Abstract
Purpose: Transcranial high intensity focused ultrasound (HIFU) therapy guided by magnetic resonance imaging (MRI) is a promising approach for the treatment of brain tumours. Our objective is to validate a dedicated therapy monitoring system for rodents for transcranial HIFU therapy under MRI guidance in an in vivo brain tumour model. Materials and methods: A dedicated MR-compatible ultrasound therapy system and positioning frame was developed. Three MR-compatible prefocused ultrasonic monoelement transducers were designed, operating at 1.5 MHz and 2.5 MHz with different geometries. A full protocol of transcranial HIFU brain therapy under MRI guidance was applied in n = 19 rats without and n = 6 rats with transplanted tumours (RG2). Different heating strategies were tested. After treatment, histological study of the brain was performed in order to confirm thermal lesions. Results: Relying on a larger aperture and a higher frequency, the 2.5 MHz transducer was found to give better results than other ones. This single element transducer optimised the ratio of the temperature elevation at the focus to the one at the skull surface. Using optimised transducer and heating strategies enabled thermal necrosis both in normal and tumour tissues as verified by histology while limiting overheating in the tissues in contact with the skull. Conclusions: In this study, a system for transcranial HIFU therapy guided by MRI was developed and tested in an in vivo rat brain tumour model. The feasibility of this therapy set-up to induce thermal lesions within brain tumours was demonstrated.
Introduction
High intensity focused ultrasound (HIFU) surgery guided by magnetic resonance imaging (MRgFUS) is an innovative approach for the treatment of intracranial tumours. In the near future this therapy could be an interesting alternative to brain surgery and radiotherapy. HIFU induces a temperature elevation at the focus and a defined tissue volume can be thermally destroyed (thermal ablation). For a given frequency and given acoustic parameters, the ablation volume depends on the temperature rise and exposure time (thermal dose). Thermal ablation occurs when the targeted delivery of ultrasound energy causes a rapid temperature rise over 53 °C for more than one second, or equivalently a temperature of 43 °C for 20 to 240 min according to the thermal dose concept [Citation1]. The thermal dose threshold for tissue damage depends on the tissue type [Citation2–4]. If the focal zone is moved in space, in multiple individual locations [Citation5], or along a continuous spiral trajectory [Citation6], a tumour can be treated in complete fashion.
For transcranial brain therapy, the skull bone is a major limitation, making necessary the use of multi-element transducers coupled with adaptive techniques of phase correction [Citation7–15]. Due to skull distortion, target visualisation and exposure quantification cannot be achieved with ultrasound imaging. Magnetic resonance imaging (MRI) has been proposed to plan the treatment to spatially monitor and to control the thermal deposition during treatment and to assess tissue damages after treatment [Citation16–22].
Before treatment, MRI offers a precise tumour detection and target definition. In addition to anatomical sequences, proton resonance frequency shift thermometry techniques can be used for treatment targeting and monitoring [Citation16]. For this purpose, non-coagulative low power sonications are delivered, while small temperature elevations are mapped to identify the location of the focal zone and refine acoustic parameters before delivery of high power sonications for therapeutic doses [Citation17,Citation20,Citation22]. Recent studies have also shown the ability of magnetic resonance acoustic radiation force imaging (MR-ARFI) sequence to detect local displacement of tissues generated by radiation force caused by ultrasound wave front delivered in burst mode [Citation23–26]. It allows verification of the spatial localisation and of the shape of the target zone as well as the quantification of acoustic intensity at the focus [Citation27–30]. This sequence was optimised in a previous work of our team in order to detect displacements of a few micrometres, to quantify the acoustic pressure and also to correct aberrations [Citation27,Citation31].
During treatment, many investigations have shown that MRI thermometry provides a reliable quantification of the temperature rise in real time [Citation32–35]. The control of the energy deposition can be performed according to an open-loop concept [Citation32,Citation33] where the acoustic power is adjusted by the operator based on MR thermometry after each session, or a closed-loop feedback [Citation34,Citation35] where the power is automatically adjusted.
After treatment, different MRI sequences are used to determine the extent of tissue changes: T2-weighted MR images [Citation16,Citation17,Citation18,Citation36,Citation37], contrast enhanced T1-weighted images [Citation18,Citation19,Citation38], diffusion weighted imaging [Citation39] and MR elastography [Citation27] can verify tissue coagulation.
This approach has been tested in a number of clinical studies for the treatment of several tumours, mainly of the prostate [Citation40,Citation41], uterine [Citation42], breast [Citation43,Citation44], bone [Citation45], liver [Citation46], kidney [Citation47] and pancreas [Citation48]. Recently, the brain was also addressed with non-invasive transcanial HIFU: significant pain relieve has been achieved on nine patients with chronic neuropathic pain [Citation49]. For brain tumours, very few teams have tested MRgFUS therapy in phase 1 clinical trials, using a craniectomy [Citation20,Citation50,Citation51]. More recently sub-therapeutic transcranial temperature elevations have been reported on brain gliomas patients [Citation22], in order to validate the targeting and the monitoring but further developments are shown to be necessary before being able to achieve tissue necrosis. Due to little available data and poorly known effects of HIFU on the brain and tumour tissue, it is important to test this technique in in vivo tumour models. For this propose small animals such as rats offer a good and simple model of brain tumours in vivo. Different rodent glioblastoma models including 9 L, C6 and RG2 are characterised in the literature [Citation52–54] and used to test novel therapies. To study the biological effects of focused ultrasound in the brain, different teams have developed apparatus for HIFU therapy in rodents without tumours, to test blood–brain barrier disruption [Citation55,Citation56] and thermal ablation [Citation27]. Other studies have used rodent glioma models injected intracerebrally to test focused ultrasound therapies for blood–brain barrier opening and increased entry of chemotherapy in 9 L (doxorubicin) [Citation57] and C6 (BCNU) [Citation58] tumours. To date, thermal ablation of rodent gliomas has been reported only in subcutaneous injection of 9 L rat glioma [Citation59].
