Abstract
We review the most recent and significant results published in the field of magnetotactic bacteria (MTB), in particular data relating to the use of bacterial magnetosomes in magnetic hyperthermia for the treatment of tumours. We review different methods for cultivating MTB and preparing suspensions of bacterial magnetosomes. As well as the production of magnetosomes, we also review key data on the toxicity of the magnetosomes as well as their heating and anti-tumour efficiencies. The toxicity and efficiency of magnetosomes needs to be understood and the risk–benefit ratio with which to evaluate their use in the magnetic hyperthermia treatment of tumours needs to be measured.
Introduction
Magnetic hyperthermia is a method used for treating cancers in which iron oxide nanoparticles are administered (or sent) to tumours and heated under the application of an alternating magnetic field. The heat produced by the nanoparticles induces anti-tumour activity. To date, most nanoparticles tested pre-clinically [Citation1–3], and clinically [Citation4], were chemically synthesised, mainly in the form of superparamagnetic iron oxide nanoparticles (SPION). However, SPION possess a series of drawbacks, including a thermally unstable magnetic moment that yields non-optimised magnetic properties; they are small in size which usually results in the production of a small amount of heat under the application of an alternating magnetic field, and they tend to aggregate which can cause toxicity in vivo. Such drawbacks can be avoided by using biologically synthesised nanoparticles, called magnetosomes, which are produced by several species of a group of bacteria, called magnetotactic bacteria (MTB) [Citation5]. Magnetosomes are large monodomain ferrimagnetic nanoparticles, which are composed of a core of crystallised maghemite or magnetite surrounded by biological material essentially made of lipids and proteins. They are usually arranged in chains and are used by MTB as a compass to navigate in the direction of Earth’s magnetic field. There are essentially two features that make the magnetosomes appealing for the magnetic hyperthermia treatment of tumours: (1) their large size and ferrimagnetic behaviour enable the production of a large amount of heat when they are exposed to an alternating magnetic field, and (2) their arrangement in chains prevents aggregation, yields a high rate of cellular internalisation and enables uniform heating throughout the tumour [Citation6,Citation7].
Here, we review the most recent and significant results published in the field of MTB, in particular data relating to the use of bacterial magnetosomes in magnetic hyperthermia for the treatment of tumours. We review different methods for cultivating MTB and preparing suspensions of bacterial magnetosomes. As well as the production of magnetosomes, we also review key data on the toxicity of the magnetosomes as well as their heating and anti-tumour efficiencies. The toxicity and efficiency of magnetosomes needs to be understood and the risk–benefit ratio with which to evaluate their use in the magnetic hyperthermia treatment of tumours needs to be measured.
Culture of MTB and preparation of the magnetosomes
Culture of magnetotactic bacteria
There are several species of MTB that can be cultivated, including both marine and freshwater bacteria (MS-1, MV-1, MSR-1 and AMB-1). In this review we describe the methods used for the growth of the most widely studied and commercially available species, Magnetospirillum sp. MSR-1 and AMB-1 MTB (MSR-1 DSMZ-6361 and AMB-1 ATCC-700264). The optimisation of the growth of Magnetospirillum gryphiswaldense MSR-1 MTB has been described in a series of three successive publications [Citation8–10]. First, MSR-1 MTB were cultivated in growth medium containing magnesium sulphate (MgSO4), sodium thioglycolate, yeast extracts, minerals, ferric citrate, ammonium chloride (NH4Cl) and sodium lactate in a 42-L fermenter [Citation8]. This medium was inoculated with 4.2 L of MSR-1 MTB suspension. During growth, the pH was maintained at 7 using fed-batch conditions in which a solution containing sodium lactate, NH4Cl and ferric citrate was added to the growth medium. These conditions enabled maintenance of a sustainable growth rate for the bacteria. The concentration of dissolved oxygen (DO) in the growth medium, which is an important parameter that influences both the growth rate of the bacteria and the magnetosome production yield, was kept below 10% oxygen saturation to enhance the cell biomass. The production of magnetosomes was then triggered by reducing the DO concentration to 1% oxygen saturation. Such low DO values were obtained by progressively increasing the stir rate from 100 rpm up to 300 rpm during the growth of the bacteria, while sterile air was bubbled through the growth medium at a low speed of 0.3 L/min. These conditions allowed the synthesis of a high number of magnetosomes per bacterium (∼25) and the production of a large percentage of magnetosomes containing cells (between 90 and 100%). After 60 h of growth, the magnetosome production yield and the magnetosome productivity for MSR-1 were 42 mg/L and 17 mg/L/day respectively. This growth protocol was further optimised by using a different method for more accurate control of the percentage of oxygen contained within the fermenter [Citation9]. This was achieved by changing both the stir rate and the air flow during the growth of the bacteria. After 36 h of bacterial growth, the magnetosome production yield and magnetosome productivity increased to 83 mg/L and 55 mg/L/day respectively [Citation9]. A second improvement was achieved by replacing the sources of carbon and nitrogen in the bacterial growth medium to avoid the accumulation of sodium and chloride ions, which are toxic for non-marine species of MTB such as MSR-1 and inhibit high cell concentrations [Citation10]. Under these conditions, after 36 h of growth, the magnetosome production yield and magnetosome productivity further increased to 168 mg/L and 68 mg/L/day, respectively. Another method used to cultivate MSR-1 MTB, not involving fed-batch conditions, yielded a rapid production of magnetosomes in 4 h and a magnetosome productivity of 6.3 mg/L/h and 151/mg/L/day [Citation11]. This method has the advantage of being simpler and therefore more practicable for the industrial production of MTB under good manufacturing practice (GMP) conditions. For the growth of AMB-1 MTB, it has been shown that it is possible to grow these bacteria in a large 1,000-L fermenter which can produce 2.6 g of magnetosomes after 7 days [Citation12]. In this study, the growth medium was completely saturated with nitrogen gas [Citation12]. As for MSR-1 MTB, AMB-1 MTB were also cultivated in fed-batch conditions. These bacteria were grown in a 4-L fermenter in ATCC medium 1653 and maintained at pH 6.75 by adding nitric acid solution. This enabled a magnetosome production yield of 4.5 mg/L [Citation13]. This growth protocol was further optimised by changing the feeding solution and the source of iron [Citation14]. AMB-1 MTB were cultivated in a 10-L fermenter with a pH-stat system. The feeding solution contained succinic acid, sodium nitrate, and nitric acid. Iron gallate and iron sulphate were the iron sources found to give the most optimum yield of magnetosome production. The oxygen concentration was maintained between 9 and 15% in the gas phase, which was found to be the optimum oxygen concentration for magnetosome production. Under these conditions, the magnetosome production yield reached 15 mg/L [Citation14].
