Abstract
Microwave tissue heating is being increasingly utilised in several medical applications, including focal tumour ablation, cardiac ablation, haemostasis and resection assistance. Computational modelling of microwave ablations is a precise and repeatable technique that can assist with microwave system design, treatment planning and procedural analysis. Advances in coupling temperature and water content to electrical and thermal properties, along with tissue contraction, have led to increasingly accurate computational models. Developments in experimental validation have led to broader acceptability and applicability of these newer models. This review will discuss the basic theory, current trends and future direction of computational modelling of microwave ablations.
Introduction
Thermal ablation technology is a rapidly emerging alternative to surgical resection for many benign and malignant tumours of the liver, lung, kidney and bone [Citation1–5]. Ablation is also being investigated for the treatment of other solid tumours in organs such as the breast, prostate, adrenal glands, pancreas and uterus [Citation6–12]. Non-oncological applications include treatment of cardiac arrhythmia, as well as a means for haemostasis during surgical resection [Citation13–15]. Ablation may also be combined with adjuvant therapies such as radiation, chemotherapy and even nanoparticles to improve treatment efficacy [Citation16–22].
Methods of inducing thermal ablation include lasers, high intensity focused ultrasound, radiofrequency electrical current and microwaves. The applied energy heats the target tissue to cytotoxic temperatures, usually above 50–60 °C, although microwave can heat tissue to over 150 °C [Citation23]. Due to its minimally invasive nature, thermal ablation is characterised by quicker recoveries and fewer complications compared to surgical resection [Citation24]. Microwave ablation is gaining attention as an alternative to the more widely disseminated radiofrequency ablation, due to several advantages in tissue-heating physics. Microwave energy is capable of propagating through desiccated and charred tissue, which allows for continuous and rapid volumetric heating, potentially leading to a larger ablation zone compared to radiofrequency ablation [Citation23]. The high heating rate produced by some microwave systems can overcome heat-sink effects from nearby vessels, creating more uniform ablation zones [Citation25]. Microwave antennas can also be tuned to deliver energy more efficiently to specific organ systems such as the liver or lung [Citation26,Citation27].
Computational modelling of microwave ablation procedures plays a critical role in the development and implementation of clinical systems. Numerical simulations can rapidly predict the propagation of electromagnetic (EM) waves from antennas of arbitrary geometry and evaluate antenna performance metrics [Citation28]. Energy deposition from the applied electromagnetic fields, heat generation, as well as the subsequent water and vapour mass transfer in tissue can then be used to predict the thermal dose or surviving fraction of cells [Citation29–32]. Together, coupled electromagnetic-thermal solutions may improve predictions of how ablation zones form inside patients.
The in silico nature of computational modelling of thermal ablations provides a highly controlled environment to investigate and understand the effects of changing input variables [Citation33]. Such specific insights can potentially lead to more focused experimental studies, fewer animal studies, decreased developmental costs and greater research efficiency. Faster and more powerful computational techniques can help predict and improve microwave heating in patient-specific models. Analysis of antenna geometry and materials can also be used to optimise device design and implementation.
This paper will reflect upon the current methods and state of computational modelling for microwave tissue ablation. We will briefly review the relevant physics and basic numerical models. We will then address complexities unique to microwave tissue heating, such as the effects of high temperature on tissue properties and how resulting changes in energy delivery can be incorporated into a computational model. Lastly, we will discuss the current trends and challenges in translating simulation results into practical application.
Theory
Microwave ablation is produced by electromagnetic (EM) fields radiating from an antenna inserted into the tissue. The EM field is described most generally by the source-free, time-harmonic form of Maxwell’s equation in lossy media such as tissue:
where E is the electric field intensity (V/m), H is the magnetic field intensity (A/m), ω is the angular frequency, μ is the permeability (H/m) and ε is the complex permittivity or dielectric constant (F/m), B is the magnetic flux density (Wb/m2), D is the electric flux density (C/m2). And J is the current density (A/m2). In the case of coaxial microwave antennas in an approximately homogenous, isotropic medium, an axially symmetric cylindrical coordinate system can often be used to reduce the three-dimensional problem to a two-dimensional domain, accelerating the solution computation.
A description of heat transfer in tissues can be realised using Pennes’ formulation of the non-stationary heat equation [Citation34]:
where cp is the specific heat capacity of the tissue (J/kg K), kt is the thermal conductivity (W/m K), T is the temperature (K). Qm is the metabolic heat generated from the tissue (W/m3) but usually ignored due to its minimal impact compared to the other heat terms. Qp represents heat loss through blood perfusion,
where ωb is the blood perfusion velocity (kg/m3 s), cbl is the specific heat capacity of blood (J/kg K) and Tbl is the blood temperature (K). The remaining term of Equation 5 is the heat absorbed by the electromagnetic field into the tissue,
where E is the electric field (V/m) solution found previously through solving Maxwell’s equations, and σ is the tissue conductivity (S/m) [Citation23,Citation35]. Solving this set of equations provides a visual representation for how tissue temperature changes during a microwave ablation procedure (). The equations can also be solved simultaneously or iteratively to account for changing tissue properties; for example those that are dependent on temperature or water content.
Figure 1. (A) Model of temperature profiles during microwave ablations in liver tissue at various time points. The ablation zone temperature boundary grows more in the first 3 min, compared to the last 2 min. (B) Temperature elevation through the transverse cross section of ablation zone at various time points. Microwave ablation zones can reach temperatures as high as 150 °C. (C) SAR mapping of a microwave ablation zone along with corresponding temperature isotherms at 5 min.