In this work, we propose a protocol of thermal ablation therapy in a rat brain tumour model, in order to test this novel therapy on brain tumours in vivo. MR-ARFI was used to verify the localisation before treatment. MR thermometry sequences were used during the treatment to follow the temperature changes around the focus and around the skull. T2, T1 and contrast enhanced T1-weighted MR images were used to localise tumours, to plan treatment and to evaluate tissue damages.
Materials and methods
Animals and brain tumour model in rat
All experiments were carried out according to the European Community guiding principles in the care and use of animals (86/609/CEE). A total of 30 rats Ficher-344, male, 6 weeks and 180 g (Charles River, St Germain sur l'Arbresle, France) were used for these experiments. The full HIFU protocol was tested in 19 rats without tumours and in six rats with transplanted tumours. Two other rats were used for the measurement of the cooling of the scalp. Nine rats were intracerebrally transplanted with RG2 glioma, three rats for the tumour growth follow-up and six rats for HIFU treatments.
RG2 glioma cells were cultivated in vitro in Dulbecco’s modified Eagle’s medium (DMEM) supplemented with 10% fetal bovine serum (FBS) and 1% penicillin-streptomycin. After anaesthesia with 600 μL/180 g mixture (ketamine 4 mL, xylazine 2 mL, NaCl 9 mL), animals were placed in a stereotactic apparatus. Through a small burr Ø = 1 mm, 1 × 104 cells in a volume of 5 μJ phosphate buffered saline (PBS), pH 7.2 were implanted intracerebrally into the right striatum under stereotactic conditions, using a 10 μL Hamilton syringe with a 33 gauge needle. The injection technique and the deep injection site were adapted in a region where the ultrasound beams can focused, coordinates: 2 mm forward from the bregma, 5 mm right from the midline, and ventral 6 mm from the dura with an angle of about 60° relative to the midline. A large skin incision in an arch shape was chosen in order to avoid scars on the ultrasound pathway. The number of injected cells was optimised at 1.5 × 104 to ensure a good graft and interesting kinetics.
Tumour kinetics was followed to identify the growth time before the tumour reached an acceptable size for HIFU treatment. The tumour growth was followed with T2-weighted, T1 and contrast enhanced T1-weighted MR images every 2 days until their death for the preliminary tests and until the volume reached 50 ± 5 mm3, the volume chosen for treatment day, for the treated animals.
MR-compatible HIFU therapy system and positioning frame
A dedicated plastic positioning system was designed and constructed to position both the animals and the ultrasound transducer inside the MRI machine. This mechanical system was designed to manually move the probe in all three axes, with a precision less than 0.5 mm (). More details on the ultrasonic treatment unit can be found in a previous work [Citation27].
Figure 1. (A) MR-compatible positioning system; the transducer is at the centre of the image and a manual rotation and head--foot translation of the transducer is allowed by a dedicated chariot and a junction. Tooth and ear bars provide rigid stereotaxy under the transducer. (B) Full MRgFUS therapy set-up.
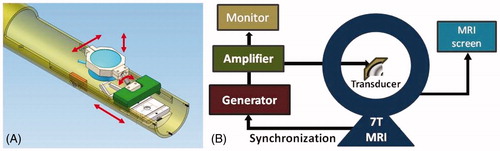
The radiofrequency driving unit consisted of an arbitrary function generator (Tektronix AFG 3021B) and a power amplifier (Aquitek 2100L). The output of the circuit was monitored using an oscilloscope (Tektronix DPO 3034) equipped with a voltage and a current probe giving real-time calculations of transmitted power. The reflected power was also measured by the amplifier itself (). The impedance of the transducer was measured and kept constant during all the experiments.
Three MR-compatible prefocused ultrasonic monoelement transducers (1) focal length F = 25 mm, focal length/diameter (F/D) = 1; 2) and 3) focal length F = 20 mm, F/D = 0.8) (Imasonic, Aix-en-Provence, France) were designed and used in this work, adapted to the anatomy of the rat skull, operating respectively at 1.5 MHz, 1.5 MHz and 2.5 MHz as summarised in .
Table I. Characteristics of the different transducers studied.
shows T2-weighted MR images of the T1 (left) and T2 (right) transducers on top of the rat head with the position and schematic representation of the extent of the corresponding focal spots.
Figure 2. T2 weighted images of the transducers on top of the rat head with the position and predicted extent of the corresponding focal spots. (A) Single element F/D = 1. (B) Single element F/D = 0.8.
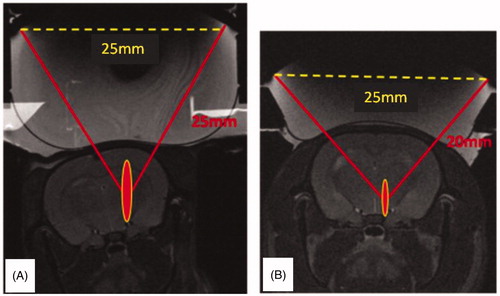
Pressure measurements were performed at the focus in a water tank, through the skull bone, or not, with a calibrated hydrophone (HGL-0200 with an electrode aperture of 200 µm, preamplifier AH-2010, Onda, Sunnyvale, CA). The good coupling of the transducer with the scalp was realised with the help of a latex membrane integrated to the transducers and filled with degassed water, and ultrasound gel (Drexco Medical, Crosne, France).
MR imaging and HIFU protocol (MRgFUS)
MR imaging and treatment were performed with a 7T small animal MRI scanner (Bruker Biospin, Ettlingen, Germany) (). Animals were anaesthetised with isoflurane 2–3%, placed in the positioning frame in prone position with their head fixed, and positioned into the MRI scanner. The respiratory rate and body temperature were monitored during the whole procedure using a commercial system (SA Instruments, Stony Brook, NY) composed of a pressure sensor and a rectal thermocouple.