The optimisations of the growth conditions described above will enable industrial developments which require the production of a large quantity of magnetosomes. These large volumes can indeed be obtained either by using a growth method that yields high level of magnetosomes via the cultivation of MSR-1 MTB [Citation10], or by the culture of MTB in large volumes [Citation12]. In this regard, iron chelating agents such as haemoglobin, EDTA and rhodamine, could be introduced into the bacterial growth medium to enhance the growth rate of magnetotactic bacteria and to increase the magnetosome production yield [Citation15]. For example, introducing 0.4 µM of haemoglobin solution into the growth medium of AMB-1 MTB increased the quantity of magnetosomes produced by a factor of ∼8 [Citation15]. Finally, reasonably high magnetosome productivity of 6.3 mg/L/h has been achieved under batch conditions, which are more favourable in an industrial setting than fed-batched conditions [Citation11].
Isolation of the magnetosomes from the magnetotactic bacteria
There are two reasons why suspensions of MTB could not be used for magnetic hyperthermia treatments of cancers: (1) the concentration of magnetosomes in a suspension of MTB is too low and results in poor heating efficiency and thus absence of any anti-tumour activity [Citation6], and (2) health and safety issues preventing the administration of whole MTB into humans. To be used in cancer therapy, the magnetosomes must therefore be isolated from the MTB. The methods used in the preparation of these isolated magnetosomes are described below. To extract the magnetosomes from MTB, four main techniques have been tested, which use sodium hydroxide (NaOH), sonication, a French press, or a pressure homogeniser to lyse the bacterial cells. The first tested method involved the mixing of MSR-1 MTB with a 5 M NaOH solution for approximately 16 h before their centrifugation for 10 h at 8,000 rpm to separate the magnetosomes from the debris of bacterial cells [Citation16]. This seemed to have resulted in magnetosomes organised in chains, clumps and loops [Citation16]. The second involved sonications at 300 W for 15 min [Citation17], 80 W for 10 min [Citation18], or 30 W for two h [Citation6], to lyse MSR-1, MS-1 and AMB-1 MTB, respectively. Using this method, the highest sonication power led to the extraction of aggregated magnetosomes [Citation17], and the lowest sonication power gave well-dispersed chains of magnetosomes [Citation6,Citation18]. The third test, involving the passing of suspensions of MTB two to three times through a French press at a pressure of 1000–20 000 lb/in2 (0.3--6.0kg/m2) to lyse AMB-1 [Citation19], and MSR-1 [Citation20,Citation21], MTB resulted in aggregated magnetosomes [Citation20,Citation21]. Finally, a pressure homogeniser has also been used to lyse MSR-1 MTB [Citation22,Citation23] at a pressure of 9000–18 000 lb/in2 (2.6--5.3 kg/m2) [Citation23]. While this method does seem to preserve the magnetosome membrane [Citation23], on which magnetosome organisation depends [Citation24], only a few transmission electron microscopic images of the magnetosomes isolated using this method have been published, making it difficult to confirm with certainty the magnetosome organisation [Citation22,Citation23]. The technique used to lyse the cells, which produces different levels of bacterial cell disruption, does affect the magnetosome organisation [Citation24].
Purification of the suspension of magnetosomes
Following extraction of the magnetosomes from whole MTB, several different methods have been suggested for their purification. One of the most comprehensive methods described involved a system of magnetic isolation followed by low power ultrasonication and treatment with urea, treatement with proteinase K to remove adsorbed and surface proteins and with electro-elution to remove nucleic acids [Citation22]. The magnetosomes were then lyophilised and sterilised with gamma rays [Citation22]. Other techniques have used phenylmethylsulphonyl fluoride to inhibit the activity of the protease; a MACS magnetic column to wash the suspension of magnetosomes with HEPES buffer [Citation25], and a treatment with 5 µg/mL of DNase I for 2 h to remove DNA [Citation26]. To examine whether the purification was successful, the suspension of magnetosomes was centrifuged and the absorption of the supernatant was measured at 280 nm. Therefore, the amount of biological material remaining in the supernatant could be quantified. A successful purification method would lead to only a small quantity of biological material remaining in the supernatant and therefore a low absorbance [Citation27]. The percentage of magnetosomes obtained from 1 g of cells depends on the extraction and purification methods used and ranges between 0.6% and 4% [Citation20,Citation25,Citation27].