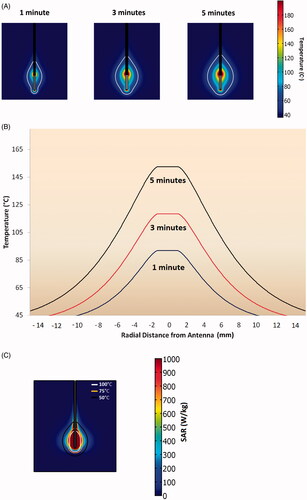
Although tissue damage is composed of many complex biological processes, there are two widely accepted models that predict thermal damage as a single simplified process: the thermal iso-effective model and the Arrhenius damage model [Citation36,Citation37]. The thermal iso-effective model describes how cellular damage is related to cumulative thermal dose; that is, the relationship between temperature and exposure time. The cumulative equivalent minutes at 43 °C (CEM43) is a common metric that equates the likelihood of cellular death to that of cells exposed to 43 °C for a specified number of minutes [Citation38,Citation39]. The Arrhenius damage model, using similar assumptions as the CEM model, expresses the percentage of cells in a given volume that will survive at a given temperature and exposure time. However, there are limits in quantifying thermal damage, especially at the higher temperatures seen in thermal ablations compared to mild or moderate hyperthermia [Citation36,Citation40]. Models that describe thermal damage due to cellular or metabolic disruption have also been described but have not been as widely utilised to date due to complex inputs and high computational costs [Citation41,Citation42].
Analytical solutions to Equations 1–7 are available for only certain antenna geometries and assumptions about the medium, such as a homogenous tissue environment. For more general models, numerical methods are required. The most commonly used techniques include the finite element method (FEM), the finite difference time domain method, and the method of moments. The FEM has been used extensively due to its availability in commercial software such as COMSOL and ANSYS, which are capable of coupling electromagnetic field solutions to other physics models such as heat transfer and diffusion.
Uses of computational modelling in microwave ablation
Antenna evaluation
Common microwave ablation antenna performance metrics include those that describe how efficiently an antenna can deliver power into the tissue and the resulting heating pattern. Antenna efficiency can be described by the ratio of power output to power input, or reflection coefficient Γ,
where Pr(f) is the reflected power and Pin is the input power (W). More efficient power delivery is characterised by lower reflection coefficients. Specific absorption rate (SAR), which represents the rate of power deposition in tissue, is given as
where E is the electric field (V/m), σ is the tissue conductivity (S/m) and ρ is the liver tissue density (kg/m3) [Citation35,Citation25]. SAR can roughly estimate potential shape of an ablation zone created by a given antenna ().
With transient analysis of coupled EM-thermal problems, it is also possible to observe the changes in reflection coefficient and SAR occurring during the ablation process. Using these metrics, automated optimisation techniques can be employed to find microwave antenna geometries that suit a specific clinical need. Due to the multiple factors involved in antenna design, the final design is usually the result of a trade-off between the objective heating pattern and reflection coefficient [Citation43–45]. Improving ablation antenna design remains an area of active research [Citation46–49].
Power delivery frequencies
In addition to facilitating computer-aided antenna design, computational modelling can also be used to evaluate the effect of the applied frequency during power delivery. Current microwave ablation generators provide continuous power, from 30–180 W at 915 MHz or 2.45 GHz, although higher and lower frequencies have been described [Citation50–54]. While some previous studies suggested that lower frequencies are associated with greater penetration depth and larger ablation zones, power output was not normalised to offset cable losses at each frequency [Citation55,Citation56]. When modelling the dipole antennas used in those studies, and controlling for applied power, we found virtually no difference in ablation SAR diameter between 915 MHz and 2.45 GHz (). This result confirmed the conclusions of an earlier microwave hyperthermia antenna study [Citation57]. When comparing antenna designs in a controlled environment with varying powers, computational modelling can play a critical role.
Figure 2. (A) Numerical modelling demonstrating nearly equivalent maximum ablation zone diameters between dipole antenna designs emitting power at 2.45 GHz (right) and 915 MHz (left). The colour bars represent the heating rate from 0--1000 W/kg. The white line iso-contour gives a visual approximation to the ablation heating zone. (B) The heating rate normalised by density, also known as the specific absorption rate (SAR). Microwave antenna performance at the two frequencies shows nearly equivalent SARs at radial distances 7 mm from the antenna.
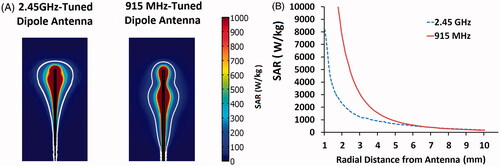
Multiple antenna optimisations
Modelling has also been utilised to analyse and optimise antenna arrays for microwave tissue heating. Such arrays can produce larger, more homogenous ablation zones than with a single antenna [Citation58–62]. As with single antennas, computational models can predict the electromagnetic interactions between antennas and resulting heating pattern in tissue. Zones of constructive and destructive wave interference that can affect relative heating rates have been of particular interest. Computational modelling can help optimise the input wave amplitude and phase to produce deeper tissue penetration and improved local control of the tumour ablation zone [Citation63–65]. Temporal modulation of the amplitude and phase of each antenna has also been analysed during computational modelling [Citation66,Citation67]. Placement of phased antennas in non-parallel fashion while maintaining the same homogenous ablation zone has also been explored [Citation68]. Array optimisation with modelling has led to increased efficacy in treating more irregularly shaped tumours that are deep within the body and provide more options in the number of antennas placed and their insertion points [Citation35,Citation69–72].
Tissue property changes during thermal ablation
Dielectric properties of various tissues are strongly influenced by temperatures and tissue water content [Citation73]. The dielectric properties of tissue include relative permittivity εr, which describes the tissue’s ability to hold electrical charge, and effective conductivity σ, a measure of how well the tissue absorbs electromagnetic energy. The dielectric properties of biological tissue can be described across a wide frequency domain by the multi-pole Cole–Cole dispersion model:
where τ is a time constant to characterise the polarisation in a relaxation region, ε∞ is the permittivity at field frequencies where
, Δε is the magnitude of dispersion, α is a measure of the broadening of the dispersion and σs is the static conductivity [Citation74].