For the tumour growth follow up, T2-weighted (echo time TE = 12 ms, repetition time TR = 4200 ms, acquisition time 202 s for each orientation) and contrast enhanced T1-weighted (TE = 8 ms, TR = 2500 ms, acquisition time 300 s for each orientation) MR images were acquired in three orientations and used to localise the tumour in the brain, and to measure its dimensions. For both contrasts, a rapid acquisition with refocused echoes (RARE) sequence was used with the following parameters: 32 × 32 mm field of view (matrix size 256 × 256, zero-fill phase acceleration factor of 1.34, RARE factor 8), sufficient number of slices to cover the whole brain in each orientation (slice thickness 1 mm). For contrast enhanced images, gadolinium chelate (Dotarem 0.5 mmol/mL, Guerbet, Aulnay Sous Bois, France) was injected intraperitoneally 0.3 mL/180 g bolus, and MRI acquisitions were done 10–20 min later. Specific contrast enhancement was visible in the tumour for 50–60 min following gadolinium injection. Tumour volume was measured using the formula V = (D × d2)/2 (D = the large diameter, d = the small diameter).
Once the tumour has reached the desired size, transcranial HIFU therapy was performed. The rat heads were shaved with depilatory cream before installation. The MR-compatible ultrasound transducer was positioned on top of their heads using acoustic coupling gel before insertion in the MRI bore hole. The anatomical images described above were acquired to localise the transducer position, to verify that the geometrical position of the focal spot was at the desired target in the brain or in the tumour, and to ensure that there was a good coupling of the membrane with the scalp with no air bubbles trapped in between.
After anatomical imaging, the image of the acoustic radiation force (MR-ARFI) was acquired to verify the spatial localisation of the target zone and the quality of the focusing before treatment. MR-ARFI consisted of a standard multi-slice spin echo sequence (TE = 40 ms, TR = 1600 ms) with two additional bipolar sinusoidal gradients in the slice selection direction, equally distant to the 180 ° radiofrequency pulse synchronised with ultrasound bursts of 3 ms at relatively high acoustic intensity (1–2 MPa estimated intracranial peak negative pressure). The sequence lasted 3 min 25 s, and had a sensitivity to motion of around 1 µm with an acoustic duty cycle of less than 5%. The imaging resolution was 0.7 mm in place resolution, 5 slices, and 2 mm slice thickness.
MR thermometry with high resolution was then used to measure and map the temperature rise. Temperature rise was acquired in real time (continuously) during treatment, measured and mapped immediately after each session. The acoustic power was increased to different levels (4.56–23.22 acoustic W) to reach a peak temperature higher than 50 °C. The duration of the treatments was 2–100 s and the number of HIFU sonications was 1–6 at the same location. The thermal doses were calculated offline using Matlab 7.04 software (Mathworks, Natick, MA, USA), in order to test different heating strategies. The initial temperature of the brain was set to the measured rectal temperature of the animal. First, a unique imaged slice was positioned in the focal plane, orthogonal to the ultrasound beam, identified with MR-ARFI and according to geometrical position of the transducer. For the experiments with transducers T2 and T3 (1.5 and 2.5 MHz F/D = 0.8), a second imaging plane (parallel with the previous) was acquired across the skull to monitor cortex and skin overheats which appeared to be critical. The effect of the circulation of cold water (10 °C) in the water balloon on top of the rats’ heads right before the HIFU session was also evaluated. MR thermometry sequence was prepared from a standard fast low angle shot (FLASH) sequence (TE = 3.5 ms, TR = 15 ms and flip angle 30 °). It generated one temperature map every 1 s with 64 × 64 voxels. The spatial resolution was 0.7 × 0.7 × 3 mm3 giving a temperature precision of 2 °C.
After the treatment, T2 and T1-weighted MR images were performed immediately, 30 and 60 min later, to verify and follow tissue damages in brain and tumour tissue. Contrast enhanced T1-weighted images were used in three cases for the same purpose. The anatomical images were compared to MR thermometry mappings and MR-ARFI images to verify the good localisation of target zone in the brain or within the tumour.
Histological examination
After MRI examinations, the animals were euthanised and the brains were immediately removed and fixated in 4% paraformaldehyde for 7 days. The brains were embedded in paraffin and sectioned using a microtome. Sections of the whole brain (5 μm, 19 without and 6 with tumour), were stained with haematoxylin and eosin for basic histological analysis.
Results
Brain tumour model
Overall survival time of animals without treatment was 16–19 days and mean survival was 17.6 days. The target tumour volume chosen for treatment was 50 ± 5 mm3. According to our MRI measurements, this tumour size was reached 12–13 days after grafts of RG2 glioma ().
HIFU therapy (treatments)
Heating strategies at 1.5 MHz (F/D = 1)
Initially, a series of acoustic pressures ranging from 1.19 to 3.65 MPa (peak negative pressure estimated from the calibration at the focus) were delivered during 6 to 100 s, in one or two treatment sessions, with the first transducer operating at 1.5 MHz (F/D = 1), in 10 rats without tumour, in order to test different thermal doses. summarises the experimental conditions that were tested on a total of 10 rats. Three heating strategies were principally tested: (1) low pressure 1.19–1.82 MPa during 12–40 s, (2) middle pressure 1.69–2.49 MPa during 100 s and (3) high pressure 2.66–3.65 MPa during 6–10 s (see ). The average temperature elevation at the focus was 14 °C, estimated from MR thermometry. The average of the maximum temperature at the focus was 47 °C, which was sufficient to induce radiological changes detectable on T2-weighted images corresponding to necrosis found at histology in only three cases. Limited oedema was also found at histology in the focal region for two additional cases. In all cases, skin burns (macroscopic post-treatment observation) and oedema in the cortex (post-treatment T2-weighted images) were observed, indicating that heating was more pronounced at the skull surface than at the focus. Unfortunately, no monitoring of the temperature rise at the skull surface was done at that time of our study. This monitoring was added to the protocol for the following experiments.
Table II. In vivo experiments at 1.5 MHz (temperature rise at the focus estimated from MR thermometry data).
