Abstract
Purpose: The present study aims to create and characterise a cell-embedding tissue-mimicking material (TMM) that has thermal and acoustic properties similar to liver tissue, in order to enable study and optimisation of protocols for ultrasound-induced hyperthermia and drug delivery.
Materials and methods: An agarose-based, cell-embedding TMM was iteratively developed and characterised. The acoustic properties (attenuation coefficient, speed of sound and cavitation threshold) and thermal response of the material were compared with those of fresh degassed liver tissue over a range of acoustic pressures and frequencies. A luminescence intensity assay was used to evaluate viability of HuH-7 cells in the material. The efficacy of ultrasound-mediated chemotherapeutic treatment in the material was tested by localised activation of low temperature thermally sensitive liposomes. Drug activation was measured by fluorescence microscopy.
Results: Similar acoustic properties (attenuation coefficient, speed of sound) to liver tissue were achieved over the therapeutically relevant frequency range of 1–4 MHz and similar thermal response was achieved for acoustic pressures up to 4.8 MPa peak to peak (ppk) at 1.1 MHz. Above 4.8 MPa ppk cavitation enhanced heating occurred in the TMM. Drug release from low-temperature-sensitive liposomes was achieved with 4.4 MPa ppk 6-s exposures at 1.1 MHz and cell compatibility of the material was confirmed.
Conclusions: A platform for in vitro work for activation of thermally sensitive liposomes using high intensity focused ultrasound (HIFU)-induced hyperthermia was established. The TMM presents similar acoustic properties and thermal response to liver tissue over a broad range of ultrasound exposure conditions.
Introduction
High intensity focused ultrasound (HIFU) has been developing rapidly as a therapeutic modality. The thermal effects of HIFU have been exploited for non-invasive tissue ablation Citation[1], Citation[2] and, more recently, for drug delivery in combination with novel thermosensitive drug carriers Citation[3–6]. This creates a need for the development of suitable platforms for in vitro studies. Tissue-mimicking materials (TMM) enable repeatable and controlled large parametric studies and are thus preferable as a first in vitro experimental approach. The necessary TMM properties sought are (1) stability, (2) cell-compatibility, (3) non-exothermic preparation, so that no drug activation occurs in the TMM during preparation and (4) acoustic properties and thermal response similar to liver.
Previously developed generations of materials include agarose gels Citation[7–11], polyvinyl alcohol gels (PVA) Citation[12–14], polyacrylamide gels (PAA) Citation[15–17] and silicone Citation[18]. Among these, agarose gel was the most promising for experiments with low-temperature-sensitive drug carriers because it has a low gelling temperature (below 37°C) which allows it to be mixed with temperature-sensitive ingredients without compromising their stability. Also, unlike other materials, agarose is not cytotoxic and permits cell growth Citation[19]. Because literature values of attenuation in pure agarose gel are much lower than in tissue Citation[18], particles have been incorporated in the agarose gels to increase effective attenuation. Agarose-based TMM with dispersed powdered graphite has been widely used, because its acoustic characteristics can be easily controlled in the manufacturing process Citation[7], but the toxicity of the graphite particles renders it ineffective for studies involving cells. Matsukawa et al. Citation[9] did a comparative study of the attenuation and speed of sound in particle-compounded agarose gels. The particles were talc powder, glass beads and graphite. Unlike talc particles and graphite, glass beads are cell-compatible. The concentrations of glass beads used were found to yield values of acoustic attenuation coefficients much larger than in tissue, indicating that for the right concentration of glass particles, attenuation and thus heating response similar to tissue can be achieved.
In the present work, a stable, non-exothermic, cell-embedding tissue model is iteratively developed and characterised, with the aim of achieving similar acoustic properties and thermal response to liver tissue. Varying number densities of glass spheres are embedded in gels formed using a range of agarose concentrations, which are subsequently characterised. The acoustic properties that are directly measured include attenuation coefficient and inertial cavitation threshold, because they are the most relevant to mild ultrasonic hyperthermia induced at moderate HIFU pressure amplitudes Citation[20–25]. Cell viability is also assessed by measuring the luminescence intensity for each of the TMM compositions. The temperature rises achieved during HIFU exposure in the material, which best mimics the acoustic properties of tissue, are also compared to heating in freshly excised bovine liver tissue under the same exposure conditions. Finally, TMM performance is tested as a potential test platform for ultrasound-enhanced drug delivery by embedding low-temperature-sensitive liposomes (ThermoDox, Celsion, Lawrenceville, NJ) loaded with doxorubicin, a commonly used chemotherapeutic agent, and exposing the gels to mild HIFU-induced hyperthermia.
Materials and methods
Material composition
Agarose (UltraPure™ Agarose 1000, Invitrogen, Paisley, UK) with a low setting temperature (35°C) was used as a base for the TMM. Agarose powder was suspended in phosphate buffered saline (PBS), the solution was heated in a 1000 W microwave oven until it came to the boil and then cooled down to 37°C. The low gelling temperature allowed for sustaining the mixture at 37°C in liquid form and mixing low-temperature-sensitive agents, without triggering drug release. For culturing cells, the mixture was then diluted 1:1 in colourless high glucose culture medium (DMEM high glucose, w/o phenol red, PAA, Yeovil, UK). The agarose concentrations tested were 5 mg/mL and 10 mg/mL total. Polydisperse soda lime glass microspheres (Duke Scientific, Spherical Glass Materials, Palo Alto, CA) were added to a concentration in the range 0.44% v/v–4% v/v to increase acoustic attenuation. Human hepatocellular carcinoma cells (HuH-7) were embedded in the phantom in a concentration of 8 × 105 cells/mL. Before being mixed with the gel they were centrifuged and diluted in colourless DMEM, for minimisation of background fluorescence. The mixture was subsequently poured into a Lucite phantom holder and care was taken not to induce bubbles in the gel or the gel/Mylar interface, which would compromise the experiment.
