Abstract
Purpose: The aim of this study was to numerically and experimentally characterise the influence of tissues dimensions on the size and shape of microwave-induced ablation zones. Materials and methods: A 2.45 GHz interstitial antenna was introduced into ex vivo bovine liver samples, delivering 60 W for 10 min; then the dimensions of the coagulated area were measured. Ablations were performed both in large samples (termed unrestricted tissue) for characterising the tissue response, and in thin samples, whose dimensions in the plane perpendicular to the antenna were smaller than the short axis of the ablated area obtained in unrestricted samples. In the numerical study the electromagnetic field emitted from the antenna and the corresponding temperature increase were evaluated in both unrestricted and thin tissue samples. Results: When the height of the tissue was smaller than the ablation diameter measured in unrestricted samples, a 7.5% increase in length of the ablated zone was experimentally observed. When both the height and width were lower than the diameter measured in unrestricted samples, an elongation of about 23.4% was experimentally obtained. The numerical study showed that the boundary conditions between the target tissue and the surrounding materials are critical. Conclusions: The ex vivo performances of microwave ablation devices are notably influenced by the shape and dimension of the tissues where the procedure takes place. Accordingly, dedicated interventional protocols should be developed for treatment planning on targets of different shape and size.
Introduction
Microwave thermal ablation (MWA) is a therapeutic technique used to destroy pathological tissue thanks to the very high temperature increase obtained by the absorption of an electromagnetic (EM) field at microwave frequencies. Thermal ablation is achieved when the temperature of the target tissue increases up to about 60 °C [Citation1,Citation2]. At this temperature nearly instantaneous protein coagulation is induced, which in turn leads to cell death [Citation3,Citation4].
Clinical applications of MWA are the treatment of heart diseases [Citation5], endometrial disorders [Citation6], and several types of solid tumours [Citation7,Citation8]. In particular, the most recent developments are mainly focused on the treatment of hepatocellular carcinoma (HCC) and secondary liver tumours (with particular reference to colorectal cancer metastasis) in non-surgical patients [Citation9–11]. In fact, while surgical resection remains the gold standard therapy for the treatment of liver malignancies, often this option is not viable due to impaired liver function, or number and distribution of tumour masses, or too high intra-operative risks, co-morbidities [Citation12]. Accordingly, minimally invasive techniques, such as MWA, are gaining attention and consensus.
The correct application of thermal ablation systems relies on the definition of clinical protocols, which link the microwave power emitted by an interstitial antenna and the time of irradiation with the achievable dimensions of the ablated zone [Citation13,Citation14]. To this aim, a preliminary but necessary step is the characterisation of the coagulative performance of a given interstitial antenna. The typical characterisation test is performed by inserting the antenna under evaluation into ex vivo tissue and allowing it to radiate a given amount of microwave power for the time of choice. Then, at the end of the procedure, the tissue is sectioned and the coagulated zone (named thermal lesion also) is measured [Citation15]. Similar studies are performed in vivo on animals to characterise the influence of blood perfusion [Citation16]. Numerical simulations could also be used to predict the induced thermal lesion. In this case, the Maxwell’s equations (ME) are solved to calculate the EM field distribution into the target tissue; then the temperature distribution is obtained by solving the so-called bioheat equation (BHE), in which all the mechanisms of heat production and heat exchange typical of human tissues are considered [Citation17,Citation18].
A number of parameters influence the outcomes of MWA procedures, such as the microwave antenna design, the frequency of operation, the power and time of irradiation, the characteristics of the target tissue, the presence of blood [Citation19,Citation20]. Several studies have been conducted on different antenna designs, and on the different shapes and dimensions of the achievable thermal lesions [Citation21,Citation22]. Nevertheless, to the authors’ knowledge, no studies are yet available on the influence that the shape and dimension of the target tissue have on the outcomes of the thermal ablation procedure. Indeed, dimensional constraints or location of the radiating antenna close to the tissue boundary are quite common conditions in pre-clinical or clinical scenarios (e.g. porcine liver lobes, sub-capsular hepatic lesions, exophytic renal tumours, exposed nodules treated intra-surgically). On the other hand, if the tissue has dimensions close to those achievable for the coagulated area, the boundary between the tissue under test and the air (ex vivo) or other surrounding tissues with different thermal properties (in vivo) could influence the outcomes of the study.
