increase in calcein-AM fluorescence which is consistent with what was seen in the DOX uptake study. This assay indicated that the rapid rate laser-ICG hyperthermia can achieve better P-gp inhibition than the slow rate incubator hyperthermia, resulting in greater DOX accumulation in the cell and its nucleus.
Figure 8. Calcein fluorescence after hyperthermia treatment. (A) Dx5 cells without any treatment; (B) Dx5 cells treated by 1 h 43°C incubator hyperthermia; (C) Dx5 cells treated by 5 µM ICG + 3 min laser; (D) Dx5 treated with verapamil. All the images were taken under 20× magnification and the exposure time was 1000 ms. The scale bar represents 24 µm.
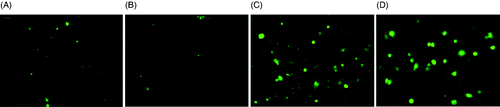
Discussion
Induction of thermotolerance
It has been previously shown by both in vitro and in vivo studies that a rapid rate of heat accumulation can reduce cell survival or increase thermal injury Citation[30–32]. In this regard, some researchers have already tried to correlate cellular Hsp70 level as the biomarker of thermotolerance with different heating rates but none of them achieved positive results. In 1995, Flanagan et al. Citation[30] found that Hsp70 level was significantly higher after rapid rate hyperthermia but not increased after a slow one, a result opposite to ours. The reason for this difference could be that their heating was too slow, even in the rapid rate heating group. In their study, the rapid rate was 0.166°C/min, a rate that is similar to the slow rate (0.1°C/min) used in this study. As a comparison, the rapid rate heating employed in this study was about 4°C/min. From this point of view, their result supported our conclusion.
In this study, it is hypothesised that the rate of heating is a determinant factor in cells acquiring thermal tolerance, which is more likely to occur in low dose hyperthermia with longer exposure time. Therefore, it was proposed that the rapid rate NIR laser-ICG hyperthermia could avoid the induction of thermal tolerance because the duration of treatment is short, while the slow rate incubator hyperthermia could cause thermal tolerance induction. This hypothesis is supported by the results from the Hsp70 ELISA assay where the Hsp70 level after 1 h 43°C hyperthermia was significantly higher than the non-treated cells while at the same thermal dose, the rapid rate 3 min laser + 5 µM ICG hyperthermia did not cause any increase compared to the controls. This difference suggested the importance of the rate of heating because thermotolerance obviously developed to a greater degree during slow heating rates.
Thermal resistance and drug resistance
The HSPs are a family of highly conserved stress proteins present in the cells of all living organisms Citation[33]. Hsp70 can be found expressed at very low levels in healthy cells to help newly synthesised proteins to fold to an appropriate special conformation. Its expression can increase not only when cells are under heat stress but also due to other stresses such as infection, inflammation, or toxin exposure Citation[34]. Our observation that the baseline Hsp70 level in Dx5 cells was twice as high as in MES-SA cells suggests that the induction of MDR might induce Hsp70 over-expression. Dx5 cells are developed by incubating DOX-sensitive MES-SA cells with stepwise increased concentration of DOX, which is a serious stress condition to the cells. It has long been known that the induction of thermal tolerance can simultaneously increase drug resistance. Tumour cells sequentially exposed to non-lethal hyperthermia and then chemotherapy showed higher cell survival than when the two were given simultaneously Citation[19], Citation[35], Citation[36]. Pirity et al. Citation[37] observed that rat hepatoma cells induced to over-express P-gp did not over-express HSPs, whereas cells which were induced to over-express HSPs showed an increased level of P-gp. Consistent with previously reported findings Citation[19], Citation[35–37], our results suggest that hyperthermia should not be given prior to chemotherapy. With the induction of ‘smart’ nanoparticles, such as thermally sensitive polymers/liposomes, the sequence of hyperthermia and chemotherapy can be controlled precisely to avoid heat-induced MDR. Recently, Tchénio et al. Citation[38] successfully isolated heat shock factor 1 (HSF1) transcription factor, which regulates the expression of the HSPs, in cultured human U2-OS osteosarcoma cells that confer DOX resistance. Their results indirectly supported our observation in Dx5 cells that drug resistance might also increase cell thermal tolerance.