Liposomal doxorubicin
Low-temperature-sensitive liposomes (ThermoDox) were embedded in the TMM to evaluate the effectiveness of the phantom as a platform for ultrasound-induced hyperthermia drug-release experiments. ThermoDox comprises low-temperature-sensitive liposomes loaded with doxorubicin at a concentration of 2 mg/mL. When the liposomes are heated at temperatures in the range 39°C to 42°C, the integrity of the lipid bilayers is compromised and the encapsulated anticancer agent is locally released within the heated area. The therapeutically relevant concentration of ThermoDox that was used in this work is 0.02 mg/mL, as recommended by the manufacturer.
Attenuation and speed of sound measurements
Two unfocused 2.25 MHz transducers, one in the transmitting and one in the receiving end, were submerged in a water tank filled with deionised and degassed water at 37°C and the TMM was interposed between them. The transmitting transducer was driven by a signal generator (33220A, Agilent Technologies, Santa Clara, CA) at 20 cycles, 10 V peak-to-peak (ppk) over the frequency range of 1–4 MHz. The acoustic signal was transmitted through the TMM, detected by the second 2.25 MHz unfocused transducer and subsequently analysed with custom-made Labview software. Losses in signal amplitude were due to sound attenuation in water, attenuation in the TMM and reflections at the interfaces between water and the front wall and back wall of the material.
Reflection losses at the material surface boundaries were accounted for by comparing material samples of 10 cm and 5 cm thickness (n = 4) so that there is a high enough signal to noise ratio. As reflection losses at the surface boundaries were the same in both samples, any difference in the signal received across the two samples was solely due to differences in the propagation path length. The acoustic distance was kept constant in both cases by adjusting the physical distance of the transducers accordingly. The attenuation coefficient was calculated via the relationship , where P1 and P2 (MPa) were the pressure amplitudes acquired at two different depths in the material, x (m) is the distance between them and α (Np/m) is the attenuation coefficient. The speed of sound was measured by the difference in time delay between the two materials. The same process was performed with degassed bovine liver tissue (n = 3), which was obtained from a local abattoir and used within 8 h of excision.
HIFU exposure
The experimental set-up shown in was used for sample insonation, inertial cavitation detection and temperature measurements Citation[26], Citation[27]. A narrowband single element HIFU transducer (HIFU 102E035, Sonic Concepts, Bothell, WA) with 62.64 mm focal length and centre frequency of 1.1 MHz was used for thermal and cavitational characterisation of the TMM. The output of the transducer was characterised using a 0.075-mm diameter needle hydrophone (SN1360, Precision Acoustics, Dorchester, UK). All subsequently quoted values refer to ppk focal pressure (MPa) as obtained on the basis of this calibration.
Figure 1. Block diagram of the experimental setup for temperature measurements and cavitation detection. The cell and liposome-embedding tissue-mimicking material was immersed in a water tank maintained at 37°C. A thermocouple was inserted in the material and aligned to the HIFU focus, to enable monitoring of the focal temperature throughout the exposure. Acoustic emissions were monitored with a Passive Cavitation Detector (PCD) confocally aligned with the High Intensity Focused Ultrasound (HIFU) transducer. The continuous, dashed and dotted lines indicate the sonication, cavitation-detection and temperature measurement loops respectively.
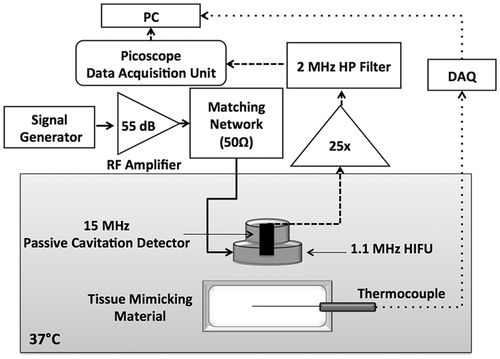
Cavitation detection
The HIFU transducer had a 20 mm central opening, through which a passive cavitation detector (PCD) was mounted. The PCD was a spherically focused single element transducer with 7.5-MHz centre frequency and 75-mm focal length and was used for recording the acoustic emissions during each HIFU exposure. The HIFU transducer had a 20-mm central opening, through which a PCD could be coaxially mounted. The PCD was a spherically focused single element transducer with a 7.5-MHz centre frequency and a 75-mm focal length that was used to detect acoustic emissions generated within the HIFU focus during ultrasound exposure. Prior to each experiment, a previously described alignment procedure was used to ensure coincidence of the HIFU and PCD foci.