Based on this first study, it was thus not possible to use this transducer for reproducible transcranial tumour ablations.
Measurement of the cooling of the scalp
As shown by the skin burns and cortex oedema observed previously, it is difficult to find a heating scenario allowing more energy to be deposited at the focus than in the skull using a F/D = 1, 1.5 MHz transducer.
Simulations showed that an efficient way to avoid overheating of the skull and cortex was to cool the scalp with circulating cold water around 10 °C during HIFU treatment. We have tested experimentally this technique in two rats without treatment and in each case MR thermometry is realised with cooling. Temperature drops were measured in two horizontal planes (at the focus and in the cortex) and a difference due to cooling was estimated to be −4 °C to −5 °C between the focal and the cortex planes (). After these tests, the cooling was used in all rats 3 s before the start and during all HIFU treatment.
Evaluation of the transducer T2 (1.5 MHz, F/D = 0.8)
A series of sonications was performed in the healthy brains of three rats () with transducer T2 (1.5 MHz, F/D = 0.8). The acoustic power varied from 12.40 to 23.22 acoustic W, the duration from 5–10 s and the number of sessions from 1 to 3. With these changes the temperature elevation in the focal plane was 15 °C and in the cortex plane 14 °C. Despite the improvement of the ratio in the focal and cortex plane, the temperature rise in cortex remained high, with 1 lesion in both the focal and cortex plane in 1 case.
Table III. In vivo experiments at 1.5 MHz F/D = 0.8 (temperature rise at the focus estimated from MR thermometry data).
Improvement of heating strategies at 2.5 MHz
With the transducer T3 (2.5 MHz, F/D = 0.8), different acoustic powers, sonication durations and the number of sessions were also tested to improve heating strategies and to avoid the overheating of the skull. gives four examples of thermometry measurements in the cortex and at the focus for the following strategies: (A) a single sonication at 4.33 MPa for 20 s, (B) four sonications at 7.58 MPa for 2 s with a break of 2 s between each sonication, (C) two sonications at 7.58 MPa for 5 s with a break of 5 s between each sonication, (D) a single sonication at 7.58 MPa for 7 s.
Figure 5. Different heating strategies with the 2.5 MHz transducer. (A) Single sonication at 4.33 MPa for 20 s. (B) Four sonications at 7.58 MPa, 2 s on 2 s off. (C) Two sonications at 7.58 MPa, 5 s on 5 s off. (D) Single sonication at 7.58 MPa for 7 s.
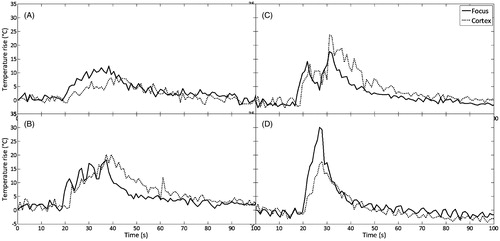
In all cases active cooling was applied at 10 °C during sonications. The best strategy with less heating of the skull and sufficient heating at the focus was using high acoustic pressures 7.58 MPa (acoustic power 15.20 W) and short duration of sonications (7 s), with 10 s active cooling at 10 °C which was started 3 s before HIFU (). In this case, temperature elevation in the focal plane was 31 °C and in the cortex plane 17 °C. summarises all the experimental conditions that were tested at 2.5 MHz on a total of 12 rats, six rats without tumours (1–6) and six rats with RG2 tumours (7–12). For all rats treated with the 2.5 MHz transducer, the average temperature elevation, in the focal plane was 17 °C in rats without tumours and 20 °C in rats with tumour. In the cortex plane the average temperature rise was 14 °C and 15 °C respectively in rats without and with tumour. The average of the maximum temperature at the focus was 50 °C in rats without tumours and 52 °C in rats with tumour; and at cortex was 47 °C in rats without tumours and 46 °C in rats with tumour. Temperature rise at the focus was sufficient to induce necrosis (verified at histology) in healthy brain in four cases and within the tumour in three cases. Temperature rise at cortex was high in three cases, causing three mild cortex lesions. No skin burns were observed after treatments (see ).
Table IV. In vivo experiments at 2.5 MHz F/D = 0.8 (temperature rise at the focus is estimated from MR thermometry data); 1–6 rats without tumours, 7–12 rats with tumours.
The results for all the proposed transducers are summarised in . The 1.5 MHz transducer (F/D = 1) appeared to be sufficient to induce necrosis at the focus with many difficulties and complications, because of the overheating of the skull. With the 1.5 MHz transducer (F/D = 0.8) this overheating was lower, but still significant. Using the 2.5 MHz transducer (F/D = 0.8), the overheating of the skull was limited and the ratio of the temperature elevation at the focus and on the skull surface was optimised. shows that the changes of transducers, although not completely cancelling skull overheating, enabled the number of unwanted damages outside the focal zone to be greatly reduced and increased the capabilities of inducing a thermal necrosis at the focus.
Table V. Summary of in vivo experiments.
MR Imaging and assessment of tissue changes
Three MRI sequences were successively applied to target, monitor and assess HIFU treatment in vivo on brain tumours. The focal zone was positioned inside the tumour on the basis of MR-ARFI images. T2-weighted images were a good tool to detect tumour >1 mm diameter, oedema, thermal necrosis in the brain tissue and haemorrhages in the treated zone in the tumours. T2-weighted images appeared to be insufficient to visualise thermal necrosis in tumours without haemorrhage. Contrast enhanced T1-weighted imaging was a very good tool to detect small tumours (≈1 mm diameter), but not superior to T2-weighted images to detect necrosis in brain and tumour. The temperature rise was measured and mapped with a MR thermometry sequence for each session with a ≈1–2 °C precision, and corresponding thermal doses were calculated. Maximum temperatures at the focus and at the cortex are summarised in .
Thermal lesions at the focus were found in T2-weighted images as iso-signals surrounded by a ring-shaped hyper signal in the healthy brain of four rats without tumours (), and as hypo T2 signals in three cases in tumours ().