Apoptosis in DOX chemotherapy
In the study, 1 µM DOX induced apoptosis in DOX-sensitive MES-SA cells but not in DOX resistant Dx5 cells probably because the intracellular DOX concentration in MES-SA cells was much higher than in Dx5 cells due to the P-gp pump in the Dx5 cells. DOX can cause apoptotic cell death through several distinct mechanisms Citation[39]. DOX-induced apoptosis had been reported in various cell lines and with a wide range of concentrations Citation[40–44]. In a study conducted by Angelini et al. Citation[45], 5 µM and 10 µM DOX concentration induced apoptosis in Dx5 cells. Moreover, they observed increasing apoptosis with increasing DOX intracellular accumulation, which to some extent supports the data presented in this study.
Mode of cell death by hyperthermia
Hyperthermia is capable of inducing apoptosis or necrosis depending on the temperature/thermal dose Citation[4]. Generally, above the ‘breakpoint’ temperature cancer cells start to die because of heat stress. With the increase of temperature the percentage of apoptotic cells decreases with a concomitant increase in necrotic cells Citation[46], Citation[47]. Some other factors can slightly affect this response process, such as cell type, stage of cell cycle and prior exposure to heat Citation[13], Citation[46], Citation[48].
The results of the Hoechst/PI assay on the 50°C-treated cells shows that the two hyperthermia treatments caused complete necrosis both immediately or 24 h after treatment, which is consistent with what was reported in the literature. The results of the Hoechst/PI assay on the 43°C-treated cells support our hypothesis in that rapid rate laser-ICG hyperthermia results in necrosis while slow rate incubator hyperthermia results in apoptosis. Moreover, it indicates for the first time that as long as the rate of heating is rapid necrotic cell death could be induced at a temperature that typically would cause apoptosis. Since a variety of cancer cells have an impaired apoptosis pathway, the ability to induce necrosis by rapid rate heating at 43°C provides more opportunity to treat cancers that are resistant to apoptosis.
DOX-hyperthermia synergism and clinical prospect
Although DOX is considered one of the most effective anticancer agents Citation[49], its clinical use is restricted due to its side effect of inducing cardiomyopathy and congestive heart failure, which is cumulative and irreversible Citation[50]. In order to increase its therapeutic effect as well as minimise its cardiotoxicity to cancer, considerable research has been conducted. Second-generation DOX analogues such as epirubicin or idarubicin showed improved therapeutic index over DOX, but the side effect of cardiotoxicity still exists Citation[51]. Besides the side effects, MDR induction in tumours is another major obstacle to DOX chemotherapy. In order to overcome MDR, drug carriers such as liposomes have been used. Pegylated liposomal DOX has been clinically used to treat advanced ovarian cancer Citation[52]. However, its improvement in response rate was not satisfying (the response rate was less than 30%) Citation[52].
In the previous study Citation[15], we observed a synergistic effect when combining DOX chemotherapy with rapid rate hyperthermia, especially in the MDR expressing Dx5 cells. In the current study we show that the synergistic effect is due to increased uptake of DOX and the reversal of MDR as evident by the DOX fluorescence imaging. Therefore, by adding rapid rate hyperthermia to DOX chemotherapy, a significant reduction in the overall patient dose could be achieved while still reaching the same therapeutic effect compared to when using DOX alone, thus the side effect of DOX could be minimised. More importantly, rapid rate hyperthermia could be a safe approach to help DOX overcome MDR.