The PCD signal was amplified by a factor of 25 using a low-noise broadband preamplifier (SR445A, Stanford Research Systems, Sunnyvale, CA) and then filtered with a Butterworth filter (SN3944, Krohnhite, Brockton, MA), which was set to act as a 2-MHz high-pass filter, to reject the fundamental HIFU frequency, before being connected to an 8-bit Picoscope (PicoScope 3205, Pico Technology, St Neots, UK) that digitised PCD data with a sampling frequency of 25 MHz. Inertial cavitation activity, associated with broadband noise emissions Citation[28], was classified and quantified on the basis of the frequency content of the acquired acoustic emissions, which was calculated by taking fast Fourier transforms (FFT) of the PCD time traces Citation[29]. It was assumed that the energy contained within harmonic bands arises from non-linear propagation, and that broadband energy (outside harmonic bands) was indicative of inertial cavitation.
Temperature measurement
Each of the TMM samples (n = 4) and the degassed liver tissue samples (n = 3) was exposed to a set of 6-s continuous wave (CW) sonications in the pressure range of 2.4 to 8.8 MPa ppk. A T-type fine-needle thermocouple (HYP0, Omega Engineering, Stamford, CN), coated with a stainless steel hypodermic needle of outer diameter 200 µm, was embedded in the TMM for focal temperature measurements. The signal from the thermocouple was recorded with a multiplexer with embedded reference temperature compensation (34970A, Agilent Technologies), which sampled and digitised the thermocouple signal 100 times per second before passing the value to a PC. The thermocouple was inserted into the TMM from the side with its longitudinal axis in an approximately horizontal orientation. Because the flexibility of the fine-needle thermocouple made it difficult to position it in a precisely horizontal location, the TMM was aligned with respect to the HIFU transducer only after insertion of the thermocouple. The presence of a foreign body, in this case the thermocouple, at the HIFU focus is on its own a source of error, due to the interaction of the HIFU beam with the thermocouple needle. An additional local temperature rise occurs due to the viscous forces that arise from the relative motion between the thermocouple and the surrounding medium as well as from the heat conduction between the thermocouple and the medium Citation[30], Citation[31]. Hynynen et al. Citation[32] have reported that ultrasound sonications at 1 MHz, yielding temperature rises in the range of 5–8°C in vivo, induce a 0.3–1°C additional temperature rise due to thermocouple interference, for thermocouples of diameter up to 300 µm. In the present work, it is assumed that a thermocouple artefact of 1°C or less is induced in all cases.
Cell viability and doxorubicin release from the liposomes
Cell viability in the TMM was measured with a luminescent cell viability assay (CellTiter-Glo, Promega, Southampton, UK), a method of determining the number of viable cells in the sample by quantisation of the adenosine triphosphate (ATP) present, which is an indicator of metabolically active cells. The sample volume was 125 µL, n = 4 and the tested combinations were 1% (mg/mL) agarose with 0%, 2% and 4% (mL/mL) glass microspheres. The samples were added in a 96-well plate, mixed with 125 µL of the reagent and examined after 1 and 2 h. Cell viability was determined by the luminescence intensity of the samples, which is directly correlated to the number of cells. Luminescence intensity was subsequently compared to the luminescence intensity of cells in PBS at the same concentration. In all cases the background luminescence of 1% agarose TMM without cells was subtracted.
Doxorubicin release from the liposomes was measured with fluorescence microscopy (excitation/emission 487/590 nm). Doxorubicin has the inherent ability to fluoresce when released from the liposomes, whereas when encapsulated in the liposomes it self-quenches and does not fluoresce. Doxorubicin release was identified in the ThermoDox-embedding TMM after HIFU exposure at 4.4 MPa ppk for 6 s by sampling the gel (n = 4) and measuring fluorescence intensity of the sample at the exposed and non-exposed areas.
Results
Particle characterisation
The size distribution of the glass microspheres was confirmed based on the laser diffraction technique with a particle size analyser (Mastersizer, Malvern, UK) and found to fit a Gaussian distribution of mean 27.44 and standard deviation 9.78. The optimal attenuation coefficient was achieved with polydispersed soda lime glass microspheres of diameter 5–50 µm, at a concentration of 4% (v/v).
Attenuation coefficient and speed of sound
The experimental results for attenuation in degassed liver at 37°C are presented in as mean values with error bars representing the standard deviation. The black line represents the linear fit to the experimental data. The dotted and dashed lines represent the upper and lower limit of the reported literature values for liver attenuation in mammals Citation[33–35], and α = 6.67f Np/m is a representative linear relationship of attenuation against frequency for ox-liver tissue in vitro as reported by Duck et al. Citation[36]. The linear fit to experimental measurements in the frequency range 1–4 MHz was calculated to be α = 8.3f − 2.2 Np/m and the speed of sound was found to be c = 1614 ± 17.7 m/s, in the range 1570–1660 m/s, which is reported in the literature Citation[33–35]. The attenuation coefficient of liver at 1.1 MHz (frequency of interest because it is the central frequency of the therapeutic transducer) was found to be α = 6.93 Np/m, which is in the range of 5.17–7.964 Np/m that is found in the literature [33–36].
Figure 2. Experimental data for degassed bovine liver at 37°C and literature values for liver tissue in mammals. The experimental results are shown as mean values and error bars of size double the standard deviation. The dotted and dashed lines represent the upper and lower limits for mammalian liver attenuation as reported in the literature. The continuous line shows a representative linear approximation for ox liver attenuation from Duck et al. [36].