The temperature dependence of dielectric permittivity and conductivity in tissue has been documented in multiple studies. Within a narrow range around physiological temperatures (20–45 °C), temperature dependence can be reasonably described as a linear function [Citation75,Citation76]. Parabolic temperature-dependent models have also been described in the range of 20–60 °C [Citation77]. Microwave ablation systems, however, are capable of heating tissue to temperatures well over 100 °C [Citation27,Citation78]. At these higher temperatures tissues undergo structural changes and water loss, which affect tissue properties substantially [Citation38,Citation79,Citation80]. By lumping physical phenomena such as water vaporisation and tissue dehydration into a temperature-dependent model and using coupled EM-thermal simulations, more accurate temperature profiles can be predicted, even at temperatures over 100 °C. One study compiled a table of dielectric properties of tissue which were measured at the end of a microwave ablation with various sets of power and time [Citation81]. The results were fitted to a Cole–Cole model and suggested significant changes in the amount of dispersion, time constant and static conductivity values during heating [Citation82]. Two other studies monitored the dielectric property values during high temperature microwave heating of ex vivo bovine liver, revealing a sigmoidal relationship between permittivity and conductivity with temperature [Citation83,Citation84]. These curves were then applied to the computer simulations and found to be more accurate in predicting experimental temperatures when compared to existing models [Citation83]. The temperature-dependent model also more accurately described changes in antenna reflection coefficient and heating pattern caused by non-uniform changes in the tissue dielectric property during ablation. Establishing the relationship between dielectric properties and heating physics is an ongoing topic of research that holds promise for even more accurate simulations.
Trends in microwave ablation modelling
Microwave ablation technology development has undergone rapid growth in the last decade and there are multiple areas of active research that utilise the benefits of numerical modelling. The effect of individual parameters on the entire ablation process can be predicted prior to experimental work, saving time and resources for more focused study [Citation85]. But despite the advantages of working with simulations and drawing upon nearly a century of research in electromagnetic theory, there is much work needed with regard to the inputs and the validation of existing numerical models.
Water vaporisation and tissue contraction in tissue
Intense heating during microwave ablation leads to physical changes such as water vaporisation, vapour mass diffusion and re-condensation. This is evident during experimental studies with microwave antennas in tissue, where vapour formation can be observed with imaging during longer and more powerful ablations. The centre of the ablation zone typically becomes dehydrated first, and the water is driven outwards from the antenna. Vapour formation and transport leads to dehydration of the tissue around the ablation zone [Citation3,Citation38,Citation78–80,Citation86]. Two separate groups tried improving the match between modelling and experimental results by incorporating a latent heat of vaporisation term to account for the energy needed to change water from its liquid to gas phase within tissue [Citation78,Citation87]. However, there was a rapid decrease in heating rate after 100 °C and a high temperature SAR correction term was required to match the model to the experiments. One possible explanation for this model mismatch is a change in the EM field profile secondary to changes in permittivity and conductivity as noted in other works [Citation79,Citation83]. Incorporating the dielectric changes due to tissue dehydration in the ablation treatment may yield a more accurate model to predict the physical changes occurring during an ablation treatment.
Another important phenomenon affecting the accuracy of computational modelling is the contraction of tissue exposed to ablative temperatures. One previous study had demonstrated that ablation zones contract up to 15–50% in diameter and 27–75% in volume during radiofrequency and microwave ablation. The same study implemented an exponential correction term to the computational model to compensate for the 20–25% radial contraction at various distances from the antenna after 5 min [Citation88]. Greater contraction was noted during microwave ablations than RF ablations, likely due to increased water vaporisation and collagen dehydration (). Contraction can complicate the validation of numerical and experimental results because temperature sensors placed into the tissue will move during contraction, artificially increasing temperatures compared to static points in a computational model. Contraction can also confound imaging-based diagnostic and post-ablation staging efforts by shrinking the original boundaries of the tumour. Accurate comparison of numerical simulations and experimental results is possible only by accounting for such contractions [Citation83].
Figure 3. (A) Ex vivo tissue contraction is shown by placing pairs of high density wire markers 10–40 mm away from the antenna shaft. The original marker positions are shown in the top image and begin to move closer together as the microwave ablation begins and continues shrinking until the end of the procedure. (B) Graph showing distances between marker pairs decreasing during the ablation. Contraction is most rapid in the first 2–3 min.
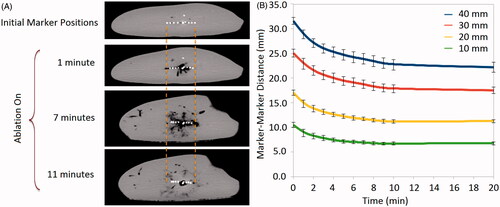
Model validation
Experimental validation of computational model predictions is critical to establish broader applicability of the model. Visual inspection and correlation of experimental ablation zone dimensions to numerical predictions is one validation technique. Blanching and desiccation inside the ablation zone are good predictors of cell death in many parenchymal tissues such as the liver (). Further histological analysis such as microscopic examination, enzyme histochemistry and transmission electron microscopic analysis can help stratify various regions of ablation [Citation89–91]. These techniques compare cell death to the predictors of cytotoxicity calculated during simulation. However, such comparisons rely on the numerical metric of cell death, which can vary widely by method [Citation36].
Figure 4. (A) Microwave ablation created in ex vivo liver tissue. The charred zone is the darkened area at the centre of the ablation zone which was highly desiccated from the intense heat and water vaporisation (>100 °C). The coagulation zone is the blanched region of the ablation zone, usually showing pyknotic nuclei on histology (70–100 °C). The congestive zone is the red peripheral area of the ablation zone that represents the boundary of the ablation zone and is sometimes called the transition zone (50–70 °C). (B) Intra-procedural imaging of a monopole antenna during an in vivo ablation procedure. The central hypo-dense zone identifies water vaporisation. (C) Gas bubbles resulting from water vaporisation are visible on ultrasound imaging and can be used to monitor treatment progress.