Characterisation of the suspension of magnetosomes
Characterisation of the magnetosome suspension is another important aspect to be considered. Indeed, before administering a suspension of magnetosomes to humans, its full characterisation is first required. The biological material surrounding magnetosomes, which is essentially made of fatty acids, phospholipids and proteins, has been mainly characterised using chromatography, infrared spectroscopy, SDS-PAGE and mass spectroscopy. Previous studies have shown that approximately 18 proteins surrounded the magnetosomes in the MSR-1 species, representing 4% of the total weight [Citation25]. Lipids represent a higher percentage of the total weight of the magnetosomes, (∼17%), consisting of 1.4% of neutral lipids and free fatty acids, 5.1% of glycolipids, sulpholipids and phosphatides and 10.5% of phospholipids [Citation27]. The concentration of the magnetosome suspension, which needs to be known before administration, has been estimated previously using optical density at 650 nm [Citation19], or 480 nm [Citation6], and by a using a destructive assay that measures the iron concentration in the suspension [Citation6]. Transmission electron microscopy has been used to measure the sizes of the magnetosome cores, which are made of crystallised iron oxide and range between 5 and 120 nm [Citation5]. For AMB-1 and MSR-1 species, the sizes of most the magnetosomes range between 30 and 50 nm and the magnetosomes usually possess a cubo-octahedral geometry. The composition of the magnetosome core has been reported to be either magnetite, maghemite or intermediate between magnetite and maghemite, depending on the level of exposure of the magnetosomes to oxygen [Citation28]. Magnetic measurements were used to distinguish between these two compositions by measuring the presence (or not) of the Verwey transition, which revealed the presence (or not) of magnetite in the magnetosome core [Citation28].
Magnetosome toxicity and biodistribution
Cytotoxicity, acute toxicity and immunotoxicity of the bacterial magnetosomes have been assessed and the magnetosome biodistribution has been studied following both intravenous and intratumoral routes of administration. These studies were carried out using bacterial magnetosomes isolated from MTB and then purified.
Cytotoxicity of the magnetosomes
Despite their bacterial origin, suspensions of magnetosomes have shown few cytotoxic effects on mouse fibroblast H22, HL60 and EMT-6 cells [Citation29]. It was found that 9 µg/mL of bacterial magnetosomes was not toxic for H22, HL60 and EMT-6 cells. For mouse fibroblast cells incubated in the presence of various concentrations of suspensions of bacterial magnetosomes, no cytotoxicity was observed at a magnetosome concentration below 1.3 mg/mL [Citation21]. Bacterial magnetosomes incubated with MDA-MB-231 cells did not induce cell death or a significant cell inhibition at a magnetosome concentration below 125 µg/mL [Citation6].
Acute toxicity of the magnetosomes
The acute toxicity of magnetosomes has also been studied in mice and rats. Suspensions of magnetosomes at doses of 270, 360 or 480 mg/kg were injected intravenously. All mice survived except those treated with the highest dose of magnetosomes, i.e. ∼0.5% of body weight. In comparison, the mice treated with standard chemical SPION began to die after a lower dose of nanoparticles injected at 135 mg/kg [Citation32]. In another study, suspensions of magnetosomes were administered to rats intravenously. The rats survived when less than 82 mg/kg of magnetosomes were administered [Citation29]. However, for the rats that died in this study (when administered with more than 82 mg/kg of magnetosomes), it was reported that their death may have been accidental, due to bacterial infection and not to the administration of the magnetosome suspension. Histological analysis of the main organs (heart, liver, spleen, lung, kidney, brain, intestines, lymph glands and thymus) indicated the absence of haemorrhage, inflammation and hyperaemia in the rats treated with less than 82 mg/kg of bacterial magnetosomes.
Immunotoxicity of the magnetosomes
The toxicity of magnetosomes is not likely to be due to iron chemical toxicity since Fe3O4 is relatively insoluble. While dissolved iron ions may be toxic due to a Fenton reaction, which leads to the formation of hydroxyl radicals that are strong oxidants, Fe3O4 does not produce the same toxic effect. Indeed, the magnetosomes are not reported to trigger the Fenton reaction. They even seem to produce the opposite effect by scavenging reactive oxygen species [Citation31]. The toxicity of magnetosomes could also be due to their nanometric sizes that might result in embolism, blood clot, cell toxicity or acute toxicity as described above. On the other hand, it could also arise from the magnetosome biological impurities, such as the proteins, the nucleic acids and the polysaccharides potentially surrounding the magnetosomes that could lead to immunotoxicity. The latter was studied by administering a 1 mg suspension of magnetosomes into the ears of rabbits and then monitoring body temperatures after the injection [Citation29]. Body temperature did not increase suggesting the absence of a pyrogenic effect. Moreover, intravenous administration of 40 mg/kg magnetosome suspension into rats revealed no difference in the number of white blood cells and lymphocytes to those in untreated rats. The stimulation index of lymphocyte cells, which is the ratio between the number of proliferating T lymphocytes present in a lymphocyte culture after exposure to antigen to the total number of cells, was also measured for the rats treated (or untreated) with an intravenous administration of a suspension of bacterial magnetosomes using lipopolysaccharides (LPS) as antigens. No difference was observed in the stimulating index between the treated and untreated rats, suggesting the absence of immunotoxicity induced by the intravenous injection of 40 mg/kg of bacterial magnetosomes [Citation29]. Together, these results suggest that, in some specific conditions, the magnetosomes may not be pyrogenic [29]. However, in the paper by Sun et al. [29], the authors say that “it is not yet clear whether purified magnetosomes contain antigens or pyrogens” (p. 278). Therefore, their results on immunotoxicity summarised here should be interpreted with caution.