![Figure 2. Experimental data for degassed bovine liver at 37°C and literature values for liver tissue in mammals. The experimental results are shown as mean values and error bars of size double the standard deviation. The dotted and dashed lines represent the upper and lower limits for mammalian liver attenuation as reported in the literature. The continuous line shows a representative linear approximation for ox liver attenuation from Duck et al. [36].](/cms/asset/4e50579c-ddd4-4ca0-b0a9-52d0477b1476/ihyt_a_762553_f0002_b.gif)
Subsequently, the agarose and glass microsphere concentrations that would give similar values of attenuation and speed of sound in the TMM were identified. As shown in , the difference in attenuation measured for TMM made with 0.5% or 1% agarose was insignificant. shows the change in the attenuation with frequency for four different concentrations of glass microspheres; 0.5%, 1%, 2% and 4%. A 4% concentration of microspheres in the TMM yielded a mean value of the attenuation coefficient that fell within the range of the attenuation values measured for tissue, and a standard deviation that was smaller than the standard deviation of measured tissue attenuation values (). The speed of sound was found to be 1545 ± 2.1 m/s.
Figure 3. Measured values of attenuation coefficient versus frequency with the through-transmission method in a 0.5% and 1% agarose tissue-mimicking material (TMM) with 2% glass microspheres (A) and in a 1% agarose TMM with 0.44%, 1%, 2% and 4% glass microspheres (B). The 4% microsphere TMM presents significantly higher attenuation over the whole frequency range.
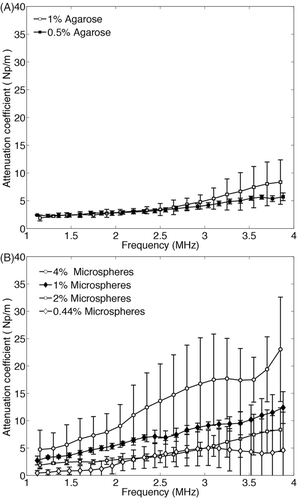
Figure 4. Comparison of the experimental values of the attenuation coefficient of degassed liver (n = 3), the TMM (n = 4) and plain agarose gel. The TMM exhibits a much higher attenuation than the plain agarose gel, with similar attenuation profile to the degassed liver over the frequency range of 1–4 MHz.
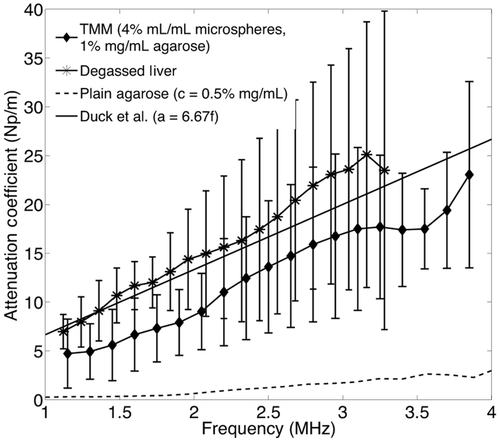
Cavitation threshold
shows representative results for the frequency content of the recorded emissions from liver tissue and the TMM for 2.2, 4.4 and 8.8 MPa ppk acoustic pressures. Degassed liver presented linear response at very low sonications (2.2 MPa ppk), whereas at higher exposures non-linear behaviour was observed (4.4 and 8.8 MPa ppk). Non-linear response is indicated by the presence of harmonic emissions and was expected, because liver tissue is known to be a highly non-linear medium with a nonlinearity parameter (B/A) ranging from 6.2 to 8.9 Citation[34]. Evidence of non-linear scattering is seen in the TMM for even lower incident pressure amplitudes (2.2 MPa ppk), because of the presence of glass spheres, which can act as strong scatterers of the incident wave. At 4.4 MPa ppk, harmonic emissions were dominating, but broadband noise was also observed, indicating onset of inertial cavitation activity. From 4.4 to 8.8 MPa ppk a 50-fold increase in the broadband noise was observed. The acoustic emissions acquired from the TMM are two orders of magnitude higher than the emissions acquired from liver tissue.
Figure 5. Representative FFTs of the signal received after exposure of degassed liver (left) and the novel TMM (right) to 6s continuous wave HIFU sonications at 1.1 MHz. (A), (C) and (E) correspond to 2.2, 4.4 and 8.8 MPa peak to peak HIFU exposures on liver tissue respectively. At 2.2 MPa no harmonic or broadband emissions are observed, whereas at 4.4 and 8.8 MPa there are harmonic emissions indicating non-linearity of the medium. No inertial cavitation activity is observed at any frequency. (B), (D) and (F) correspond to the same acoustic pressures applied to the TMM. Non-linearity of the medium can be seen by the high levels of harmonic emissions even at the low pressure of 2.2 MPa peak to peak. At 4.4 MPa, there are both harmonic and broadband emissions, with harmonic emissions dominating, whereas broadband emissions dominate at 8.8 MPa.