In this work, the coagulative performances of a 2.45 GHz coaxial antenna for interstitial MWA were studied both experimentally and numerically in ex vivo bovine liver. The aim of the work was to put into evidence whether the presence of a boundary between tissue and air close to the radiating antenna could have any influence on the ablative performance of the device itself. To this end, both unrestricted tissue samples, i.e. whose dimensions were appreciably larger than the final ablation volume, and thin samples, i.e. smaller in thickness and/or width compared to the short axis of the coagulated zone measured in unrestricted samples, were considered. Furthermore, the numerical study investigated the role of the thermal boundary at the sample surface.
Materials and methods
Experimental set-up
Ex vivo experiments were conducted independently in two laboratories, the Casaccia Research Centre (ENEA) and HS Hospital Service, S.p.A, Rome, Italy (HS) using the same set-up and procedure ().
The microwave applicator was a 14-gauge coaxial asymmetric dipole antenna with a miniaturised quarter-wave choke, equipped with an internal cooling system (AMICA PROBE, HS Hospital Service S.p.A., Rome, Italy) [Citation23,Citation24]. The antenna was inserted into ex vivo bovine liver and supplied with a continuous wave (CW) signal at 2.45 GHz from a microwave solid state power generator (AMICA GEN, HS Hospital Service S.p.A., Rome, Italy). A two-channel power meter (Agilent E4419B, Agilent Technologies Inc., Santa Clara, CA) was used for monitoring, through a type-N dual-coaxial reflectometer (Narda 3022, Narda Microwave – East, Hauppage, NY), the power supplied to and reflected by the microwave antenna. All the power levels reported in this work are referred to the antenna input port (net power). A peristaltic pump integrated in the microwave generator, whose operation is automatic and synchronised with energy delivery start and stop, ensured closed-loop water circulation at constant flow rate (40 ml/min) within the antenna, up to the choke section. The coolant reservoir was a thermostatic bath, kept at 10 °C. Software purposely developed in Labview™ (National Instruments Corporation, Austin, TX) and running on a PC managed the whole MWA session, and automatically stored the measured data.
In , H and W represent, respectively, the height (mm) and the width (mm) of the tissue sample in the plane perpendicular to the antenna, while P is the insertion depth (mm) of the antenna into the sample.
Numerical model
The numerical study was conducted solving the EM problem, represented by the ME, and the thermal problem, represented by the BHE. The BHE equates the several mechanisms responsible for heat accumulation or dissipation into a biological tissue to the temperature increase (or decrease) into the tissue [Citation17], as reported by Equation Equation1(1) :
(1)
In Equation Equation1(1) , C (J/(kg °C)) represents the specific heat, ρ (kg/m3) the mass density, k (W/(m °C)) the thermal conductivity, M (W/m3) the metabolic heat production, Pdiss (W/m3) is the microwave power density dissipated in the tissue, B (W/(m3 °C)) accounts for blood perfusion, and TB (°C) is the temperature of blood. The ex-vivo condition was obtained in Equation Equation1
(1) discarding the contributions from the metabolic heat and blood perfusion. At the tissue margin a boundary condition representing the heat exchange through convection must also be considered, as reported in Equation Equation2
(2) .
(2)
where S is the boundary surface (mm2), n0 is the outward unit vector normal to S, H (W/(m °C)) is the convective coefficient, TS is the surface temperature of the tissue (°C), and Te is the temperature (°C) of the surrounding environment.
Simulations were performed using self-developed codes in cylindrical coordinates [Citation25,Citation26], which solved the EM problem by way of the finite difference time domain (FDTD) method, and the thermal problem by way of an explicit finite difference (FD) solution of the BHE. shows the simulated geometry. The antenna’s axis coincides with the z-axis, and r represents the radial distance from the antenna; given the cylindrical symmetry, the simulated domain reduces to one half of the transversal plane. Finally, a graded mesh is used in the radial direction to reduce the computational burden [Citation27].