Figure 6. Image correlations. (A) Thermal lesion in the healthy brain. (B) Thermal lesion in the brain tumour. The red arrows indicate the region of acoustic energy deposition. The black arrows indicate the tumour location. (1) T2-weighted MRI, (2) MR-ARFI before treatment, (3) MR thermometry map during treatment, (4) T2-weighted MRI after treatment, (5) macroscopic section of whole brain H&E.
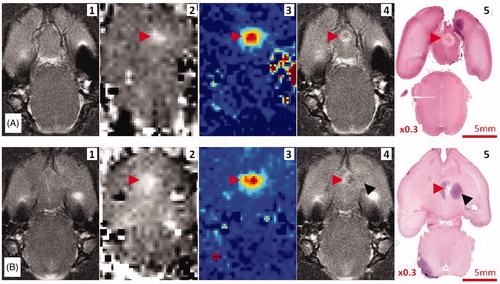
Cortex lesions close to the skull appeared as a T2 hypersignal corresponding with oedema and necrosis in histological examination; severe lesions in four cases and mild lesions in six cases (in all 10 rats treated with the 1.5 MHz transducer). In the cases treated with the 2.5 MHz transducer, three mild cortex lesions were found by MRI and histological analysis (among all 12 treated rats).
Histological analysis
Thermal lesions were induced in cerebral tissue in eight cases and in brain tumours in three cases. These lesions were presented with a sharp demarcated area. Histological analysis demonstrated two different zones of damages: a central zone with coagulative necrosis and peripheral zone dominated by oedema ().
Figure 7. (A) Thermal lesion in healthy brain (rat sacrificed 48 h after HIFU); (B) Thermal lesion in brain tumour (rat sacrificed 4 h after HIFU). (1) centre, (2) peripheral, (3) healthy brain, (4) untreated tumour.
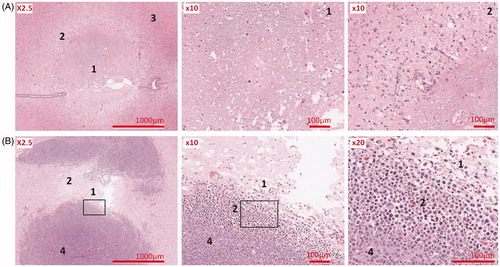
In three cases, treated with the 2.5 MHz transducer, thermal lesions were verified in cortex surface near to the skull ().
Correlations
The acoustic power did not correlate with the temperature elevation as it was depending on the localisation of the focal spot with respect to the different brain interfaces. Temperature elevations and thermal dose maps correlated well with the thermal lesions found in histological analyses.
Good correlation between MR-ARFI and MR thermometry mapping, anatomical MR images and histological findings is shown in .
Discussion
We have shown in a previous study the use of a 1.5-MHz device dedicated set-up for HIFU therapy under MRI guidance in small animals as a proof-of-concept [Citation27]. The first objective of this work was to pursue the development and to improve the stereotactic device for transcranial HIFU therapy in rodent brains under MRI guidance. The second objective was to evaluate the ability of this therapeutic system to properly target the tumour in vivo in the rat brain glioma model. For this purpose, an in vivo brain tumour model was implanted and its kinetic has been established. Implanted RG2 glioma developed rapidly and in all cases with good reproducibility. The growth fashion and the radiological and histological characteristics of these gliomas resemble more to the ones of cerebral metastasis in humans.
Small animals have the advantage of developing brain tumours in a fast and reproducible fashion, and they offer conditions to evaluate the biological effects of HIFU therapy in both brain tissue and tumours. As mentioned above, other teams have tested thermal ablation of rodent gliomas in subcutaneous injection [Citation59]. But the in vivo tumour model into the brain and skull, which is a closed box, and the transcranial treatment with high intensity focused ultrasound offer similar conditions to brain tumours in humans. Thus, this model enables the investigation of the potential interaction and reverberation of ultrasound into the cranial box.
The positioning system was improved and adapted to move the probe in all three axis directions, with a precision of 0.2–0.5 mm. After many tests and thermal simulations, a novel transducer operating at 2.5 MHz was designed and optimised to the head geometry of rats. A full protocol of transcranial HIFU therapy has been achieved under MRI guidance in each step with high resolution and in real time. For each sonication, different parameters such as the acoustic power, pulse duration and number of sonications were tested in order to determine the appropriate strategy. The best strategy for this model was shown to be high acoustic pressures (7.58 MPa) with one short sonication (7 s) at 2.5 MHz. MR temperature elevation measurement showed that heating at the focal plane was higher than in the cortex plane. Additional cooling (10 s cooling time, starting 3 s before sonication) increased the temperature difference between focus and cortex by 3–4 °C. Thermal lesions were generated, demonstrating that the system can induce thermal lesions transcranially on brain tumours, which was challenging, given the small size of the rat brain, and thus the relatively high acoustic frequency employed. A good correlation between MR-ARFI, MR thermometry, anatomical MR images and histological finding was achieved (). Complications such as cortex oedema and skin burns were found mainly with the 1.5 MHz transducers, due to the dimensions of the focal spot (1.5 × 1.5 × 8 mm) that was too long relative to the dimensions of the rat brain and to an insufficient antenna gain: the cross-section of the ultrasonic beam at the focus was not small enough compared to the cross-section of the beam at the skull surface. Such a ratio of both surfaces is inversely proportional to the energy deposition at each location. As shown by the skin burns and cortex oedema, it was difficult to find a heating scenario allowing more energy to be deposited at the focus than in the skull using the F/D = 1, 1.5 MHz transducer. This has been corrected by using a transducer working at a higher 2.5 MHz frequency (thus decreasing the cross-section of the focus) and with a larger diameter (thus decreasing the cross-section of the focus and increasing the cross section at the surface of the skull).