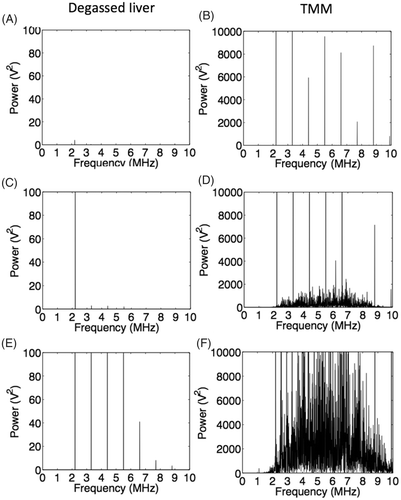
The threshold for cavitation-enhanced heating of the TMM was evaluated from the temperature data that were acquired. In the absence of inertial cavitation, temperature elevation at the HIFU focus follows a linear profile with respect to the acoustic intensity of the sonication Citation[37], but when inertial cavitation was initiated, cavitation-enhanced heating caused irregularities in the temperature profile and thus deviation from the linear profile Citation[23]. In , the maximum temperature rise that was recorded at the HIFU focus during 6-s CW sonications in the range of 2.5–5.6 MPa ppk HIFU excitation, with 0.5 MPa steps, is plotted against the respective peak acoustic intensity for representative samples of both liver tissue and the TMM.
Figure 6. Effect of inertial cavitation activity on temperature rise at the HIFU focus in the TMM and the degassed tissue, during 6s exposures at 1.1 MHz. At 1.8 · 106 W · m−2 (corresponding to 4.8 MPa ppk), the maximum temperature rise caused to the TMM was almost 10°C higher than the one predicted by the linear model.
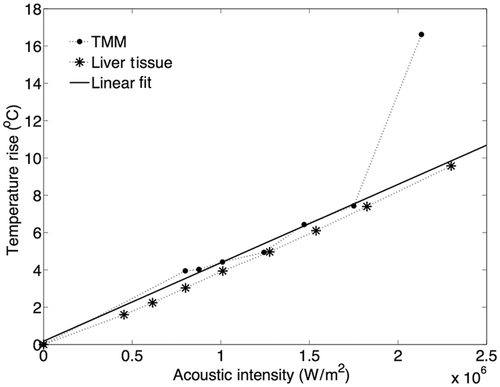
Thermal response
For acoustic pressures up to 4.4 MPa ppk, the mean values of the temperature rise between liver tissue and the TMM agree to within 0.5°C and the standard deviations of the temperature rise agree to within 1°C, as shown in and . Above 4.4 MPa ppk heating in the TMM was significantly different from degassed liver tissue, due to the onset of cavitation-enhanced heating ( and ).
Figure 7. Temperature measurements at the HIFU focus during 6 s exposures at 1.1 MHz at three pressures below the cavitation threshold: 2.5, 3.4 and 4.4 MPa ppk, (A) in the liver and (B) the tissue-mimicking material (TMM) and three pressures above the cavitation threshold: 4.8, 5.6 and 6.6 MPa ppk in (C) the liver and (D) the TMM. Exposures at 4.4 MPa ppk produce hyperthermia in the range over which low-temperature sensitive liposomes release their content. At pressures above 4.8 MPa ppk, the presence of inertial cavitation activity causes significant increase in the temperature rise in the TMM (D), resulting in discrepancy from the temperature rises observed in liver (C) in the absence of inertial cavitation. For experiments in the liver tissue n = 3 and for experiments in the TMM n = 4.
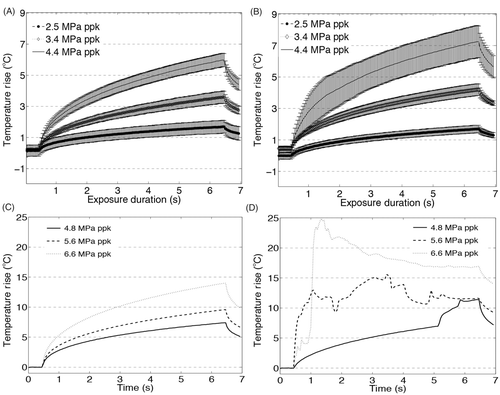
In order to characterise the thermal response of the TMM not only in terms of the peak temperature rise on the HIFU axis, but also in terms of heat conduction off-axis, temperature rises were recorded at various locations across the HIFU beam in both liver and the TMM at 4.4 MPa ppk focal pressure (). The temperature rise on-axis was found to be highest both in liver and in the TMM, and to decrease symmetrically off-axis. The cross-sectional area in the tissue and TMM that is above 40°C was found to be 0.79 mm2 and 0.9 mm2 respectively at 2 s, 1.60 mm2 and 1.74 mm2 respectively at 4 s, and 3.38 mm2 and 5.08 mm2 respectively at 6 s. This suggests that heat diffusivity in the two materials is comparable.
Cell compatibility
Cell viability was represented as a percentage of cell viability of cells in PBS (). In all cases the mean value of cell viability was between 80% and 120%, suggesting cell-compatibility of the TMM. Increasing luminescence intensity is observed with increasing concentration of glass microspheres. This could be an outcome of the microspheres acting as light scatterers, hence increasing the luminescence signal of the sample. Variation in luminescence could also be attributed to variation in the initial concentration of cells in each sample.