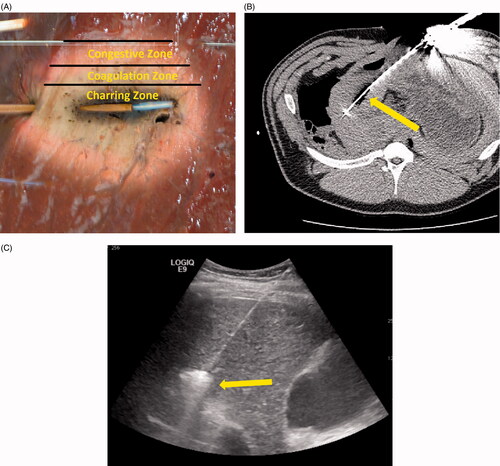
Alternatively, arrays of thermocouples or fibre-optic temperature probes have been successfully used to map temperatures around the ablation antenna [Citation83,Citation87,Citation92]. These temperature probes are structurally robust, exhibit quick response times and provide enough temporal resolution in real-time. Temperature data can be recorded and correlated spatially and temporally with the modelling data. Since contraction can change the location of the temperature probes, it is important to similarly recalibrate the evaluation points in the simulation output or establish a temperature–contraction relationship in the model to accurately compare numerical and experimental results [Citation83,Citation88].
Imaging techniques such as ultrasound, CT or MRI can also be used to validate computational models [Citation93–95]. Contrast-enhanced scans delineate boundaries of the coagulation zone compared to perfused tissues and can be used to compare ablation zone size to predicted sizes [Citation94]. Non-contrast scans with sufficient temporal resolution can be used to monitor ablation zone growth, but thermal ablation features can still be difficult to discern. One more prominent feature is water vapour formation, which appears as hyper-echoic gas bubbles under ultrasound and hypo-dense regions under CT. The visible gas is not a surrogate for the ablation zone, but can provide an estimate of the most desiccated zone of tissue. The highly visible gas can also be tracked during the ablation to estimate vapour transport (). However, water vapour formation and transport must be calculated in the computational model to use gas as the validation metric. Alternatively, MR thermometry can be used to quantify the temperature change in tissue experimentally for comparison with numerical predictions, but most studies have been targeted toward radiofrequency ablation measurements [Citation96–98]. Current microwave devices are not MR compatible so the utility of MR thermometry has been limited [Citation99–101].
Extension to patient-specific planning
Treatment planning is most typically performed by the attending physician and is, therefore, heavily dependent on physician experience. Patient-specific treatment planning based on computational modelling may be able to assist in the physicians’ decision-making process. Ablation precision would theoretically improve as physicians would be able to recognise whether a given antenna placement could achieve the necessary margins, but also potentially avoid critical structures that could lead to procedural complications [Citation102].
While information needed to create patient-specific antenna guidance systems is available, there are still multiple challenges that need to be addressed before being integrated into hospital workflow. Cooling effects from vasculature can disrupt the growth of a thermal ablation zone, decreasing the efficacy of an ablation treatment and increasing the risk for local recurrence. Previous literature investigating the effect of blood vessels on thermal ablations are based primarily on radiofrequency ablation [Citation103–105]. Studies relating the effects of blood vessels and vascular trees to radiofrequency ablation zone sizes have revealed additional flow mechanics that influence how a thermal ablation zone may appear [Citation106–109]. While the focus of these studies were on radiofrequency ablation, the implications are increasingly relevant for microwave ablations, which are much more efficient at heating tissue and blood vessels. Vessels near microwave ablations have been shown to act as a heat conduit, as opposed to a heat sink for thrombotic events and perivascular damage [Citation110–112]. Understanding the effect of vessel flow and nearby vascular beds in thermal ablations is currently an area of active research.
In addition to the modelling of local anatomy near the antenna placement location, patient-specific planning requires detailed knowledge of many other parameters such as the thermal and electrical properties of the organ and surrounding tissue that may be affected by underlying disease processes [Citation113,Citation114]. Accurately integrating these additional facets into clinically useful information in the hospital workflow in real-time has led to the development of ranking algorithms, image registration and computing reduction techniques. Optimisation algorithms have been used to rank the possible insertion points and find the best antenna placement location, maximising chances of the best clinical outcome and minimising risks for complications [Citation115–117]. To cut down on computing costs, some groups have utilised an objective cost function that optimises the temperature distribution by avoiding larger vasculatures, creating an optimal trajectory of an RFA applicator [Citation104]. Decreasing the computational time of calculating ablation zone behaviour near major vasculatures has also been demonstrated using patient-independent look-up tables. These tables utilise previously calculated cooling rates of blood vessels based on vessel size and distance from an RF applicator. Using these tables to predict ablation zone characteristics was demonstrated to be computationally faster than solving the electromagnetic and heat transfer solutions independently for a patient-specific anatomy [Citation118]. Other efforts to improve computational speed have involved a GPU-driven real-time calculation of the projected ablation zone, while incorporating the cooling effects of nearby liver vasculature [Citation119].
Conclusion
Microwave tumour ablation is a rapidly growing modality already used to treat early stage tumours and gaining traction in combination therapies. The ability to predict the active heating zone and heating behaviour within tissue has led to improved understanding of the basic physics behind microwave ablations. The exquisite control of modelling studies affords in-depth investigation of the effects of single variables, leading to decreased experimental sample sizes and costs. Computational and experimental studies have helped optimise the design of microwave antennas, generators and techniques, facilitating clinical translation and further adoption into clinics worldwide. The concurrent development of modelling and validation will lead to better integration of patient-specific, interactive models that can change how ablation procedures are performed in the future.
Declaration of interest
C.L. Brace is a shareholder and consultant for Neuwave Medical Inc., Madison, WI. The authors alone are responsible for the content and writing of the paper.
Acknowledgements
The authors wish to thank Zhen Ji, PhD for assistance with tissue contraction figures.