During this work we have identified two main difficulties of transcranial HIFU therapy in small animals: skull bone and cortex overheating, and variability of energy deposition at the focus. As discussed before, the skull overheating is mainly linked to the antenna gain of the transducer and a high frequency transducer with a large aperture has to be favoured. Nevertheless, the frequency range has an upper limit when using a single element transducer. A single element transducer does not allow the performing of aberration correction, and the defocusing effect of the rat skull needs to remain low. This is typically the case if the thickness of the skull is smaller than the ultrasonic wavelength. With a mean 0.5-mm thick rat skull, the maximum acceptable frequency to keep this hypothesis valid should be around 2.5 MHz. We thus chose this frequency as a compromise and then we tested different heating strategies. We verified that for longer exposure time stronger skull heating was induced. This makes sense as in that case thermal conduction and perfusion give a smoother temperature rise at the focal zone due to sharp temperature gradients, whereas the skull accumulates more energy (). Similar results were obtained with strategies using several sonications in a row with the same total exposure time (). The best strategy was short and fast treatment duration of 2–7 s with high acoustic pressure 6.93–7.58 MPa (13.68–15.22 acoustic W) and cooling during the sessions of ≈10 s ().
The variability of the energy deposition at the focus mainly depends on the skull geometry and the incident angle of the ultrasound beam on the bone surface. Recently O’Reilly and colleagues [Citation60] mapped the acoustic field with ultrasound propagating at different frequencies through different locations of the rat skull. No dependency of the skull attenuation with body mass was found in our range of frequencies. Such results support our assumption that skull attenuation was set constant among animals in this paper. We always used the attenuation values measured during calibration in a water tank for each transducer through ex vivo skulls of adult rats. Our attenuation coefficient is coherent with theirs. However, O’Reilly and colleagues [Citation60] also show that for frequencies higher than 2.5 MHz the transmission factor depends on the position and incidence of the skull and that the field distortions become significant. These phenomena explain the variability of the heat deposition obtained in this paper for identical emitted energy (as measured by the acoustic power). MR-ARFI signal appeared to be better related to the obtained heating than measured acoustic power. When an abnormally small tissue displacement at the focus was measured (corresponding to poor energy deposition) the transducer was removed and its coupling was double-checked, and the alignment of the transducer was improved; the direction of the beam path was set as perpendicular as possible to the skull surface. To help in the repositioning process, we did our best to implant the tumour within an accurate and reproducible location. Another factor that influences the energy deposition at the focus is the presence of potential incision scars induced during tumour injection. In order to avoid this, an arch shaped incision was performed out of the beam path.
By applying this complete protocol, the MR-guided ultrasonic therapy system was able to induce a treatment spot within the brain tumour with a satisfactory success rate of 50% (n = 3 among 6) of the targeted treatments. Manual positioning of the transducer appeared to be a limiting factor in the targeting accuracy. Adjustment required pulling out the whole set-up from the magnet to change the location of the focal point based on the T2 and ARFI images. We believe that the use of step motors would increase the success rate. A prototype system is under development with MR compatible 100 µm step motors to help repositioning with a higher precision.
The animal model and the full set-up can be used for small animals to investigate HIFU therapy for thermal ablation of brain tumours. In the future, such a model could be used to investigate the biological effects of ultrasound in both brain tissue and brain tumour for thermal ablation, but also for blood–brain barrier disruption in tumours, and targeted drug and gene delivery by hyperthermia.
Conclusion
In this study, a system for transcranial HIFU therapy guided by MRI was developed and evaluated in an in vivo rat brain tumour model. The feasibility of this therapy set-up to induce thermal lesions within brain tumours was demonstrated. Magnetic resonance imaging was used for controlling HIFU brain therapy at all steps, in order to target, monitor and assess treatment. MR imagines of the acoustic radiation force (MR-ARFI) were implemented to verify the spatial localisation of the target zone and the quality of the acoustic pressure in this zone. Three MR-compatible ultrasonic monoelement transducers were designed, operating at 1.5 MHz and 2.5 MHz with different geometries, and different heating strategies were tested. Relying on a larger aperture and a higher frequency, the 2.5 MHz transducer compared with the other two (1.5 MHz F/D = 1 and 1.5 MHz F/D = 0.8), was found to give the best results. This single element transducer optimised the ratio of the temperature elevation at the focus to the one on the skull surface. Using optimised transducer and heating strategies enabled to obtain thermal necrosis both in normal and tumour tissues as verified by histology while limiting overheating in the tissues in contact with the skull.
The next step will be to characterise the tissue changes induced by HIFU therapy in the rat brain tumours (follow up of the biological effects) and to prove the safety and efficacy of this treatment for brain tumours.
Declaration of interest
This work was supported by the Laboratory of Excellence within the French Program ‘Investments for the Future’ (LABEX-WIFI) under reference ANR-10-IDEX-0001-02 PSL. The authors acknowledge the Agence Nationale pour la Recherche and the Association pour la Recherche sur le Cancer for financial support. The authors alone are responsible for the content and writing of the paper.
Acknowledgements
We thank Abdel Souilah for his help on mechanical design and manufacturing.