The developed codes have been validated with reference to ablation procedures against numerical and experimental data [Citation23,Citation25,Citation26]. The numerical model of the antenna represents with the antenna used in the experimental set-up, and it was validated through comparisons with experimental data [Citation28]. In the present study the fine-region cells of the graded mesh were 0.03 mm × 0.05 mm (r,z) wide, while the largest cell size was 0.5 mm × 0.05 mm (r,z). In this way, the antenna’s geometry is modelled with an adequate number of FD cells, and optimisation of the computational requirements is allowed as well. The antenna cooling system was modelled through a convective boundary condition along the antenna feeding cable; the convection coefficient was settled equal to 1000 W/m2 °C, and the temperature of the refrigerating liquid to 18.6 °C [Citation29]. The chosen value for the numerical temperature of the cooling liquid is based on measurements purposely carried out; starting from the temperature of 10 °C of the thermostatic bath, the cooling liquid reaches a final temperature of 18.6 °C while travelling along the feeding coaxial cable due to antenna losses into the cable structure [Citation29].
The antenna was inserted into liver tissue whose dielectric and thermal properties at 37 °C are reported in . The initial temperature of the set-up was equal to that measured in the experimental trials, i.e. 16 °C. During the ablation procedure, the temperature increase changes the dielectric properties of the tissue, leading to a reduction of both the relative permittivity and the electric conductivity [Citation30–32]. Moreover, water vaporisation takes place close to the radiating antenna; this phenomenon can be modelled as a changing specific heat [Citation33]. All these phenomena were included in the numerical simulation [Citation34,Citation35].
Table 1. Dielectric and thermal properties of liver and fat tissue at 37 °C.
The thermal lesion was numerically defined as the area where the tissue temperature exceeded 60 °C. To this aim, the temperature was computed at several positions close to the antenna starting from an initial temperature of 16 °C, close to that measured during the experimental trials. Correspondingly, a temperature increase of at least 44 °C was numerically necessary to achieve tissue ablation.
Experimental procedure
Measurements were conducted with the antenna fed with 60 W at 2.45 GHz for 10 min. Upon ablation completion, the tissue sample was sectioned to expose the thermal lesion for visual inspection and for measuring its dimensions.
Thermal lesions in unrestricted tissue showed a circular cross-section in the transversal plane (i.e. orthogonal to the antenna’s axis: see ) and an elliptical cross-section in the longitudinal plane (i.e. along the antenna axis), as a consequence of the cylindrical symmetry of the antenna when operated in unrestricted electromagnetically homogeneous tissue. Therefore, the ellipsoidal coagulation zone found in unrestricted targets may be fully described by two geometric parameters alone: the long axis length L (i.e. the maximum coagulation size in the longitudinal plane) and the short axis diameter D (i.e. the maximum coagulation size in the transversal plane). The terms ablation length and ablation diameter will be used to refer respectively to the long and short axis diameters of the coagulation zone. In some experimental trials the elongation of the thermal lesion beyond the antenna’s tip (A) was also measured.
Figure 3. Section along the transversal plane (orthogonal to the antenna’s axis) of an ablated sample of tissue. Cylindrical symmetry is apparent.
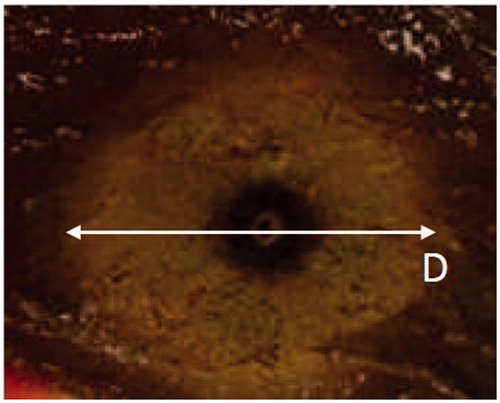
To characterise the influence of the sample dimension on the achievable thermal lesion, unrestricted samples of tissue were used first, in order to evaluate the maximum achievable dimensions of the zone of ablation for the considered power and time settings. Then, tissue samples with height (H as depicted in ) lower than the diameter of the thermal lesion evaluated in unrestricted samples were used (thin samples). Moreover, further measurements were conducted considering samples of tissue with both height (H) and width (W) lower than the transversal dimension of the thermal lesion in unrestricted samples (double-thin samples). This last arrangement is close to the simulated one, since the cylindrical symmetry leads to a circular shape of the numerical sample (target tissue) in the transversal plane. Thin and double-thin samples were ablated and cross-sectioned in the same way as unrestricted samples, the antenna being inserted along the longest axis of the sample. Each ablation was repeated four times at each laboratory.