References
- De Lope CR, Tremosini S, Forner A, Reig M, Bruix J. Management of HCC. J Hepatol 2012;56:S75–87
- Belghiti J, Carr BI, Greig PD, Lencioni R, Poon RT. Treatment before liver transplantation for HCC. Ann Surg Oncol 2008;15:993–1000
- Brace CL. Radiofrequency and microwave ablation of the liver, lung, kidney, and bone: What are the differences? Curr Probl Diagn Radiol 2009;38:135–43
- Gervais DA, Arellano RS, Mueller P. Percutaneous ablation of kidney tumors in nonsurgical candidates. Oncology (Williston Park) 2005;19:S6–11
- Pusceddu C, Sotgia B, Fele RM, Melis L. Treatment of bone metastases with microwave thermal ablation. J Vasc Interv Radiol 2013;24:229–33
- Tanaka T, Westphal S, Isfort P, Braunschweig T, Penzkofer T, Bruners P, et al. Microwave ablation compared with radiofrequency ablation for breast tissue in an ex vivo bovine udder model. Cardiovasc Interv Radiol 2012;35:914–20
- Zhou W, Zha X, Liu X, Ding Q, Chen L, Ni Y, et al. US-guided percutaneous microwave coagulation of small breast cancers: A clinical study. Radiology 2012;263:364–73
- Montorsi F, Guazzoni G, Colombo R, Galli L, Bergamaschi F, Rigatti P. Transrectal microwave hyperthermia for advanced prostate cancer: Long-term clinical results. J Urol 1992;148:342–5
- Li X, Fan W, Zhang L, Zhao M, Huang Z, Li W, et al. CT-guided percutaneous microwave ablation of adrenal malignant carcinoma: Preliminary results. Cancer 2011;117:5182–8
- Carrafiello G, Laganà D, Mangini M, Fontana F, Dionigi G, Boni L, et al. Microwave tumors ablation: Principles, clinical applications and review of preliminary experiences. Int J Surg 2008;6:S65–69
- Kanaoka Y, Hirai K, Ishiko O. Microwave endometrial ablation for an enlarged uterus. Arch Gynecol Obstet 2003;269:30–2
- Tawfeek S, Sharp N. Successful microwave endometrial ablation (MEA) in uterus didelphys. J Obstet Gynaecol 2006;26:582–3
- Gaynor SL, Byrd GD, Diodato MD, Ishii Y, Lee AM, Prasad SM, et al. Microwave ablation for atrial fibrillation: Dose-response curves in the cardioplegia-arrested and beating heart. Ann Thorac Surg 2006;81:72–6
- Tabuse K, Katsumi M. Application of a microwave tissue coagulator to hepatic surgery the hemostatic effects on spontaneous rupture of hepatoma and tumor necrosis. Nippon Geka Hokan 1981;50:571–9
- Laeseke PF, Winter TC III, Davis CL, Stevens KR, Johnson CD, Fronczak FJ, et al. Postbiopsy bleeding in a porcine model: Reduction with radio-frequency ablation – Preliminary results. Radiology 2003;227:493–9
- Rojas-Chapana JA, Correa-Duarte MA, Zhifeng R, Krysztof K, Giersig M. Enhanced introduction of gold nanoparticles into vital Acidothiobacillus ferrooxidans by carbon nanotube-based microwave electroporation. Nano Lett 2004;4:985–8
- Sazgarnia A, Taheri AR, Soudmand S, Parizi AJ, Rajabi O, Darbandi MS. Antiparasitic effects of gold nanoparticles with microwave radiation on promastigotes and amastigotes of Leishmania major. Int J Hyperthermia 2013;29:79–86
- Manthe RL, Foy SP, Krishnamurthy N, Sharma B, Labhasetwar V. Tumor ablation and nanotechnology. Mol Pharm 2010;7:1880–98
- Ahmed M, Moussa M, Goldberg SN. Synergy in cancer treatment between liposomal chemotherapeutics and thermal ablation. Chem Phys Lipids 2012;165:424–37
- Wallen CA, Michaelson SM. Proceedings: Microwave-induced hyperthermia as an adjuvant to cancer therapy. J Microw Power 1976;11:175–6
- Ahmed M, Moussa M, Goldberg SN. Synergy in cancer treatment between liposomal chemotherapeutics and thermal ablation. Chem Phys Lipids 2012;165:424–37
- Ghahremani FH, Sazgarnia A, Bahreyni-Toosi MH, Rajabi O, Aledavood A. Efficacy of microwave hyperthermia and chemotherapy in the presence of gold nanoparticles: An in vitro study on osteosarcoma. Int J Hyperthermia 2011;27:625–36
- Brace CL. Microwave tissue ablation: Biophysics, technology, and applications. Crit Rev Biomed Eng 2010;38:65–78
- Carrafiello G, Mangini M, Fontana F, Di Massa A, Ierardi AM, Cotta E, et al. Complications of microwave and radiofrequency lung ablation: Personal experience and review of the literature. Radiol Med 2012;117:201–13
- Dodd GD III, Dodd NA, Lanctot AC, Glueck DA. Effect of variation of portal venous blood flow on radiofrequency and microwave ablations in a blood-perfused bovine liver model. Radiology 2013;267:129–36
- Durick NA, Laeseke PF, Broderick LS, Lee FT Jr, Sampson LA, Frey TM, et al. Microwave ablation with triaxial antennas tuned for lung: Results in an in vivo porcine model. Radiology 2008;247:80–7
- Brace CL, Laeseke PF, van der Weide DW, Lee FT. Microwave ablation with a triaxial antenna: Results in ex vivo bovine liver. IEEE Trans Microw Theory Tech 2005;53:215–20
- Prakash P. Theoretical modeling for hepatic microwave ablation. Open Biomed Eng J 2010;4:27–38
- Henriques FJ. Studies of thermal injury; the predictability and the significance of thermally induced rate processes leading to irreversible epidermal injury. Arch Pathol (Chic) 1947;43:489–502
- Dewey WC, Diederich CJ, Dewhirst MW. Hyperthermia classic commentary: ‘Arrhenius relationships from the molecule and cell to the clinic’ by William Dewey, Int J Hyperthermia 10:457–483, 1994, 2009;25:21–4
- Moritz AR, Henriques FC. Studies of thermal injury: II. The relative importance of time and surface temperature in the causation of cutaneous burns. Am J Pathol 1947;23:695–720