References
- Sapareto SA, Dewey WC. Thermal dose determination in cancer therapy. Int J Radiat Oncol Biol Phys 1984;10:787–800
- Dewey WC. Arrhenius relationships from the molecule and cell to the clinic. Int J Hyperthermia 1994;10:457–83
- Diederich CJ. Thermal ablation and high-temperature thermal therapy: Overview of technology and clinical implementation. Int J Hyperthermia 2005;21:745–53
- Ter Haar G. Therapeutic applications of ultrasound. Prog Biophys Mol Biol 2007;93:111–29
- Kohler MO, Mougenot C, Quesson B, Enholm J, Le Bail B, Laurent C, et al. Volumetric HIFU ablation under 3D guidance of rapid MRI thermometry. Med Phys 2009;36:3521–35
- Salomir R, Palussiere J, Vimeux FC, de Zwart JA, Quesson B, Gauchet M, et al. Local hyperthermia with MR-guided focused ultrasound: Spiral trajectory of the focal point optimized for temperature uniformity in the target region. J Magn Reson Imaging 2000;12:571–83
- Thomas JL, Fink M. Ultrasonic beam focusing through tissue inhomogeneities with a time reversal mirror: Application to transskull therapy. IEEE Trans UFFC 1996;43:1122–9
- Tanter M, Thomas JL, Fink M. Focusing and steering through absorbing and aberrating layers: Application to ultrasonic propagation through the skull. J Acoust Soc Am 1998;103:2403–10
- Hynynen K, Jolesz FA. Demonstration of potential noninvasive ultrasound brain therapy through intact skull. Ultrasound Med Biol 1998;24:275–83
- Clement GT, Sun J, Giesecke T, Hynynen K. A hemisphere array for noninvasive ultrasound brain therapy and surgery. Phys Med Biol 2000;45:3707–19
- Clement GT, Hynynen K. A non-invasive method for focusing ultrasound through the human skull. Phys Med Biol 2002;47:1219–36
- Aubry JF, Tanter M, Thomas JL, Pernot M, Fink M. Experimental demonstration of non invasive transskull adaptive focusing based on prior CT scans. J Acoust Soc Am 2003;113:84–94
- Pernot M, Aubry JF, Tanter M, Thomas JL, Fink M. High power transcranial beam steering for ultrasonic brain therapy. Phys Med Biol 2003;48:2577–89
- Tanter M, Pernot M, Aubry JF, Montaldo G, Marquet F, Fink M. Compensating for bone interfaces and respiratory motion in high intensity focused ultrasound. Int J Hyperthermia 2007;23:141–51
- Marsac L, Chauvet D, Larrat B, Pernot M, Robert B, Fink M, et al. MR-guided adaptive focusing of therapeutic ultrasound beams in the human head. Med Phys 2012;39:1141--9
- De Senneville BD, Quesson B, Moonen CT. Magnetic resonance temperature imaging. Int J Hyperthermia 2005;21:515–31
- Hynynen K, Vykhodtseva N, Chung A, Sorrentino V, Colucci V, Jolesz FA. Thermal effects of focused ultrasound on the brain: determination with MR Imaging. Radiology 1997;204:247–53
- Vykhodtseva N, Sorrentino V, Jolesz FA, Bronson RT, Hynynen K. MRI detection of the thermal effects of focused ultrasound on the brain. Ultrasound Med Biol 2000;26:871–80
- McDannold NJ, Tempany CM, Fennessy FM, So MJ, Rybicki FJ, Stewart EA, et al. Uterine leiomyomas: MR imaging-based thermometry and thermal dosimetry during focused ultrasound thermal ablation. Radiology 2006;240:263–72
- Ram Z, Cohen ZR, Harnof S, Tal S, Faibel M, Nass D, et al. Magnetic resonance imaging-guided, high-intensity focused ultrasound for brain tumor therapy. Neurosurgery 2006;59:949–55
- Cohen ZR, Zaubermann J, Harnof S, Mardor Y, Nass D, Zadicario E, et al. Magnetic Resonance Imaging-Guided Focused Ultrasound for thermal ablation in the brain: A feasibility study in a swine model. Neurosurgery 2007;60:593–600
- McDannold NJ, Clement G, Black PM, Jolesz FA, Hynynen K. Transcranial MRI-guided focused ultrasound surgery of brain tumors: Initial findings in 3 patients. Neurosurgery 2010;66:323–32
- Sarvazyan AP, Rudenko OV, Swanson SD, Fowlkes JB, Emelianov SY. Shear wave elasticity imaging: A new ultrasonic technology of medical diagnostics. Ultrasound Med Biol 1998;24:1419–35
- Sinkus R, Tanter M, Bercoff J, Siegmann K, Pernot M, Athanasiou A, et al. Potential of MRI and ultrasound radiation force in elastography: Applications to diagnosis and therapy. Proc IEEE 2008;96:490–9
- McDannold NJ, Maier SE. Magnetic resonance acoustic radiation force imaging. Med Phys 2008;35:3748–58
- Souchon R, Salomir R, Beuf O, Milot L, Grenier D, Lyonnet D, et al. Transient MR elastography (t-MRE) using ultrasound radiation force: Theory, safety, and initial experiments in vitro. Magn Reson Med 2008;60:871–81
- Larrat B, Pernot M, Aubry JF, Dervishi E, Sinkus R, Seilhean D, et al. MR-guided transcranial brain HIFU in small animal models. Phys Med Biol 2010;55:365–88
- Chen J, Watkins R, Pauly KB. Optimization of encoding gradients for MR-ARFI. Magn Reson Med 2010;63:1050–8
- Hertzberg Y, Volovick A, Zur Y, Medan Y, Vitek S, Navon G. Ultrasound focusing using magnetic resonance acoustic radiation force imaging: Application to ultrasound transcranial therapy. Med Phys 2010;37:2934–42
- Auboiroux V, Viallon M, Roland J, Hyacinthe JN, Petruska L, Morel DR, et al. ARFI-prepared MRgHIFU in liver: Simultaneous mapping of ARFI-displacement and temperature elevation, using a fast GRE-EPI sequence. Magn Reson Med 2012;68:932–46
- Larrat B, Pernot M, Montaldo G, Fink M, Tanter M. MR-guided adaptive focusing of ultrasound. IEEE Trans Ultrason Ferroelectr Freq Control 2010;57:1734–7
- Quesson B, de Zwart JA, Moonen CT. Magnetic resonance temperature imaging for guidance of thermotherapy. J Magn Reson Imag 2000;12:525–33