Drug release
Low-temperature-sensitive liposomes release their content over the narrow range of 39–45°C, with a maximum at 42°C. Since the experiments were conducted at 37°C to mimic physiological body temperature, the 5–8°C temperature rise demonstrated in following a 4.4 MPa sonication led to a final temperature of 42–45°C, which was sufficient for triggered drug release, whereas a 2.5 MPa sonication led to a 1°C temperature rise, inadequate for drug release. shows drug release from ThermoDox after continuous wave HIFU exposure at 4.4 MPa ppk for 6 s, which results in subsequent cell death () and no release or cell death after continuous wave exposure at 2.5 MPa ppk. Higher values of fluorescence intensity at the exposed areas with respect to the non-exposed indicate liposomal activation at the elevated temperatures due to HIFU excitation.
Figure 9. Cell viability in 1% agarose TMM with 0%, 2% and 4% (mL/mL) glass microspheres measured as a percentage of the luminescence intensity of cells in the various TMM compositions with respect to the luminescence intensity of cells in PBS (n = 4). In all cases, the background luminescence (luminescence of TMM without cells) was subtracted.
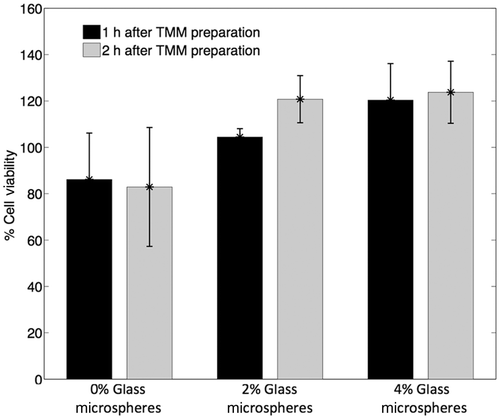
Figure 10. (A) Assessment of cell viability after exposing the liposome-embedding phantom to ultrasound conditions that cause and do not cause mild hyperthermia (4.4 MPa ppk, 6 s CW and 2.5 MPa ppk, 6 s CW respectively). Cell viability is measured by luminescence intensity, as a percentage of the luminescence intensity of areas of the phantom not exposed to HIFU (n = 4). (B) Assessment of doxorubicin release from the liposomes under the same conditions, measured by the fluorescence intensity of released doxorubicin (n = 4). No cell death is induced unless sufficient levels of hyperthermia are reached to release the drug.
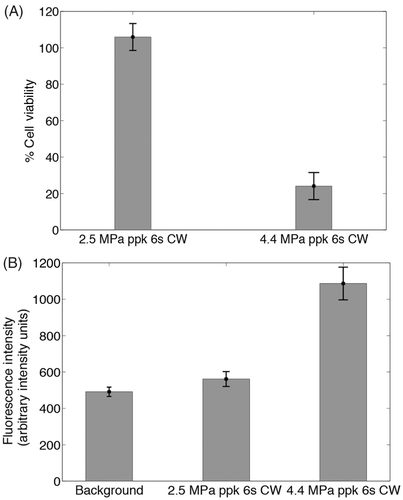
Discussion
The aim of this work was to produce a TMM with acoustic and thermal properties similar to liver tissue that yields a similar rate of heating under HIFU excitation as would be experienced in the liver, which can be utilised as a platform for ultrasound-induced mild hyperthermia and drug delivery experiments. For this reason it should exhibit similar acoustic properties to liver tissue and, most importantly, similar heating behaviour due to HIFU exposures, as this was the principal goal.
In , the size of the error bars in the measurements for liver attenuation could be attributed to the fact that liver is a highly heterogeneous tissue and thus there are structural differences within different samples, as well as within the same sample. Apart from that, before each experiment the tissue was cut into square pieces of the size of the holder in which they were mounted. As this procedure was done by hand, there could be slight variations in the thickness of the samples, or the curvature of their sides that were exposed to ultrasound.
The size of the error bars in the TMM attenuation results could be attributed either to intrinsic experimental errors of the attenuation measurement technique, or to entrapped bubbles and small variations in the local number density of the glass microspheres along particular ultrasound propagation paths. The existence of 5 µm to 50 µm microspheres renders the gel inhomogeneous. A variation of the local concentration (or size) of the glass microspheres along particular sound propagation paths could cause a variation in the measured attenuation values across different paths. As can be observed in , more than doubling of the local concentration from 0.44% to 1% in the TMM can cause significant difference in the measured attenuation value. Moreover, the phantom was not degassed before the experiments, as that would be unfavourable for the viability of the embedded cells.
Ultimately, the large error bars in the attenuation coefficient measurements shown in do not affect the highly reproducible heating curves obtained below the cavitation threshold in . It is therefore hypothesised that much of the variation in the attenuation coefficient measurements that come from local variations in glass sphere concentrations along particular propagation paths do not affect bulk heating of the material. Also, the temperature measurement method is less prone to experimental error than the attenuation measurement method. As and indicate, the divergence in thermal response above 4.4 MPa relates to the onset of inertial cavitation activity in the TMM, an effect not observed in the degassed liver tissue even at pressures as high as 8.8 MPa ppk. Although the cavitation threshold of the TMM is significantly lower than the threshold of degassed liver, the actual cavitation threshold of an in vivo liver remains unknown and it is likely that the degassing procedure may have further increased the liver cavitation threshold. The TMM is thus found to be a good platform for studies of ultrasound-induced mild hyperthermia on the basis of both the similarity in acoustic properties and thermal response of tissue for pressures of up to 4.4 MPa ppk at 1 MHz.