- He X, Bischof JC. Quantification of temperature and injury response in thermal therapy and cryosurgery. Crit Rev Biomed Eng 2003;31:355–422
- Berjano EJ. Theoretical modeling for radiofrequency ablation: State-of-the-art and challenges for the future. Biomed Eng Online 2006;5:24
- Pennes HH. Analysis of tissue and arterial blood temperatures in the resting human forearm. J Appl Physiol 1948;1:93–122
- Lin J, Hirai S, Chiang C-L, Hsu W-L, Su J-L, Wang Y-J. Computer simulation and experimental studies of SAR distributions of interstitial arrays of sleeved-slot microwave antennas for hyperthermia treatment of brain tumors. IEEE Trans Microwave Theory Tech 2000;48:2191–8
- Pearce JA. Relationship between Arrhenius models of thermal damage and the CEM 43 thermal dose. Proc SPIE 2009;7181:718104–718115. doi:10.1117/12.807999
- Prakash P, Diederich CJ. Considerations for theoretical modelling of thermal ablation with catheter-based ultrasonic sources: Implications for treatment planning, monitoring and control. Int J Hyperthermia 2012;28:69–86
- Sapareto SA, Dewey WC. Thermal dose determination in cancer therapy. Int J Radiat Oncol Biol Phys 1984;10:787–800
- Haemmerich D, Webster J, Mahvi D. Thermal dose versus isotherm as lesion boundary estimator for cardiac and hepatic radio-frequency ablation. IEEE Eng Med Biol Conf 2003;1:134–7
- Mertyna P, Dewhirst MW, Halpern E, Goldberg W, Goldberg SN. Radiofrequency ablation: The effect of distance and baseline temperature on thermal dose required for coagulation. Int J Hyperthermia 2008;24:550–9
- Xu Y, Qian R. Analysis of thermal injury process based on enzyme deactivation mechanisms. J Biomech Eng 1995;117:462–5
- Feng Y, Oden JT, Rylander MN. A Two-state cell damage model under hyperthermic conditions: Theory and in vitro experiments. J Biomech Eng 2008;130:041016
- Prakash P, Deng G, Converse MC, Webster JG, Mahvi DM, Ferris MC. Design optimization of a robust sleeve antenna for hepatic microwave ablation. Phys Med Biol 2008;53:1057–69
- Brace CL. Dual-slot antennas for microwave tissue heating: Parametric analysis and experimental validation. Med Phys 2011;38:4232–40
- Cavagnaro M, Amabile C, Bernardi P, Pisa S, Tosoratti N. A minimally invasive antenna for microwave ablation therapies: Design, performances, and experimental assessment. IEEE Trans Biomed Eng 2011;58:949–59
- Longo I, Gentili G, Cerretelli M, Tosoratti N. A coaxial antenna with miniaturized choke for minimally invasive interstitial heating. IEEE Trans Microwave Theory Tech 2003;50:82–8
- Hurter W, Reinbold F, Lorenz W. A dipole antenna for interstitial microwave hyperthermia. IEEE Trans Microwave Theory Tech 1991;39:1048–54
- Schaller G, Erb J, Engelbrecht R. Field simulation of dipole antennas for interstitial microwave hyperthermia. IEEE Trans Microwave Theory Tech 1996;44:887–95
- Brace CL, van der Weide DW, Lee FT, Laeseke PF, Sampson L. Analysis and experimental validation of a triaxial antenna for microwave tumor ablation. IEEE MTTS Int Microw Symp 2004;3:1437–40
- Lubner MG, Brace CL, Hinshaw JL, Lee FT. Microwave tumor ablation: Mechanism of action, clinical results, and devices. J Vasc Interv Radiol 2010;21:S192–203
- Bakker JF, Paulides MM, Westra AH, Schippers H, Van Rhoon GC. Design and test of a 434 MHz multi-channel amplifier system for targeted hyperthermia applicators. Int J Hyperthermia 2010;26:158–70
- Converse M, Bond EJ, Van Veen BD, Hagness SC. A computational study of ultra-wideband versus narrowband microwave hyperthermia for breast cancer treatment. IEEE Trans Microw Theory Tech 2006;54:2169–80
- Jones RP, Kitteringham NR, Terlizzo M, Hancock C, Dunne D, Fenwick SW, et al. Microwave ablation of ex vivo human liver and colorectal liver metastases with a novel 14.5 GHz generator. Int J Hyperthermia 2012;28:43–54
- Ryan TP, Turner PF, Hamilton B. Interstitial microwave transition from hyperthermia to ablation: Historical perspectives and current trends in thermal therapy. Int J Hyperthermia 2010;26:415–33
- Liu F-Y, Yu X-L, Liang P, Wang Y, Zhou P, Yu J. Comparison of percutaneous 915 MHz microwave ablation and 2450 MHz microwave ablation in large hepatocellular carcinoma. Int J Hyperthermia 2010;26:448–55
- Sun Y, Cheng Z, Dong L, Zhang G, Wang Y, Liang P. Comparison of temperature curve and ablation zone between 915- and 2450-MHz cooled-shaft microwave antenna: Results in ex vivo porcine livers. Eur J Radiol 2012;81:553–7
- Henderson A, James JR. Near-field power transfer effects in small electromagnetic applicators for inducing hyperthermia. IEE Proc Microw Antennas Propag 1985;132:189–97
- Brace CL, Laeseke PF, Sampson LA, Frey TM, van der Weide DW, Lee FT. Microwave ablation with multiple simultaneously powered small-gauge triaxial antennas: Results from an in vivo swine liver model. Radiology 2007;244:151–6
- Laeseke PF, Sampson LA, Lee FTJ, Brace CL. Multiple-antenna microwave ablation: Spatially distributing power improves thermal profiles and reduces invasiveness. J Interv Oncol 2010;2:65–72
- Oshima F, Yamakado K, Nakatsuka A, Takaki H, Makita M, Takeda K. Simultaneous microwave ablation using multiple antennas in explanted bovine livers: Relationship between ablative zone and antenna. Radiat Med 2008;26:408–14
- Wright AS, Lee FTJ, Mahvi DM. Hepatic microwave ablation with multiple antennae results in synergistically larger zones of coagulation necrosis. Ann Surg Oncol 2003;10:275–83