Magnetosome biodistribution
Studies have also been carried out in mice and rats to investigate the biodistribution of magnetosomes following either an intravenous or an intratumoral route of administration. Their aims were to examine the possible degradation of the magnetosomes and to determine the elimination pathways. First studied was the potential decomposition of magnetosomes by lysosomes, known to degrade nanoparticles. For this, a suspension of magnetosomes isolated from MTB was mixed with crude protease from bovine pancreas that simulates intracellular lysosomal conditions [Citation32]. The magnetosomes appeared to be degraded after 28 days, suggesting that lysosomes are indeed able to degrade magnetosomes [Citation32]. The decomposition of magnetosomes by lysosomes was further confirmed by two experimental results. Firstly, suspensions of bacterial magnetosomes were administered to rats [Citation33] or mice [Citation23,Citation32], and their localisation was determined by fluorescence microscopy [Citation23] or histological analysis of the main organs [Citation32,Citation33], 1–14 days following administration. Magnetosomes were found in the liver [Citation32,Citation33], spleen [Citation32], and lungs [Citation23], where they were located inside lysosomes in a partly degraded state. In these studies, the magnetosomes that ended up in the liver and spleen were digested by lysosomes [Citation32,Citation33]. Secondly, another study showed that three days following intravenous injection the magnetosomes were neither found in the faeces nor in the urine [Citation33]. These results seem to indicate that the magnetosomes were degraded by the mice or rats. The hypothesis proposed in light of these results was that the reticuloendothelial system, composed of liver and spleen macrophages, removes magnetosomes from the bloodstream [Citation33]. Other studies, however, have found magnetosomes in the faeces of mice following both intra-tumoral and intravenous routes of administrations [6]. Taken together, these results suggest that a portion of the bacterial magnetosomes is probably degraded while another portion is eliminated in the faeces. Biodistribution studies of chemically synthesised nanoparticles revealed different features. For example, long-term follow-up over a period of 6 months of chemically synthesised nanoparticles injected intravenously showed that these nanoparticles were metabolised in the organ’s parenchyma with metal ions leaking from the core material [Citation34]. However, there are still too few results published on the magnetosome biodistribution to be able to compare in detail the biodistribution of the magnetosomes with that of chemically synthesised nanoparticles. Moreover, further studies are necessary to determine more precisely the elimination and degradation pathways of bacterial magnetosomes and to examine whether or not the magnetosomes are metabolised.
Magnetic hyperthermia treatment of tumours with magnetosomes
Heating properties of the magnetosomes
Bacterial magnetosomes are good candidates to carry out magnetic hyperthermia since they produce a large amount of heat when exposed to an alternating magnetic field. In this review, we report values of magnetosome losses per cycle as a measurement of the amount of heat produced by magnetosomes. The magnetosome loss per cycle is defined as the magnetosome specific absorption rate (SAR) divided by the frequency of oscillation of the alternating magnetic field, where the SAR is usually estimated using the formula SAR = ρsCs(ΔT/δt)/CFe, where ρs, Cs and CFe are the density, calorific capacity and iron concentration of the sample respectively, and ΔT/δt is the slope of the variation in temperature with time estimated at the beginning of the heat treatment [Citation35]. Using loss per cycle is convenient since it enables comparison between SAR obtained for different alternating magnetic field frequencies. The production of a large amount of heat by the magnetosomes is due to their large sizes and ferrimagnetic behaviour at physiological temperatures. Indeed, since the magnetosomes are monodomain, ferrimagnetic and of cubic shape, the amount of heat that they produce is proportional to their sizes. In addition, due to their thermally stable magnetic moment, the magnetosomes produce a larger amount of heat than the smaller super-paramagnetic chemically synthesised nanoparticles. These behaviours have been shown both experimentally and theoretically [Citation35–41]. For suspensions of magnetosomes exposed to a magnetic field of 6.2 mT, losses per cycles of 0.1–0.2 J/kg were reported [Citation35–39]. When the strength of the magnetic field was increased to 12 mT, the losses per cycle increased to 0.5–1 J/kg (33, 37–39), showing that the magnetosome loss per cycle strongly increases with increasing magnetic field strength. By comparison with chemically synthesised nanoparticles, these losses per cycle are considerably smaller at magnetic field strengths below 10 mT, and either equivalent [Citation39], or larger at magnetic field strengths above 10 mT [Citation40]. The heating mechanisms of bacterial magnetosomes have also been studied. Three different types of sample were prepared containing whole MTB, chains of magnetosomes extracted from AMB-1 MTB or individual magnetosomes detached from the chains by heat and SDS treatments [Citation40]. shows transmission electron microscopy images of whole MTB (A), chains of magnetosomes extracted from MTB (B) and individual magnetosomes (C). When suspensions of whole AMB-1 MTB were mixed with water and exposed to an alternating magnetic field frequency of 108 KHz and strength of 23 to 88 mT, the losses per cycle ranged between 1.1 and 8 J/kgFe and the heat released by the magnetosomes was found to mainly arise from the inversion of the magnetosome magnetic moment under the application of an alternating magnetic field (magnetic viscosity). Indeed, it has been demonstrated that the contribution of the rotation of the whole bacteria to the production of heat was negligible [Citation40]. When the magnetosomes were extracted from whole MTB either as chains or individual nanoparticles, the heat released by the magnetosomes was reported to arise both from the inversion of the magnetosome magnetic moment (magnetic viscosity) and from the magnetosome rotation (hydrodynamic viscous coupling of the medium). Consequently, under the application of the same magnetic field strengths, the losses per cycle increased considerably to 5–11 J/kgFe compared with the values obtained using whole bacteria. It was not possible to determine from this study alone if the magnetosome chains or the individual magnetosomes would be the best candidate to carry out magnetic hyperthermia treatment of tumours, since both types of magnetosomes led to high heating efficiencies. The influence on the heating properties of the magnetosome size and magnetosome magnetic anisotropy has also been studied [Citation15,Citation30]. To increase the magnetosome magnetic anisotropy, a 2 µM solution of cobalt quinate was introduced into the bacterial growth medium resulting in an increase in the magnetocristalline anisotropy constant by a factor of ∼9 from kgeff = 12 kJ/m3 in the absence of cobalt up to Keff = 104 KJ/m3 in the presence of cobalt quinate [Citation30]. This increase in magnetosome magnetic anisotropy resulted in an increase in the SAR [Citation30]. To increase magnetosome sizes, iron chelating agents such as EDTA and rhodamine B were introduced in the bacterial growth medium. This resulted in an increase of the mean magnetosome sizes from 42.5 nm in the absence of iron chelating agents up to 60–65 nm in the presence of rhodamine B or EDTA that produced an enhancement of the magnetosome heating properties [Citation15].