Conclusion
This in vitro study in cultured cancer cells shows that the potentiating effect of hyperthermia to chemotherapy can be maximised by increasing the rate of heating. When delivered at a similar thermal dose, rapidly increasing temperature from 37°C to 43°C caused more cell membrane damage than when gradually heating the cells from 37°C to 43°C and thus allowed for more intracellular accumulation of the chemotherapeutic agent DOX. Different modes of cell death were observed under low thermal dose hyperthermia by the two hyperthermia delivery methods. The rapid rate laser-ICG hyperthermia at 43°C caused cell necrosis whereas the slow rate incubator hyperthermia at 43°C induced very mild apoptosis. At 43°C a positive correlation between thermal tolerance and the length of hyperthermia exposure was identified. More importantly, this study shows that by increasing the rate of heating, less thermal dose is needed in order to overcome P-gp-mediated MDR.
Declaration of interest: This work was partly funded by a Florida International University Dissertation Year Fellowship to Yuan Tang. The authors alone are responsible for the content and writing of the paper.
References
- Mostafa EM, Ganguli S, Faintuch S, Mertyna P, Goldberg SN. Optimal strategies for combining transcatheter arterial chemoembolization and radiofrequency ablation in rabbit VX2 hepatic tumors. J Vasc Intervent Radiol 2008; 19: 1740–1748
- Taeger G, Grabellus F, Podleska LE, Mèuller S, Ruchholtz S. Effectiveness of regional chemotherapy with TNF-alpha/melphalan in advanced soft tissue sarcoma of the extremities. Int J Hyperthermia 2008; 24: 193–203
- Ko SH, Ueno T, Yoshimoto Y, Yoo JS, Abdel-Wahab OI, Abdel-Wahab Z, et al. Optimizing a novel regional chemotherapeutic agent against melanoma: Hyperthermia-induced enhancement of temozolomide cytotoxicity. Clin Cancer Res 2006; 12: 289–297
- Kampinga HH. Cell biological effects of hyperthermia alone or combined with radiation or drugs: A short introduction to newcomers in the field. Int J Hyperthermia 2006; 22: 191–196
- Gottesman MM. How cancer cells evade chemotherapy: Sixteenth Richard and Hinda Rosenthal Foundation Award Lecture. Cancer Res 1993; 53: 747–754
- Riordan JR, Deuchars K, Kartner N, Alon N, Trent J, Ling V. Amplification of P-glycoprotein genes in multidrug-resistant mammalian cell lines. Nature 1985; 316: 817–819
- Liu Y, Cho CW, Yan X, Henthorn TK, Lillehei KO, Cobb WN, et al. Ultrasound-induced hyperthermia increases cellular uptake and cytotoxicity of P-glycoprotein substrates in multi-drug resistant cells. Pharm Res 2001; 18: 1255–1261
- Huang CH, Li YP, Cao PG, Xie ZX, Qin ZQ. Synergistic effect of hyperthermia and neferine on reverse multidrug resistance in adriamycin-resistant SGC7901/ADM gastric cancer cells. J Huazhong Univ Sci Tech Med Sci 2011; 31: 488–496
- Bidwell GL, Davis AN, Fokt I, Priebe W, Raucher D. A thermally targeted elastin-like polypeptide-doxorubicin conjugate overcomes drug resistance. Investig New Drug 2007; 25: 313–326
- Moriyama-Gonda N, Igawa M, Shiina H, Wada Y. Heat-induced membrane damage combined with adriamycin on prostate carcinoma PC-3 cells: Correlation of cytotoxicity, permeability and P-glycoprotein or metallothionein expression. Br J Urology 1998; 82: 552–559