Numerical simulations
In the simulations the thermal lesion size was calculated taking into consideration phantoms whose dimensions were greater than, smaller than, and close to those of the thermal lesion measured in unrestricted phantoms. With reference to the thermal boundary condition between the ex vivo liver and the surrounding air (Equation Equation2(2) ), since during the experimental studies a leakage of gases was observed, in the numerical simulations a convective exchange between overheated water vapour and air was modelled. In the literature, convective coefficient values between 5 and 50 W/(m2 °C) are assigned to air free convection, convective coefficient values between 30 and 300 W/(m2 °C) to air forced convection, while values in the range 3000–30 000 W/(m2 °C) to water vapour convection [Citation36,Citation37]. Accordingly, since no measurements of the actual value of the convective coefficient were performed during the experimental study, a convective coefficient equal to 300 W/(m2 °C) was used as a reference for representing the gas leakage [Citation36], while, to point out the influence of the considered boundary condition, further simulations were carried out using convective coefficient values in the range 20–3000 W/(m2 °C).
In a clinical scenario the tissue in which the antenna is inserted could be surrounded by air (e.g. in laparoscopic surgery) or by other tissues/organs (e.g. sub-capsular hepatic lesions). Accordingly, a numerical simulation was carried out to look for possible differences in the results obtained by considering fat around the liver instead of air. gives the dielectric and thermal properties of fat.
Results
Experimental ex vivo studies
reports the results obtained considering unrestricted tissue samples, thin samples, and double-thin samples, respectively, in terms of net power (Pnet) fed to the antenna in each trial and thermal lesion length (L), diameter (D), and elongation beyond the antenna tip (A). In the case of thin samples, D gives the diameter of the thermal lesion in the direction not limited by the sample height. In the trials numbered E-1 to E-4 refer to trials performed at ENEA, while H-1 to H-4 refer to trials performed at HS. At ENEA, the mean height of the thin samples was 24 mm, range 20–30 mm; the mean height and width of the double-thin samples was 28 mm, range 25–30 mm. In the HS trials, the mean height of the thin samples was 23 mm, range 22–24 mm; while the mean height and width of the double-thin samples was 30 mm, range 28–32 mm.
Table 2. Thermal lesion dimensions from ENEA (E) and HS (H) experiments carried out in unrestricted, thin and double-thin liver samples.
compares the length of the ablated zone measured by the two laboratories in unrestricted, thin, and double-thin liver samples, represented in terms of average and range of variation (minimum–maximum values of measured data). In the figure the p-values evaluated by the Student’s t-test on the different series of data (i.e. unrestricted, thin and double-thin samples) are also reported.
Figure 4. Range of variation (minimum–maximum values) of the length of the ablated zone obtained in unrestricted, thin, double-thin tissue samples in two laboratories. The p values of the t-test are also reported (95% CI).
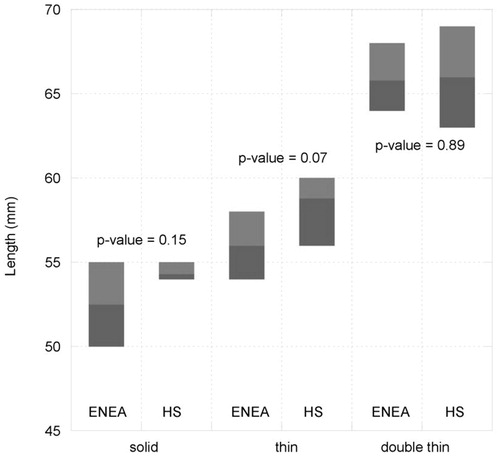
Since the p-values are always higher than 0.05 (assuming a 95% confidence interval (CI)) it can be inferred that for each set of data there are no statistically significant differences in the results achieved by the two laboratories. This outcome allows the claim of repeatability and reproducibility of the results obtained, in terms of the lengthening of the ablated area in thin phantoms, and the merging of the sets of data from the two laboratories. Accordingly, aggregating the eight experimental trials, the mean length of the ablated area and the corresponding standard deviation can be evaluated, as reported in the last two rows of . It should be noted here that data measured in four trials out of eight do not provide statistical power to evaluate a standard deviation; accordingly, the range (minimum–maximum) is more suitable to represent the dispersion of the experimental data.