Anti-tumour activity of the magnetosomes
Although bacterial magnetosomes belong to a type of iron oxide nanoparticles that produces a large amount of heat when exposed to an alternating magnetic field [Citation6,Citation35–40], the anti-tumour activity resulting from a magnetic hyperthermia treatment has to our knowledge only been evaluated in two studies [Citation6,Citation32]. When MCF-7 tumour cells were mixed with bacterial magnetosomes and heated under the application of an alternating magnetic field to reach a temperature of 47 °C, 80% of the cell proliferation was inhibited [Citation32]. It was also mentioned in this study that a temperature of 47 °C inhibited more MCF-7 tumour cells than normal L929 cells. This result further supports the safety of the treatment. Indeed, it suggests that if during the course of a magnetic hyperthermia treatment healthy tissues are heated by mistake, the heat would be less damaging to healthy tissues than to tumour tissues. MDA-MB-231 tumour cells were also incubated with a suspension of magnetosomes and exposed to an alternating magnetic field under various conditions [Citation6]. The percentage of cell death and cell inhibition increased with increasing concentrations of magnetosomes, increasing incubation times, magnetic field strength and the number of times that the heat treatment was repeated. This suggests that an efficient treatment can be achieved provided optimum values of these four parameters are chosen.
We performed a proof of concept study for the therapy on breast tumours xenografted under the skin of mice [Citation6]. Four different types of suspensions were tested containing whole MTB (), magnetosomes organised in chains (), individual magnetosomes () and SPION. Each suspension contained 10 mg/mL of iron oxide, and 100 µL of each suspension was administered with a syringe into the centre of the xenografted breast tumours (∼100 mm3 in size) The mice were then exposed three times for 20 min to an alternating magnetic field strength of 40 mT and frequency of 198 kHz. The schematic diagram in shows the set-up for the treatment using an induction system that generates an alternating magnetic field. The treatments, which used the suspensions of whole MTB, individual magnetosomes and SPION, were inefficient and were unable to stop the growth of the tumour. In contrast, when the heat treatment was carried out using suspensions of chains of magnetosomes, the tumours in several mice disappeared. shows a mouse during the treatment. Typical temperatures reached during the treatment with the chains of magnetosomes were around 45–46 °C as estimated by infrared measurements (). Thirty days following the treatment, the tumour had disappeared in the mouse treated with the chains of magnetosomes (), while it was still located at the skin surface of the mice treated with individual magnetosomes () or SPION (). We can conclude from these results that the efficiency of the bacterial magnetosomes is not only due to their large sizes but also comes from their arrangement in chains. When the magnetosomes are organised in chains, they are less prone to aggregation. Indeed, several chains of magnetosomes interact in a head to tail manner in such a way that they form a longer chain. This behaviour can clearly be observed in . In , the chains of magnetosomes contained inside the magnetotactic bacterium are short, containing ∼10 magnetosomes. After extraction from the magnetotactic bacteria, the chains of magnetosomes are much longer, containing typically more than ∼20 magnetosomes (). By contrast, when the magnetosomes are not organised in chains, they tend to form compactly aggregated assemblies of individual magnetosomes. This has been shown by studying the organisation of individual magnetosomes obtained by heat and SDS treatments [Citation6], or by genetic manipulations of magnetotactic bacteria that led to the removal of the filament binding the magnetosomes together in a chain inside MTB [Citation42].
Figure 2. The set-up used to carry out the treatment of the mice by positioning the mice inside the copper coil and by applying an alternating magnetic field (A). The measurement of the temperature during the treatment (B). Photographs of the mice treated with a suspension containing chains of magnetosomes (C), individual magnetosomes, (D), or superparamagnetic iron oxide nanoparticles (SPION) (E).
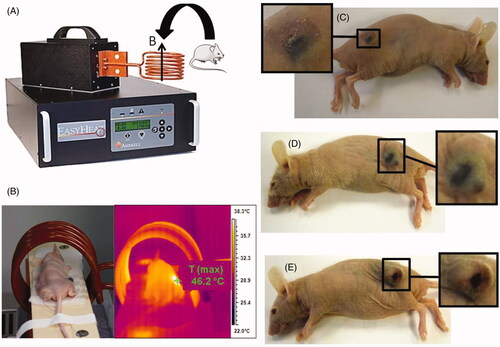
We then examined the impact of the chain organisation of the magnetosomes on the heating and distribution uniformity as well as on cellular internalisation [Citation6,Citation7]. The heating uniformity was studied by infrared measurements and histological analysis, which showed a more uniform heating and distribution throughout the tumour for the chains of magnetosomes than for the individual magnetosomes. The internalisation of the chains of magnetosomes inside the cells was observed by two types of measurements. The chains of magnetosomes were mixed with MDA-MB-231 cells and exposed to an alternating magnetic field which resulted in the formation of magnetic cells 5 min after the beginning of the treatment. shows an Eppendorf tube containing MDA-MB-231 cells mixed with chains of magnetosomes being exposed to the alternating magnetic field. Before the application of the alternating magnetic field, shows that the cells are not attracted by a magnet positioned next to the Eppendorf tube and are therefore non-magnetic. Therefore, only a small black spot located next to the magnet is visible corresponding to magnetosomes without cells being attracted by the magnet. By contrast, after the application of the alternating magnetic field, as shown in , a large dark spot appeared next to the magnet relating to the magnetic cells attracted by the magnet. MDA-MB-231 cells were also incubated in the presence of the chains of magnetosomes for 1 min, and 24 h using Prussian blue, which reveals the presence of iron. shows the absence of magnetosome internalisation after 1 min, and shows an efficient internalisation after 24 h of incubation. These results indicate that both the application of the alternating magnetic field and the incubation of magnetosomes for a long period of time cause high cellular internalisation. Histological analysis of the tumour tissues revealed the presence of aggregated magnetosomes in the mice treated with individual magnetosomes (), whereas magnetosomes internalised within the cells were observed only in the mice treated with suspensions of chains of magnetosomes (). We concluded that uniform heating, uniform magnetosome distribution, as well as effective cellular internalisation were responsible for the high anti-tumour efficiency observed with the chains of magnetosomes. The large sizes of the magnetosomes, which yield high values of the SAR, are not sufficient to produce high anti-tumour activity. The distribution of the magnetosomes in the tumour is another key parameter that influences the efficiency of the treatment.