- Yu NC, Lu DSK, Raman SS, Dupuy DE, Simon CJ, Lassman C, et al. Hepatocellular carcinoma: Microwave ablation with multiple straight and loop antenna clusters – Pilot comparison with pathologic findings. Radiology 2006;239:269–75
- Magin RL, Peterson AF. Noninvasive microwave phased arrays for local hyperthermia: A review. Int J Hyperthermia 1989;5:429–50
- Turner PF. Interstitial equal-phased arrays for EM hyperthermia. IEEE Trans Microw Theory Tech 1986;34:572–8
- Furse CM, Iskander MF. Three-dimensional electromagnetic power deposition in tumors using interstitial antenna arrays. IEEE Trans Biomed Eng 1989;36:977–86
- Camart JC, Dubois L, Fabre JJ, Vanloot D, Chive M. 915 MHz microwave interstitial hyperthermia. Part II: Array of phase-monitored antennas. Int J Hyperthermia 1993;9:445–54
- Trembly BS, Wilson AH, Sullivan MJ, Stein AD, Wong TZ, Strohbehn JW. Control of the SAR pattern withing an interstitial microwave array through variation of antenna driving phase. IEEE Trans Microw Theory Tech 1986;34:568–71
- Clibbon KL, McCowen A. Efficient computation of SAR distributions from interstitial microwave antenna arrays. IEEE Trans Microw Theory Tech 1994;42:595–600
- Ryan TP, Trembly BS, Roberts DW, Strohbehn JW, Coughlin CT, Hoopes PJ. Brain hyperthermia: I. Interstitial microwave antenna array techniques – The Dartmouth experience. Int J Radiat Oncol Biol Phys 1994;29:1065–78
- Ryan TP, Mechling JA, Strohbehn JW. Absorbed power deposition for various insertion depths for 915 MHz interstitial dipole antenna arrays: Experiment versus theory. Int J Radiat Oncol Biol Phys 1990;19:377–87
- James BJ, Strohbehn JW, Mechling JA, Trembly BS. The effect of insertion depth on the theoretical SAR patterns of 915 MHz dipole antenna arrays for hyperthermia. Int J Hyperthermia 1989;5:733–47
- Mechling JA, Strohbehn JW, France LJ. A theoretical evaluation of the performance of the Dartmouth IMAAH system to heat cylindrical and ellipsoidal tumour models. Int J Hyperthermia 1991;7:465–83
- Stuchly MA, Kraszewski A, Stuchly SS, Smith AM. Dielectric properties of animal tissues in vivo at radio and microwave frequencies: Comparison between species. Phys Med Biol 1982;27:927–36
- Gabriel S, Lau RW, Gabriel C. The dielectric properties of biological tissues: III. Parametric models for the dielectric spectrum of tissues. Phys Med Biol 1996;41:2271–93
- Schwan HP, Li K. Capacity and conductivity of body tissues at ultrahigh frequencies. Proc IRE 1953;41:1735–40
- Schwan HP, Li K. Measurements of materials with high dielectric constant and conductivity at ultrahigh frequencies. AIEE Trans Comm Electron 1955;74:603–7
- Lazebnik M, Converse MC, Booske JH, Hagness SC. Ultrawideband temperature-dependent dielectric properties of animal liver tissue in the microwave frequency range. Phys Med Biol 2006;51:1941–55
- Yang D, Converse MC, Mahvi DM, Webster JG. Expanding the bioheat equation to include tissue internal water evaporation during heating. IEEE Trans Biomed Eng 2007;54:1382–8
- Schepps JL, Foster KR. The UHF and microwave dielectric properties of normal and tumour tissues: Variation in dielectric properties with tissue water content. Phys Med Biol 1980;25:1149–59
- Bircan C, Barringer S. Determination of protein denaturation of muscle foods using the dielectric properties. J Food Sci 2002;67:202–5
- Wang P, Brace CL. Tissue dielectric measurement using an interstitial dipole antenna. IEEE Trans Biomed Eng 2012;59:115–21
- Chin L, Sherar M. Changes in the dielectric properties of rat prostate ex vivo at 915 MHz during heating. Int J Hyperthermia 2004;20:517–27
- Ji Z, Brace CL. Expanded modeling of temperature-dependent dielectric properties for microwave thermal ablation. Phys Med Biol 2011;56:5249–64
- Lopresto V, Pinto R, Lovisolo GA, Cavagnaro M. Changes in the dielectric properties of ex vivo bovine liver during microwave thermal ablation at 2.45 GHz. Phys Med Biol 2012;57:2309–27
- He X, McGee S, Coad JE, Schmidlin F, Iaizzo PA, Swanlund DJ, et al. Investigation of the thermal and tissue injury behaviour in microwave thermal therapy using a porcine kidney model. Int J Hyperthermia 2004;20:567–93
- Wall MS, Deng XH, Torzilli PA, Doty SB, O’Brien SJ, Warren RF. Thermal modification of collagen. J Shoulder Elbow Surg 1999;8:339–44
- Ai H, Wu S, Gao H, Zhao L, Yang C, Zeng Y. Temperature distribution analysis of tissue water vaporization during microwave ablation: Experiments and simulations. Int J Hyperthermia 2012;28:674–85
- Brace CL, Diaz TA, Hinshaw JL, Lee FT. Tissue contraction caused by radiofrequency and microwave ablation: A laboratory study in liver and lung. J Vasc Interv Radiol 2010;21:1280–6
- Bhardwaj N, Strickland AD, Ahmad F, Atanesyan L, West K, Lloyd DM. A comparative histological evaluation of the ablations produced by microwave, cryotherapy and radiofrequency in the liver. Pathology 2009;41:168–72
- Bhardwaj N, Dormer J, Ahmad F, Strickland AD, Gravante G, West K, et al. Microwave ablation of the liver: A description of lesion evolution over time and an investigation of the heat sink effect. Pathology 2011;43:725–31
- Ahmad F, Gravante G, Bhardwaj N, Strickland A, Basit R, West K, et al. Changes in interleukin-1β and 6 after hepatic microwave tissue ablation compared with radiofrequency, cryotherapy and surgical resections. Am J Surg 2010;200:500–6