As a whole, an elongation of the coagulated area of 7.5% (standard deviation (SD) 3.1%) is achieved in thin samples with respect to the unrestricted tissue, whereas an elongation of 23.5% (SD 6.1%) is achieved in double-thin samples. Moreover, from it can be inferred that the diameter of the zone of ablation evaluated in thin samples (mean value 43.5 mm) is close to the value obtained in unrestricted tissue models (mean value 42.9 mm), showing that the transversal expansion of the coagulation is not significantly affected by the sample dimension in the other transversal direction. Finally, considering the elongation beyond the antenna’s tip (A), mean values of 10.3 mm, 13.5 mm and 13.0 mm were measured in unrestricted, thin, and double-thin samples, respectively.
shows a photo of the thermal lesion obtained in a case of a double-thin sample of tissue. In the photo the position of the antenna is clearly visible (the cylindrical groove), as well as the arrowhead-shaped central zone of necrosis where tissue charring occurred, shown by a arrow.
Numerical study
Simulations were performed for two cylindrical liver tissue models 100 mm long and with a diameter of 100 mm (unrestricted sample) and 30 mm (in analogy with the experiments, this will be a double-thin sample). In all cases, the tissue model was surrounded by air.
shows the distribution of the specific absorption rate (SAR), defined as the power absorbed in the tissue per unit mass (W/kg) normalised to 1 W of power radiated by the antenna, in the unrestricted (), and in the double-thin sample () in the plane represented in . For symmetry and clarity the horizontal axis of both and extends up to 50 mm.
Figure 6. Bi-dimensional distribution in cylindrical coordinates of the SAR computed in unrestricted (A) and double-thin (B) samples. The white dotted line in (B) shows the boundary between liver and air; the arrows highlight the standing wave pattern of the absorbed power, pointing to its local maximum (white arrow) and minimum (grey arrow) values.
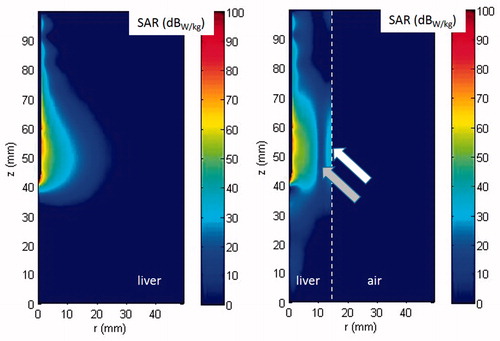
From the figure it can be noted that when the antenna is inserted in the unrestricted tissue model, the power absorption is well confined close to the antenna feed. On the contrary, in the double-thin tissue model, the absorbed power elongates longitudinally beyond the antenna tip and behind the antenna feed. Moreover, standing waves can be seen due to reflections of the EM field at the boundary between air and liver tissue, as shown by the two arrows in .
shows the distribution of the temperature computed after 10 min with the antenna radiating 60 W in two cases of unrestricted () and double-thin samples () for a convection coefficient representing the thermal boundary condition between liver and air equal to 300 W/m2K. The iso-lines reported in the figure show the boundary of the regions where the temperature is higher than 60 °C and 100 °C, corresponding to the numerically evaluated thermal lesion and to the numerical limit of the carbonised central region, respectively.
Figure 7. Bi-dimensional distribution of the temperature increase obtained radiating 60 W for 10 min in unrestricted (A) and double-thin samples (B). The white dotted line in (B) shows the boundary between liver and air.
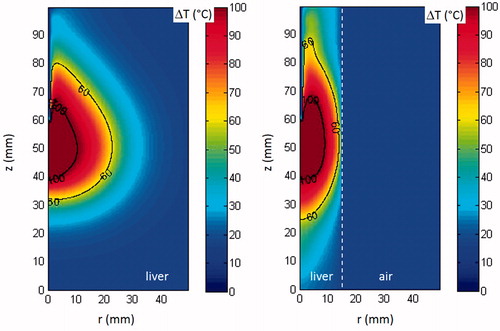
In the deformation of the coagulated area in the double-thin sample is clearly visible. In particular, close to the antenna’s cooling system (z values greater than 60 mm), the irregular shape results from the opposite action of the heating due to the absorption of the EM field () and of the cooling due to the presence of the antenna’s refrigerating system. From it can also be noted that, still in correspondence of the antenna’s cooling system, the tissues abutting the antenna’s shaft remain significantly colder than the outer neighbouring region, where the cooling system influence is lower. This result is in agreement with what was observed in .