Figure 3. The set-up used to expose a suspension containing chains of magnetosomes mixed with MDA-MB-231 cells to an alternating magnetic field of average magnetic field strength of ∼20 mT and frequency of 198 kHz (A). A photograph showing this suspension placed next to a magnet before the application of the alternating magnetic field (B), or after the application of the alternating magnetic field for 5 min (C). Micrographs of MDA-MB-231 cells incubated with a suspension containing chains of magnetosomes for 3 min (D), or 24 h (E).
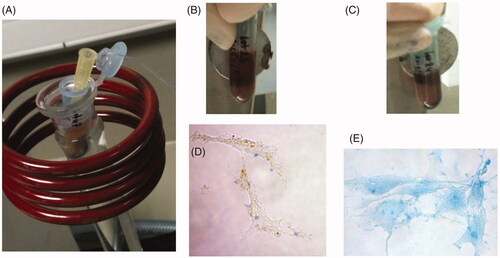
Figure 4. Micrograph of a tumour tissue collected after the administration of the bacterial magnetosomes and three applications of an alternating magnetic field of average strength (20 mT) and frequency of 198 kHz for 20 min. The bacterial magnetosomes administered were individual magnetosomes (A), or chains of magnetosomes (B), (C). (C) is an enlargement of (B) and shows bacterial magnetosomes internalised within tumour cells.
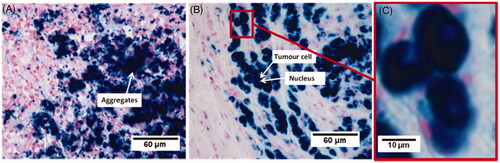
Comparison between the chains of magnetosomes and the individual magnetosomes
Chains of magnetosomes have been defined as assemblies of magnetosomes in which the crystallographic directions of most of the magnetosomes are aligned in the direction of the chain elongation [Citation43]. Individual magnetosomes are assemblies of magnetosomes in which such alignment does not take place. The chains of magnetosomes differ from the individual magnetosomes by their coating. Using infrared measurements, it has been shown that the chains of magnetosomes were coated by a mixture of lipids and proteins, whereas the individual magnetosomes were only coated by lipids [Citation7]. Most probably due to different coatings, the chains of magnetosomes were found to have a zeta potential (–22 mV at pH 7), which differs from that of the individual magnetosomes (+10 mV at pH 7) [Citation7]. The magnetic anisotropy of these two types of magnetosomes was studied by depositing a suspension of these two types of magnetosomes on a substrate under the application of a magnetic field [Citation44]. Magnetic anisotropy was evaluated by measuring the hysteresis loops of these two types of magnetosomes for a magnetic field applied either parallel or perpendicular to the direction of the alignment and by measuring the difference in coercivity and remanent magnetisation between these two field configurations. A larger magnetic anisotropy was found for the chains of magnetosomes than for the individual magnetosomes. This comes from the better alignment of the easy axes in chains of magnetosomes than in individual magnetosomes [Citation44]. The larger magnetic anisotropy of the chains of magnetosomes compared with that of the individual magnetosomes could be another factor responsible for their lower tendency to aggregate. The chains of magnetosomes possess advantageous properties for medical applications due to their low-level aggregation. Such property results in high cellular internalisation and in uniform tumour distribution. It makes the chains leave the tumour more rapidly than the individual magnetosomes or chemically synthesised nanoparticles. It also results in a percentage of chains eliminated in the faeces following intratumoral administration, which is larger than that obtained with individual magnetosomes [Citation6].
Conclusion
In conclusion, we have reviewed the most recent data published on the use of magnetosomes for magnetic hyperthermia treatment of tumours. Studies report that it is possible to produce magnetosomes in large quantity (150 mg/L/day) and to purify the magnetosomes in such a way that toxicity is avoided. The acute toxicity of the magnetosomes was found to be low, with rats surviving up to a quantity of magnetosomes administered of 480 mg per kg of animal. Suspensions of magnetosomes heated under the application of an alternating magnetic field yield high loss power per cycle of 5–11 J/kgFe, suggesting that the magnetosomes are good candidates for magnetic hyperthermia treatments of tumours. Moreover, a proof of concept of a therapy using bacterial magnetosomes as heating sources has been carried out on breast tumours xenografted under the skin of mice. It has revealed that it was possible to completely eradicate the xenografted tumours using this technique. Finally, two different types of magnetosomes have been studied, those organised in chains, which possess cristallographic directions aligned in the direction of the chain elongation, and those organised as individual magnetosomes, which do not possess such alignment of their cristallographic directions. It has been shown that the chains of magnetosomes produce the best therapeutic results, mainly because of their low level of aggregation, which results in high cellular internalisation and uniform distribution throughout the tumour.