- Pietzner K, Schmuck RB, Fotopoulou C, Gellermann J, Ismaeel F, Cho CH, et al. Long term combination treatment with bevacizumab, pegylated liposomal doxorubicin and regional abdominal hyperthermia in platinum refractory ovarian cancer: A case report and review of the literature. Anticancer Res 2011; 31: 2675–2677
- Feyerabend S, Stevanovic S, Gouttefangeas C, Wernet D, Hennenlotter J, Bedke J, et al. Novel multi-peptide vaccination in HLA-A2+ hormone sensitive patients with biochemical relapse of prostate cancer. Prostate 2009; 69: 917–927
- Hildebrandt B, Wust P, Ahlers O, Dieing A, Sreenivasa G, Kerner T, et al. The cellular and molecular basis of hyperthermia. Crit Rev Oncol Hematol 2002; 43: 33–56
- Christophi C, Winkworth A, Muralihdaran V, Evans P. The treatment of malignancy by hyperthermia. Surg Oncol 1998; 7: 83–90
- Tang Y, McGoron AJ. Combined effects of laser-ICG photothermotherapy and doxorubicin chemotherapy on ovarian cancer cells. J Photochem Photobiol B Biol 2009; 97: 138–144
- Li GC, Mivechi NF, Weitzel G. Heat shock proteins, thermotolerance, and their relevance to clinical hyperthermia. Int J Hyperthermia 1995; 11: 459–488
- Anderson RL, Herman TS, van Kersen I, Hahn GM. Thermotolerance and heat shock protein induction by slow rates of heating. Int J Radiat Oncol Biol Phys 1988; 15: 717–725
- Herman TS, Sweets CC, White DM, Gerner EW. Effect of heating on lethality due to hyperthermia and selected chemotherapeutic drugs. J Natl Cancer Inst 1982; 68: 487–491
- Hahn GM, Strande DP. Cytotoxic effects of hyperthermia and adriamycin on Chinese hamster cells. J Natl Cancer Inst 1976; 57: 1063–1067
- Ponce AM, Vujaskovic Z, Yuan F, Needham D, Dewhirst MW. Hyperthermia mediated liposomal drug delivery. Int J Hyperthermia 2006; 22: 205–213
- Mackay JA, Chilkoti A. Temperature sensitive peptides: Engineering hyperthermia-directed therapeutics. Int J Hyperthermia 2008; 24: 483–495
- Yarmolenko PS, Zhao Y, Landon C, Spasojevic I, Yuan F, Needham D, et al. Comparative effects of thermosensitive doxorubicin-containing liposomes and hyperthermia in human and murine tumours. Int J Hyperthermia 2010; 26: 485–498
- Kampinga HH. Hyperthermia, thermotolerance and topoisomerase II inhibitors. Br J Cancer 1995; 72: 333–338
- Ciocca DR, Oesterreich S, Chamness GC, McGuire WL, Fuqua SA. Biological and clinical implications of heat shock protein 27 000 (Hsp27): A review. J Natl Cancer Institute 1993; 85: 1558–1570
- Oh HJ, Chen X, Subjeck JR. Hsp110 protects heat-denatured proteins and confers cellular thermoresistance. J Biol Chem 1997; 272: 31636–31640
- Fisher B, Kraft P, Hahn GM, Anderson RL. Thermotolerance in the absence of induced heat shock proteins in a murine lymphoma. Cancer Res 1992; 52: 2854–2861
- Sapareto SA, Dewey WC. Thermal dose determination in cancer therapy. Int J Radiat Oncol Biol Phys 1984; 10: 787–800
- Rezai KA, Farrokh-Siar L, Ernest JT, van Seventer GA. Indocyanine green induces apoptosis in human retinal pigment epithelial cells. Am J Ophthalmol 2004; 137: 931–933
- Wolfe JD, Csaky KG. Indocyanine green enhanced retinal vessel laser closure in rats: Histologic and immunohistochemical observations. Exp Eye Res 2004; 79: 631–638
- Flanagan SW, Ryan AJ, Gisolfi CV, Moseley PL. Tissue-specific Hsp70 response in animals undergoing heat-stress. Am J Physiol-Regulat Integr Compar Physiol 1995; 268: R28–R32
- Burns CP, Lambert BJ, Haugstad BN, Guffy MM. Influence of rate of heating on thermosensitivity of L1210 leukemia – membrane-lipids and Mr 70 000 heat-shock protein. Cancer Res 1986; 46: 1882–1887