The thermal lesion dimensions (length and diameter) as a function of time for both the unrestricted and double-thin tissue model are reported in . At the beginning of the ablation process the length and diameter of the thermal lesion were the same in the two samples considered; at about 2 min (120 s), when the thermal lesion diameter approached the width of the double-thin sample (28 mm), the ablation length in this tissue model began to increase faster than the corresponding data in the unrestricted sample, giving rise to the longitudinal elongation observed.
Further simulations were performed considering different values of the convective coefficient, as well as sample dimensions of 100 mm (longitudinal) × 50 mm (radial, slightly greater than the diameter of the thermal lesion in unrestricted samples). shows the results obtained.
Table 3. Thermal lesion dimension numerically evaluated for different tissue model dimensions and using different convective boundary conditions.
As can be seen from , in the unrestricted tissue model (radial dimension 100 mm) the value of the convective coefficient does not affect the thermal lesion dimension. On the contrary, in the double-thin sample (radial dimension 30 mm), low values of the convection coefficient, which do not allow a rapid heat exchange between the heated tissue and the surrounding air, lead to very long thermal lesions, whereas higher values of the convection coefficient lead to shorter thermal lesion lengths. Moreover, when the highest values of the convective coefficient are considered, the thermal lesion radial extension does not reach the actual margin of the sample tissue. Finally, when sample dimensions close to those of the expected thermal lesion are considered (diameter 50 mm), changing the convective coefficient by two orders of magnitude leads to little change (less than 3%) in the length of the thermal lesion, while the radial extension is greatly influenced.
If we compare the experimental lengthening of the coagulated zone (mean and standard deviation, referred to the eight trials aggregated) with the numerical elongation evaluated for the double-thin sample with respect to the unrestricted tissue model (range of values obtained by the simulations considering the convection coefficient between 300 W/m2 K and to 1000 W/m2 K), we find that the experimental and the numerical approaches lead to compatible conclusions. Similarly, comparing the experimentally evaluated and the numerically calculated elongation of the zone of ablation beyond the antenna’s tip, represented as the range of values from the different experimental trials and from the different simulated boundary conditions, good agreement between experimental and numerical data can be inferred, though experimental data exhibit larger variation with respect to numerical results.
Finally, if fat instead of air is considered around the thin liver – so that the convective boundary is changed in a thermal discontinuity between two tissues with different thermal properties (see ) – a thermally ablated area of 37.0 mm × 63.5 mm is obtained after 10 min with the antenna radiating 60 W. Accordingly, the radial extension is slightly greater than the case of air surrounding the liver (i.e. the thermal lesion partially extends into the fat layer), and the lesion length is close to that obtained in the thin liver surrounded by air (i.e. 62.4 mm).
Discussion
The success of thermal ablation treatments is strictly linked to the ability of correctly predicting the shape and dimension of the achievable thermal lesion when developing ablation protocols, in order to improve the treatment planning.
In this study, unrestricted samples of tissues (with dimensions sufficiently large to contain the thermal lesion), thin samples (whose height is lower than the ablation diameter of unrestricted samples), as well as double-thin samples (whose height and width are both lower than the ablation diameter of unrestricted samples) were experimentally employed to characterise the thermal lesion achieved by a minimally invasive microwave antenna radiating 60 W at 2.45 GHz for 10 min.
The results obtained show that the size and shape of the target tissue may affect the treatment outcome. In particular, the experiments showed that in thin tissue samples the coagulated area has a greater length along the antenna’s axis with respect to that obtained in unrestricted tissue models. These findings proved to be repeatable in two laboratories (p-values always higher than 0.05 (95% CI) – – with an average increase of the lesion length of about 7.5% (SD 3.1%) with respect to the unrestricted tissue model when the height of the tissue was reduced (thin sample), and an average increase of 23.5% (SD 6.1%) when both the height and width of the sample tissue were reduced (double-thin sample). It is worth noting that the ranges of the length of the thermal lesion obtained in unrestricted samples (mean 53.4 mm, SD 1.7 mm) and thin samples (mean 57.4 mm, SD 2.2 mm) are very close, whereas the elongation becomes statistically significant when double-thin samples are considered (mean 65.9 mm, SD 2.2 mm). Moreover, the transversal dimension of the zone of ablation (in the direction not limited by the tissue sample) was very close in thin and unrestricted samples (mean value: 43.5 mm vs. 42.9 mm), evidencing a shape of the thermal lesion not significantly affected by the asymmetry of the tissue.