Declaration of interest
The authors report no conflicts of interest. The authors alone are responsible for the content and writing of the paper.
References
- Jordan A, Scholz R, Maier-Hauff K, Van Landeghem FK, Waldoedner N, Teichgraeber U, et al. The effect of thermotherapy using magnetic nanoparticles on rat malignant glioma. J Neurooncol 2006;78:7–14
- Ito A, Tanaka K, Kondo K, Shinkai M, Honda H, Matsumoto K, et al. Tumor regression by combined immunotherapy and hyperthermia using magnetic nanoparticles in an experimental subcutaneous murine melanoma. Cancer Sci 2003;94:308–13
- Zhao Q, Wang L, Cheng R, Mao L, Arnold RD, Howerth E W, et al. Magnetic nanoparticle-based hyperthermia for head and neck cancer in mouse models. Theranostics 2012;2:113–21
- Maier-Hauff K, Ulrich F, Nestler D, Niehoff H, Wust P, Thiesen B, et al. Efficacy and safety of intratumoral thermotherapy using magnetic iron-oxide nanoparticles combined with external beam radiotherapy on patients with recurrent glioblastoma multiforme. J Neurooncol 2011;103:317–24
- Bazylinski DA, Frankel RB. Magnetosome formation in prokaryotes. Nature Rev Microbiol 2004;2:217–30
- Alphandéry E, Faure S, Seksek O, Guyot F, Chebbi I. Chains of magnetosomes extracted from AMB-1 magnetotactic bacteria for application in alternative magnetic field cancer therapy. ACS Nano 2011;5:6279–96
- Alphandéry E, Guyot F, Chebbi I. Preparation of chains of magnetosomes, isolated from Magnetospirillum magneticum AMB-1 magnetotactic bacteria, yielding efficient treatment of tumors using magnetic hyperthermia. Int J Pharm 2012;434:444–52
- Sun J-B, Zhao F, Tang T, Jiang W, Tian J-S, Li J-L. High-yield growth and magnetosome formation by Magnetospirillum gryphiswaldense MSR-1 in an oxygen-controlled fermentor supplied solely with air. Appl Microbiol Biotechnol 2008;79:389–97
- Liu Y, Li GR, Jiang W, Li Y, Li LJ. Large-scale production of magnetosomes by chemostat culture of Magnetospirillum gryphiswaldense at high cell density. Microb Cell Fact 2010;9:99
- Zhang Y, Zhang X, Jiang W, Li Y, Li J. Semicontinuous culture of Magnetospirillum gryphiswaldense MSR-1 cells in an autofermentor by nutrient-balanced and isosmotic feeding strategies. Appl Environ Microbiol 2011;77:5851–6
- Heyen U, Schüler D. Growth and magnetosome formation by microaerophilic Magnetospirillum strains in an oxygen-controlled fermentor. Appl Microbiol Biotechnol 2003;61:536–44
- Matsunaga T, Tadokoro F, Nakamura N. Mass culture of magnetic bacteria and their application to flow type immunoassays. IEEE Trans Magn 1990;26:1557–9
- Matsunaga T, Tsujimura N, Kamiya S. Enhancement of magnetic particle production by nitrate and succinate fed-batched culture of Magnetospirillum sp. AMB-1. Biotechnol Tech 1996;10:495–500
- Yang C-D, Takeyama H, Tanaka T, Matsunaga T. Effects of growth medium composition, iron sources and atmospheric oxygen concentrations on production of luciferase-bacterial magnetic particle complex by a recombinant Magnetospirillum magneticum AMB-1. Enzyme Microbila Technol 2001;29:13–19
- Alphandéry E, Amor M, Guyot F, Chebbi I. The effects of iron-chelating agents on Magnetospirillum magneticum strain AMB-1: Stimulated growth and magnetosome production and improved magnetosome heating properties. Appl Micriobiol Biotechnol 2012;96:663–70
- Philipse AP, Maas D. Magnetic colloids from magnetotactic bacteria: Chain formation and colloidal stability. Langmuir 2002;18:9977–84
- Sun J-B, Duan J-H, Dai S-L, Ren J, Guo L, Jiang W, Li Y. Preparation and anti-tumor efficiency evaluation of doxorubicin-loaded bacterial magnetosomes: Magnetic nanoparticles as drug carriers isolated from Magnetospirillum gryphiswaldense. Biotechnol Bioeng 2008;101:1313–20
- Taoka A, Asada R, Sasaki H, Anzawa K, Wu LF, Fukumori Y. Spatial localizations of Mam22 and Mam12 in the magnetosomes of Magnetospirillum magnetotacticum. J Bacteriol 2006;188:3805–12
- Matsunaga T, Maeda Y, Yoshino T, Takeyama H, Takahashi M, Ginya H, et al. Fully automated immunoassay for detection of prostate-specific antigen nano-magnetic beads and micro-polystyrene bead composites, ‘Beads on Beads’. Anal Chim Acta 2007;597:331–9
- Grünberg K, Müller E-C, Otto A, Reska R, Linder D, Kube M, et al. Biochemical and proteomic analysis of the magnetosome membrane in Magnetospirillum gryphiswaldense. Appl Environ Microbiol 2004;70:1040–50
- Xiang L, Wei J, Jianbo S, Gulli W, Feng G, Ying L. Purified and sterilized magnetosomes from Magnetospirillum gryphiswaldense MSR-1 were not toxic to mouse fibroblasts in vitro. Lett Appl Microbiol 2007;45:75–81