In this study, low-temperature-sensitive liposomes were used to demonstrate that the TMM can be used as a platform for successful activation of particles that are sensitive in the mild hyperthermia range. The successful release of the encapsulated drug from the liposomes () in addition to the non-exothermic preparation procedure of the material support the idea that the TMM can be used with any carriers that release encapsulated drugs in mild hyperthermic conditions and not only with liposomes. Moreover, the similar heating pattern between the TMM and the degassed liver, shown in , and the similar porosity that agarose has to tissue Citation[38–40] support the hypothesis that the spatial distribution of regions where the local temperature exceeds that required for release will be similar in both materials.
As stated in the Pennes bioheat transfer equation Citation[41], blood perfusion plays a significant role in heat dissipation in biological tissues because it creates a heat-sink effect. In the present work, the effect of perfusion was neglected, because the TMM was compared to and matched the acoustic properties and thermal response of non-perfused degassed liver tissue. Further experiments have to be conducted for the characterisation of a TMM that incorporates vessels and blood flow.
Conclusion
In this work, a cell-embedding TMM which has acoustic properties similar to those of liver tissue was developed and tested. The TMM was manufactured in a non-exothermic process, which renders it appropriate for use in mild hyperthermia studies, and found to be cell-compatible. The values of its speed of sound and acoustic attenuation coefficient over a range of frequencies (1–4 MHz) were also measured and found to be in the same range as the values for freshly excised liver tissue. Most importantly, the TMM exhibits almost identical heating response to liver tissue upon exposure to 1.1 MHz HIFU for focal pressure amplitudes of up to 4.4 MPa ppk, beyond which cavitation-enhanced heating was observed. Both cells and thermosensitive liposomes containing doxorubicin were successfully embedded within the phantom, and the liposomes remained stable until exposed to mild HIFU-induced hyperthermia. It is concluded that the newly developed TMM represents a viable, reproducible and meaningful test medium for studies of HIFU-induced mild hyperthermia and drug release.
Acknowledgements
The authors would like to thank James Fisk and Roger Lewis for their assistance in manufacturing the various sample holders and experimental components, and Manish Arora for fruitful discussions.
Declaration of interest: The authors thank Celsion Corporation for generously supplying ThermoDox. The authors gratefully acknowledge the support of the UK's Engineering and Physical Sciences Research Council (grant EP/EP/F011547/1) and of the Oxford Biomedical Research Centre funded by the National Institute for Health Research. The authors alone are responsible for the content and writing of the paper.
References
- Wu F, Wang ZB, Cao YD, Chen W, Bai J, Zou J, et al. A randomised clinical trial of high-intensity focused ultrasound ablation for the treatment of patients with localised breast cancer. Br J Cancer 2003; 89: 2227–2233
- Wu F, Wang ZB, Chen WZ, Wang W, Gui Y, Zhang M, et al. Extracorporeal high intensity focused ultrasound ablation in the treatment of 1038 patients with solid carcinomas in China: An overview. Ultrason Sonochem 2004; 11: 149–154
- McDannold N, Fossheim SL, Rasmussen H, Martin H, Vykhodtseva N, Hynynen K. Heat-activated liposomal MR contrast agent: Initial in vivo results in rabbit liver and kidney1. Radiology 2004; 230: 743–752
- Staruch R, Chopra R, Hynynen K. Localised drug release using MRI-controlled focused ultrasound hyperthermia. Int J Hyperthermia 2011; 27: 156–171
- De Zwart J, Salomir R, Vimeux F, Klaveness J, Moonen C. On the feasibility of local drug delivery using thermo-sensitive liposomes and MR-guided focused ultrasound. Paper presented at the International Society for Magnetic Resonance in Medicine conference, Denver, CO, USA, 1–7 April 2000. p. 43
- Dromi S, Frenkel V, Luk A, Traughber B, Angstadt M, Bur M, et al. Pulsed-high intensity focused ultrasound and low temperature-sensitive liposomes for enhanced targeted drug delivery and antitumor effect. Clin Cancer Res 2007; 13: 2722–2727
- Burlew M, Madsen E, Zagzebski J, Banjavic R, Sum S. A new ultrasound tissue-equivalent material. Radiology 1980; 134: 517–520
- Madsen EL, Frank GR, Dong F. Liquid or solid ultrasonically tissue-mimicking materials with very low scatter. Ultrasound Med Biol 1998; 24: 535–542
- Matsukawa M, Akimoto T, Ueba S, Otani T. Ultrasonic wave properties in the particle compounded agarose gels. Ultrasonics 2002; 40: 323–327
- Holt RG, Roy RA. Measurements of bubble-enhanced heating from focused, MHz-frequency ultrasound in a tissue-mimicking material. Ultrasound Med Biol 2001; 27: 1399–1412