- McDannold NJ, King RL, Jolesz FA, Hynynen K. Usefulness of MR imaging-derived thermometry and dosimetry in determining the threshold for tissue damage induced by thermal surgery in rabbits. Radiology 2000;216:517–23
- Quesson B, Vimeux F, Salomir R, de Zwart JA, Moonen CT. Automatic control of hyperthermic therapy based on real-time analysis of MR temperature maps. Magn Reson Med 2002;47:1065–72
- Vanne A, Hynynen K. MRI feedback temperature control for focused ultrasound surgery. Phys Med Biol 2003;48:31–43
- Hynynen K, Darkazanli A, Unger E, Schenck JF. MRI-guided noninvasive ultrasound surgery. Med Phys 1993;20:107–15
- Chen L, Bouley D, Yuh E, D’Arceuil H, Butts K. Study of focused ultrasound tissue damage using MRI and histology. J Magn Reson Imaging 1999;10:146–53
- Hynynen K, Darkazanli A, Damianou C, Unger E, Schenck JF. The usefulness of contrast agent and GRASS imaging sequence for MRI guided noninvasive ultrasound surgery. Invest Radiol 1994;29:897–903
- Jacobs MA, Ouwerkerk R, Kamel I, Bottomley PA, Bluemke DA, Kim HS. Proton diffusion-weighted imaging and sodium (23Na) MRI of uterine leiomyomata after MR-guided high-intensity focused ultrasound: A preliminary study. J Magn Reson Imag 2009;29:649–56
- Uchida T, Ohkusa H, Yamashita H, Shoji S, Nagata Y, Hyodo T, Satoh T. Five years experience of transrectal high-intensity focused ultrasound using the Sonablate device in the treatment of localized prostate cancer. Int J Urol 2006;13:228–33
- Poissonnier L, Chapelon JY, Rouviere O, Curiel L, Bouvier R, Martin X, et al. Control of prostate cancer by transrectal HIFU in 227 patients. Eur Urol 2007;51:381–7
- Fennessy FM, Tempany CM, McDannold NJ, So MJ, Hesley G, Gostout B, et al. Uterine leiomyomas: MR imaging guided focused ultrasound surgery – Results of different treatment protocols. Radiology 2007;243:885–93
- Hynynen K, Pomeroy O, Smith DN, Huber PE, McDannold NJ, Kettenbach J, et al. MR imaging – Guided focused ultrasound surgery of fibroadenomas in the breast: A feasibility study. Radiology 2001;219:176–85
- Furusawa H, Namba K, Nakahara H, Tanaka C, Yasuda Y, Hirabara E, et al. The evolving non-surgical ablation of breast cancer: MR guided focused ultrasound (MRgFUS). Breast Cancer 2007;14:55–8
- Liberman B, Gianfelice D, Inbar Y, Beck A, Rabin T, Shabshin N, et al. Pain palliation in patients with bone metastases using MR-guided focused ultrasound surgery: A multicenter study. Annu Surg Oncol 2009;16:140–6
- Park MY, Jung SE, Cho SH, Piao XH, Hahn ST, Han JY, et al. Preliminary experience using high intensity focused ultrasound for treating liver metastasis from colon and stomach cancer. Int J Hyperthermia 2009;25:180–8
- Klingler HC, Susani M, Seip R, Mauermann J, Sanghvi N, Marberger MJ. A novel approach to energy ablative therapy of small renal tumours: Laparoscopic high-intensity focused ultrasound. Eur Urol 2008;53:810–6
- Jung SE, Cho SH, Jang JH, Han JY. High-intensity focused ultrasound ablation in hepatic and pancreatic cancer: complications. Abdom Imaging 2010;36:185–95
- Martin E, Jeanmonod D, Morel A, Zadicario E, Werner B. High-intensity focused ultrasound for noninvasive functional neurosurgery. Ann Neurol 2009;66:858–61
- Guthkelch AN, Carter LP, Cassady JR, Hynynen K, Iacono RP, Johnson PC, et al. Treatment of malignant brain tumors with focussed ultrasound hyperthermia and radiation: results of a phase I trial. J Neurooncol 1991;10:271–84
- Park JW, Jung S, Junt TY, Lee MC. Focused ultrasound surgery for the treatment of recurrent anaplastic astrocytoma: A preliminary report. AIP Conf Proc 2006;829:238–40
- Candolfi M, Curtin JF, Nichols WS, Muhammad AG, King GD, Pluhar GE, et al. Intracranial glioblastoma models in preclinical neuro-oncology: neuropathological characterization and tumor progression. J Neurooncol 2007;85:133–48
- Barth RF, Kaur B. Rat brain tumor models in experimental neuro-oncology: The C6, 9L, T9, RG2, F98, BT4C, RT-2 and CNS-1 gliomas. J Neurooncol 2009;94:299–312
- Doblas S, He T, Saunders D, Hoyle J, Smith N, Pye Q, et al. In vivo characterization of several rodent glioma models by 1H MRS. NMR Biomed 2010;25:685–94
- Choi J, Pernot M, Small SA, Konofagou EE. Noninvasive transcranial and localized opening of the blood–brain barrier using focused ultrasound in mice. Ultrasound Med Biol 2007;33:95–104
- Chopra R, Curiel L, Staruch R, Morrison L, Hynynen K. An MRI-compatible system for focused ultrasound experiments in small animal models. Med Phys 2009;36:1867–74
- Treat LH, Zhang Y, McDannold NJ, Hynynen K. Impact of focused ultrasound-enhanced drug delivery on survival in rats with glioma. ISTU AIP Conf Proc 2009;1113:443–6
- Liu HL, Hua MY, Chen PY, Chu PC, Pan CH, Yang HW, et al. Blood–brain barrier disruption with focused ultrasound enhances delivery of chemotherapeutic drugs for glioblastoma treatment. Radiology 2010;255:415–25
- Hijnen N, Heijman E, Kohler MO, Ylihautala M, Ehnholm GJ, Simonetti A, et al. Tumour hyperthermia and ablation in rats using a clinical MR-HIFU system equipped with a dedicated small animal set-up. Int J Hyperthermia 2012;28:141–55
- O’Reilly MA, Muller A, Hynynen K. Ultrasound insertion loss of rat parietal bone appears to be proportional to animal mass at submegahertz frequencies. Ultrasound Med Biol 2011;37:1930–7