- Guo F, Liu Y, Chen Y, Tang T, Jiang W, Li Y, Li J. A novel rapid and continuous procedure for large-scale purification of magnetosomes from Magnetospirillum gryphiswaldense. Appl Microbiol Biotechnol 2011;90:1277–83
- Tang T, Zhang L, Gao R, Dai Y, Meng F, Li Y. Fluorescence imaging and targeted distribution of bacterial magnetic particles in nude mice. Appl Microbiol Biotechnol 2012;94:495–503
- Kobayashi A, Kirschvink J, Nash CZ, Kopp RE, Sauer DA, Bertani LE, et al. Experimental observation of magnetosome chain collapse in magnetotactic bacteria: Sedimentological paleomagnetic, and evolutionary implications. Earth Planet Sci Lett 2006;245:538–50
- Grünberg K, Wawer C, Tebo BM, Schüler D. A large gene cluster encoding several magnetosome proteins is conserved in different species of magnetotactic bacteria. Appl Environ Microbiol 2001;67:4573–82
- Sun J-B, Duan J-H, Dai S-L, Ren J, Zhang Y-D, Tian J-S, Li Y. In vitro and in vivo antitumor effects of doxorubicin loaded with bacterial magnetosomes (DBMs) on H22 cells: The magnetic bio-nanoparticles as drug carriers. Cancer Lett 2007;258:109–17
- Gorby YA, Beveridge TJ, Blakemore RP. Characterization of the bacterial magnetosome membrane. J Bacteriol 1988;170:834–41
- Alphandéry E, Ngo AT, Lefèvre C, Lisiecki I, Wu LF, Pileni MP. Difference between the magnetic properties of the magnetotactic bacteria and those of the extracted chains of magnetosomes: Influence of the distance between the chains of magnetosomes. J Phys Chem C 2008;112:12304–9
- Sun J, Tang T, Duan J, Xu P-X, Wang Z, Zhang Y, et al. Biocompatibility of bacterial magnetosomes: Acute toxicity, immunotoxicity and cytotoxicity. Nanotoxicology 2010;4:271–83
- Alphandéry E, Carvollo C, Menguy N, Chebbi I. Chains of cobalt doped magnetosomes extracted from AMB-1 magnetotactic bacteria for application in alternative magnetic field cancer therapy. J Phys Chem C 2011;115:11920–4
- Guo FF, Yang W, Jiang W, Geng S, Peng T, Li JL. Magnetosomes eliminate intracellular reactive oxygen species in Magnetospirillum gryphiswaledense MSR-1. Environ Microbiol 2012;14:1722–9
- Liu R-T, Liu J, Tong J-Q, Tang T, Kong W-C, Wang X-W, et al. Heating effect and biocompatibility of bacterial magnetosomes as potential materials used in magnetic fluid hyperthermia. Prog Nat Sci Mater Int 2012;22:31–9
- Sun J-B, Wang Z-L, Duan J-H, Ren J, Yang X-D, Dai S-L, et al. Targeted distribution of bacterial magnetosomes isolated from Magnetospirillum gryphiswaldense MSR-1 in healthy Sprague-Dawley rats. J Nanosci Nanotechnol 2009;9:1881–5
- Lacava LM, Garcia VAP, Kückelhaus S, Azevedo RB, Sadeghiani N, Buske N, et al. Long-term retention of dextran-coated magnetite nanoparticles in the liver and spleen. J Magn Magn Mater 2004;272:2434–5
- Timko M, Dzarova A, Skumiel A, Jozefcak A, Hornowski T, Gojzewski H, et al. Magnetic properties and heating effect in bacterial magnetic nanoparticles. J Magn Magn Mater 2009;321:1521–4
- Timko M, Molcan M, Hashim A, Skumiel A, Muller M, Gojzewski H, et al. Hyperthermic effect in suspension of magnetosomes prepared by various methods. IEE Trans Magn 2013;49:250–4
- Hergt R, Dutz S, Roder M. Effects of size distribution on hysteresis losses of magnetic nanoparticles for hyperthermia. J Phys Condens Matter 2008;20:385214--25
- Hergt R, Hiergeist R, Zeisberger M, Schüler D, Heyen U, Hilger I, et al. Magnetic properties of bacterial magnetosomes as potential diagnostic and therapeutic tools. J Magn Magn Mater 2005;293:80–6
- Dutz S, Hergt R, Mürbe J, Müller R, Zeisberger M, Andrä W, et al. Hysteresis losses of magnetic nanoparticle powders in the single domain size range. J Magn Magn Mater 2007;308:305–12
- Alphandéry E, Faure S, Raison L, Duguet E, Howse PA, Bazylinski DA. Heat production by bacterial magnetosomes exposed to an oscillating magnetic field. J Phys Chem C 2011;115:18–22
- Rosensweig RE. Heating magnetic fluid with alternating magnetic field. J Magn Magn Mater 2002;252:370–4
- Scheffel A, Gruska M, Faivre D, Linaroudis A, Grumann P, Plitzko JM, et al. An acidic protein aligns magnetosomes along a filamentous structure in magnetotactic bacteria. Nature 2006;440:110–14
- Alphandéry E, Chebbi I, Faure S. Treatment of cancer or tumor induced by the release of heat generated by various chains of magnetosomes extracted from magnetotactic bacteria and submitted to an alternative magnetic field. Patent WO2011/061259. First submission Nov 2009
- Alphandéry E, Ding Y, Ngo AT, Wang ZL, Wu LF, Pileni MP. Assemblies of aligned magnetotactic bacteria and extracted magnetosomes: What is the main factor responsible for the magnetic anisotropy? ACS Nano 2009;3:1539–47