- Brace CL. Temperature-dependent dielectric properties of liver tissue measured during thermal ablation: Toward an improved numerical model. Conf Proc IEEE Eng Med Biol Soc; 2008:230–3
- Chen MH, Yang W, Yan K, Dai Y, Wu W, Fan ZH, et al. The role of contrast-enhanced ultrasound in planning treatment protocols for hepatocellular carcinoma before radiofrequency ablation. Clin Radiol 2007;62:752–60
- Cha CH, Lee FT Jr, Gurney JM, Markhardt BK, Warner TF, Kelcz F, et al. CT versus sonography for monitoring radiofrequency ablation in a porcine liver. Am J Roentgenol 2000;175:705–11
- Limanond P, Zimmerman P, Raman SS, Kadell BM, Lu DSK. Interpretation of CT and MRI after radiofrequency ablation of hepatic malignancies. Am J Roentgenol 2003;181:1635–40
- Terraz S, Cernicanu A, Lepetit-Coiffé M, Viallon M, Salomir R, Mentha G, et al. Radiofrequency ablation of small liver malignancies under magnetic resonance guidance: Progress in targeting and preliminary observations with temperature monitoring. Eur Radiol 2010;20:886–97
- Cernicanu A, Lepetit-Coiffe M, Roland J, Becker CD, Terraz S. Validation of fast MR thermometry at 1.5 T with gradient-echo echo planar imaging sequences: Phantom and clinical feasibility studies. NMR Biomed 2008;21:849–58
- Cernicanu A, Lepetit-Coiffe M, Viallon M, Terraz S, Becker CD. New horizons in MR-controlled and monitored radiofrequency ablation of liver tumours. Cancer Imaging 2007;7:160–6
- Kurumi Y, Tani T, Naka S, Shiomi H, Shimizu T, Abe H, et al. MR-guided microwave ablation for malignancies. Int J Clin Oncol 2007;12:85–93
- Morikawa S, Inubushi T, Kurumi Y, Naka S, Sato K, Tani T, et al. MR-guided microwave thermocoagulation therapy of liver tumors: Initial clinical experiences using a 0.5 T open MR system. J Magn Reson Imaging 2002;16:576–83
- Rieke V, Pauly KB. MR thermometry. J Magn Reson Imaging 2008;27:376–90
- Meloni MF, Andreano A, Bovo G, Chiarpotto B, Amabile C, Gelsomino S, et al. Acute portal venous injury after microwave ablation in an in vivo porcine model: A rare possible complication. J Vasc Interv Radiol 2011;22:947–51
- Kröger T, Pätz T, Altrogge I, Schenk A, Lehmann KS, Frericks BB, et al. Fast estimation of the vascular cooling in RFA based on numerical simulation. Open Biomed Eng J 2010;4:16–26
- Kröger T, Pannier S, Kaliske M, Altrogge I, Graf W, Preusser T. Optimal applicator placement in hepatic radiofrequency ablation on the basis of rare data. Comput Methods Biomech Biomed Eng 2010;13:431–40
- Altrogge I, Preusser T, Kröger T, Büskens C, Pereira PL, Schmidt D, et al. Multiscale optimization of the probe placement for radiofrequency ablation. Acad Radiol 2007;14:1310–24
- Haemmerich D, Wright AW, Mahvi DM, Lee FT Jr, Webster JG. Hepatic bipolar radiofrequency ablation creates coagulation zones close to blood vessels: A finite element study. Med Biol Eng Comput 2003;41:317–23
- Consiglieri L, dos Santos I, Haemmerich D. Theoretical analysis of the heat convection coefficient in large vessels and the significance for thermal ablative therapies. Phys Med Biol 2003;48:4125–34
- Schutt DJ, Haemmerich D. Effects of variation in perfusion rates and of perfusion models in computational models of radio frequency tumor ablation. Med Phys 2008;35:3462–70
- Schramm W, Yang D, Haemmerich D. Contribution of direct heating, thermal conduction and perfusion during radiofrequency and microwave ablation. Conf Proc IEEE Eng Med Biol Soc 2006;1:5013–16
- Chiang J, Hynes K, Brace CL. Flow-dependent vascular heat transfer during microwave thermal ablation. IEEE Eng Med Biol Conf; 2012:5582–5
- Kojima Y, Suzuki S, Sakaguchi T, Tsuchiya Y, Okamoto K, Kurachi K, et al. Portal vein thrombosis caused by microwave coagulation therapy for hepatocellular carcinoma: Report of a case. Surg Today 2000;30:844–8
- Meloni MF, Andreano A, Lava M, Lazzaroni S, Okolicsanyi S, Sironi S. Segmental portal vein thrombosis after microwave ablation of liver tumors: Report of two cases. Eur J Radiol Extra 2010;76:e95–e98
- O’Rourke AP, Lazebnik M, Bertram JM, Converse MC, Hagness SC, Webster JG, et al. Dielectric properties of human normal, malignant and cirrhotic liver tissue: In vivo and ex vivo measurements from 0.5 to 20 GHz using a precision open-ended coaxial probe. Phys Med Biol 2007;52:4707–19
- Yoo D-S. The dielectric properties of cancerous tissues in a nude mouse xenograft model. Bioelectromagnetics 2004;25:492–7
- Seitel A, Engel M, Sommer CM, Radeleff BA, Essert-Villard C, Baegert C, et al. Computer-assisted trajectory planning for percutaneous needle insertions. Med Phys 2011;38:3246–59
- Villard C, Baegert C, Schreck P, Soler L, Gangi A. Optimal trajectories computation within regions of interest for hepatic RFA planning. Med Image Comput Comput Assist Interv 2005;8:49–56
- Baegert C, Villard C, Schreck P, Soler L. Multi-criteria trajectory planning for hepatic radiofrequency ablation. Med Image Comput Comput Assist Interv 2007;10:676–84
- Kröger T, Pannier S, Kaliske M, Altrogge I, Graf W, Preusser T. Optimal applicator placement in hepatic radiofrequency ablation on the basis of rare data. Comput Methods Biomech Biomed Eng 2010;13:431–40
- Rieder C, Kröger T, Schumann C, Hahn HK. GPU-based real-time approximation of the ablation zone for radiofrequency ablation. IEEE Trans Vis Comput Graph 2011;17:1812–21