- Brewin MP, Pike LC, Rowland DE, Birch MJ. The acoustic properties, centered on 20 MHz, of an IEC agar-based tissue-mimicking material and its temperature, frequency and age dependence. Ultrasound Med Biol 2008; 34: 1292–1306
- Kharine A, Manohar S, Seeton R, Kolkman RGM, Bolt RA, Steenbergen W, et al. Poly (vinyl alcohol) gels for use as tissue phantoms in photoacoustic mammography. Phys Med Biol 2003; 48: 357–370
- Manohar S, Kharine A, van Hespen JCG, Steenbergen W, van Leeuwen TG. Photoacoustic mammography laboratory prototype: Imaging of breast tissue phantoms. J Biomed Optics 2004; 9: 1172–1181
- Devi CU, Vasu R, Sood A. Design, fabrication, and characterization of a tissue-equivalent phantom for optical elastography. J Biomed Optics 2005; 10: 044020
- Takegami K, Kaneko Y, Watanabe T, Maruyama T, Matsumoto Y, Nagawa H. Polyacrylamide gel containing egg white as new model for irradiation experiments using focused ultrasound. Ultrasound Med Biol 2004; 30: 1419–1422
- Lafon C, Zderic V, Noble ML, Yuen JC, Kaczkowski PJ, Sapozhnikov OA, et al. Gel phantom for use in high-intensity focused ultrasound dosimetry. Ultrasound Med Biol 2005; 31: 1383–1389
- Howard S, Yuen J, Wegner P, Zanelli CI. Characterization and FEA simulation for a HIFU phantom material. IEEE Symposium on Ultrasonics 2003; 2: 1270–1273
- Zell K, Sperl J, Vogel M, Niessner R, Haisch C. Acoustical properties of selected tissue phantom materials for ultrasound imaging. Phys Med Biol 2007; 52: N475–N484
- Chen Q, Suki B, An KN. Dynamic mechanical properties of agarose gels modeled by a fractional derivative model. J Biomech Eng 2004; 126: 666–671
- Nyborg WL. Heat generation by ultrasound in a relaxing medium. J Acoust Soc Am 1981; 70: 310–312
- Nyborg WL. Sonically produced heat in a fluid with bulk viscosity and shear viscosity. J Acoust Soc Am 1986; 80: 1133–1139
- Hill CR, Bamber JC, ter Haar GR (eds). Physical principles of medical ultrasonics. Chichester: John Wiley & Sons, 2004
- Coussios CC, Roy RA. Applications of acoustics and cavitation to noninvasive therapy and drug delivery. Annu Rev Fluid Mech 2008; 40: 395–420
- Coussios CC, Farny C, Ter Haar G, Roy R. Role of acoustic cavitation in the delivery and monitoring of cancer treatment by high-intensity focused ultrasound (HIFU). Int J Hyperthermia 2007; 23: 105–120
- Hynynen K. The threshold for thermally significant cavitation in dog's thigh muscle in vivo. Ultrasound Med Biol 1991; 17: 157–169
- Kennedy P, Arora M, Coussios C-C. Localization and interpretation of bubble activity during HIFU exposure. In: Proceedings of the 8th International Symposium on Therapeutic Ultrasound. Minneapolis, MN: American Institute of Physics, 2009, pp. 68–72. Available from: http://adsabs.harvard.edu/abs/2009AIPC.1113...68K
- Hockham N, Coussios CC, Arora M. A real-time controller for sustaining thermally relevant acoustic cavitation during ultrasound therapy. IEEE Trans Ultrason Ferroelec Freq Control 2010; 57: 2685–2694
- Leighton TG. The Acoustic Bubble. Academic Press, London 1994
- Ilyichev V, Koretz V, Melnikov N. Spectral characteristics of acoustic cavitation. Ultrasonics 1989; 27: 357–361
- Fry WJ, Fry RB. Determination of absolute sound levels and acoustic absorption coefficients by thermocouple probes – Experiment. J Acoust Soc Am 1954; 26: 311–317
- Fry WJ, Fry RB. Determination of absolute sound levels and acoustic absorption coefficients by thermocouple probes – Theory. J Acoust Soc Am 1954; 26: 294–310
- Hynynen K, Martin C, Watmough D, Mallard J. Errors in temperature measurement by thermocouple probes during ultrasound induced hyperthermia. Br J Radiology 1983; 56: 969–970
- Goss S, Johnston R, Dunn F. Compilation of empirical ultrasonic properties of mammalian tissues. II. J Acoust Soc Am 1980; 68: 93–108
- Law W, Frizzell L, Dunn F. Determination of the nonlinearity parameter B/A of biological media. Ultrasound Med Biol 1985; 11: 307–318
- Techavipoo U, Varghese T, Chen Q, Stiles T, Zagzebski J, Frank G. Temperature dependence of ultrasonic propagation speed and attenuation in excised canine liver tissue measured using transmitted and reflected pulses. J Acoust Soc Am 2004; 115: 2859–2865
- Duck FA. Physical Properties of Tissue: A Comprehensive Reference Book. Academic Press, London; San Diego 1990
- Pierce AD. Acoustics: An Introduction to its Physical Principles and Applications. Acoustical Society of America, Melville, NY 1989
- Braet F, Wisse E. Structural and functional aspects of liver sinusoidal endothelial cell fenestrae: A review. Comp Hepatol 2002; 1: 1
- Narayanan J, Xiong JY, Liu XY. Determination of agarose gel pore size: Absorbance measurements vis a vis other techniques. J Phys Conf Ser 2006; 28: 83, Available from: http://iopscience.iop.org/1742-6596/28/1/017
- Pernodet N, Maaloum M, Tinland B. Pore size of agarose gels by atomic force microscopy. Electrophoresis 2005; 18: 55–58
- Pennes HH. Analysis of tissue and arterial blood temperatures in the resting human forearm. J Appl Physiol 1948; 1: 93–122