- Herman TS, Gerner EW, Magun BE, Stickney D, Sweets CC, White DM. Rate of heating as a determinant of hyperthermic cyto-toxicity. Cancer Res 1981; 41: 3519–3523
- Amorim FT, Yamada PM, Robergs RA, Schneider SM, Moseley PL. The effect of the rate of heat storage on serum heat shock protein 72 in humans. Eur J Appl Physiol 2008; 104: 965–972
- De Maio A. Heat shock proteins: Facts, thoughts, and dreams. Shock 1999; 11: 1–12
- Donaldson SS, Gordon LF, Hahn GM. Protective effect of hyperthermia against the cytotoxicity of actinomycin D on Chinese hamster cells. Cancer Treat Rep 1978; 62: 1489–1495
- Wallner K, Li GC. Adriamycin resistance, heat resistance and radiation response in Chinese hamster fibroblasts. Int J Radiat Oncol Biol Phys 1986; 12: 829–833
- Pirity M, Hever-Szabo A, Venetianer A. Overexpression of P-glycoprotein in heat- and/or drug-resistant hepatoma variants. Cytotechnol 1996; 19: 207–214
- Tchénio T, Havard M, Martinez LA, Dautry F. Heat shock-independent induction of multidrug resistance by heat shock factor 1. Mol Cell Biol 2006; 26: 580–591
- Thorburn A, Frankel AE. Apoptosis and anthracycline cardiotoxicity. Mol Cancer Therapeut 2006; 5: 197–199
- Ling YH, Priebe W, Perez-Soler R. Apoptosis induced by anthracycline antibiotics in P388 parent and multidrug-resistant cells. Cancer Res 1993; 53: 1845–1852
- Skladanowski A, Konopa J. Adriamycin and daunomycin induce programmed cell death (apoptosis) in tumour cells. Biochem Pharmacol 1993; 46: 375–382
- Zaleskis G, Berleth E, Verstovsek S, Ehrke MJ, Mihich E. Doxorubicin-induced DNA degradation in murine thymocytes. Mol Pharmacol 1994; 46: 901–908
- Bose R, Verheij M, Haimovitz-Friedman A, Scotto K, Fuks Z, Kolesnick R. Ceramide synthase mediates daunorubicin-induced apoptosis: An alternative mechanism for generating death signals. Cell 1995; 82: 405–414
- Jaffrâezou JP, Levade T, Bettaèieb A, Andrieu N, Bezombes C, Maestre N, et al. Daunorubicin-induced apoptosis: Triggering of ceramide generation through sphingomyelin hydrolysis. EMBO J 1996; 15: 2417–2424
- Angelini A, Iezzi M, Di Febbo C, Di Ilio C, Cuccurullo F, Porreca E. Reversal of P-glycoprotein-mediated multidrug resistance in human sarcoma MES-SA/Dx-5 cells by nonsteroidal anti-inflammatory drugs. Oncol Rep 2008; 20: 731–735
- Samali A, Holmberg CI, Sistonen L, Orrenius S. Thermotolerance and cell death are distinct cellular responses to stress: Dependence on heat shock proteins. FEBS Lett 1999; 461: 306–310
- Milleron RS, Bratton SB. 'Heated' debates in apoptosis. Cell Mol Life Sci 2007; 64: 2329–2333
- Bettaieb A, Averill-Bates DA. Thermotolerance induced at a mild temperature of 40°C protects cells against heat shock-induced apoptosis. J Cell Physiol 2005; 205: 47–57
- Weiss RB. The anthracyclines: Will we ever find a better doxorubicin?. Semin Oncol 1992; 19: 670–686
- Singal PK, Li T, Kumar D, Danelisen I, Iliskovic N. Adriamycin-induced heart failure: Mechanism and modulation. Mol Cell Biochem 2000; 207: 77–86
- Minotti G, Menna P, Salvatorelli E, Cairo G, Gianni L. Anthracyclines: Molecular advances and pharmacologic developments in antitumor activity and cardiotoxicity. Pharmacol Rev 2004; 56: 185–229
- Rose PG. Pegylated liposomal doxorubicin: Optimizing the dosing schedule in ovarian cancer. Oncologist 2005; 10: 205–214