The numerical study looked at the parameters influencing the ablation process, with particular reference to the thermal boundary condition between the sample tissue and the surrounding environment. In the numerical study, a length of the coagulated area of 48.2 mm was obtained in the unrestricted tissue model whatever thermal boundary condition was used, whereas it increased in the double-thin tissue model, with absolute values which depended on the thermal boundary condition used. Considering a convective coefficient of 300 W/m2 °C as a reference, a length of 62.4 mm was obtained in the double-thin sample, with an increase of 29.5% with respect to the unrestricted one. Comparing the numerical results with the experimental data, the numerical study finds a lower length of the coagulated area, both in the case of unrestricted and in the case of double-thin phantoms. However, the relative lengthening of the coagulated zone is close to the value experimentally obtained. Numerical uncertainties, which could explain the lower length, are linked to the values assigned to the dielectric and thermal properties of the tissue, to the model of the cooling system, and to the temperature threshold used to define the zone of ablation (here assumed to be 60 °C).
The numerical study allowed clarification of the cause of the increase of the ablation length in the double-thin phantom. At the tissue–air interface, the EM field is reflected in the tissue, causing a refocusing along the antenna axis, both in the proximal and distal directions. This different distribution of the EM power deposited with respect to the unrestricted tissue model gives rise to the inclusion of points in the thermal lesion beyond the antenna tip as well as behind the antenna feed. In particular, behind the antenna feed the shape of the thermal ablated area is deformed with respect to the case of unrestricted phantom, due to the opposite action of the EM power absorption and of the antenna’s cooling system. Beyond the antenna tip, the elongation obtained is due to the different distribution of the EM power absorption.
In the numerical study it became evident that the boundary condition between the liver tissue and the air has a great influence on the results achieved. In particular, the reported data have been obtained while considering a convective exchange between overheated water vapour and the air in order to represent the leakage of gases observed during experimental studies. If a slower heat exchange is considered a longer thermal lesion is obtained, whereas if a faster exchange is considered a shorter coagulated area is obtained. Accordingly, it can be concluded that the dimension of the ablated zone is greatly influenced both by the shape and size of the target tissue and by the thermal properties of the medium surrounding the tissue in which the microwave thermal ablation is accomplished.
A further numerical study, conducted for a phantom whose radial dimensions were close to those of the thermal lesion diameter in the unrestricted phantom, found an ablated area length close to that obtained in the unrestricted phantom, showing that the thermal lesion shape is not affected provided the tissue sample dimensions are, even only just, sufficient to include the ablated area. Finally, the presence of fat instead of air around the thin liver, gave results very close to those obtained in the reference situation.
The results achieved have great implications in the definition of clinical protocols for thermal ablation therapies. In fact, the interventional protocols are usually derived through in vivo experiments conducted on porcine livers; however, porcine liver has lobes with a lower height than human liver, so this biological target may resemble the thin phantom used in the present study. Accordingly, ablations obtained in human liver may differ from predictions based on porcine liver models. Moreover, if the radiated power and/or the time of irradiation are such to obtain a thermal lesion larger than the thickness of the target liver portion, a zone of ablation longer than planned could be obtained, which might influence the outcome of the treatment. Similar consequences are expected when considering thermal ablation procedures near the surface of a tissue or organ. As a consequence, the results obtained from this work could account for different coagulative performances obtained in intra-surgical treatments when compared to deep percutaneous ablations carried out with the same equipment and similar protocols.
Further experiments are needed to confirm these outcomes, both increasing the number of trials and considering different tissues and geometrical conditions, as porcine liver, for example, and procedures with the interstitial antenna placed close to the boundary of the tissue. Finally, in vivo studies should be performed to show how blood perfusion influences the data obtained.
Conclusions
This work investigated the influence of the dimensions of tissues on the size and shape of the coagulated zone achievable from microwave thermal ablation procedures. The study was conducted both through ex vivo experiments and numerically.
The results obtained show that, when the target tissue is thinner than the ablation diameter in large tissues, the ablation length increases with respect to the unrestricted sample case. The amount of the elongation is strongly dependent on the thermal boundary condition between the target tissue and the surrounding tissue.
The findings of this study highlight potential pitfalls in clinical treatment planning when using unrestricted tissue models as a guideline regardless of the specific target geometry and the surrounding environment.
Acknowledgements
The authors acknowledge Sergio Mancini (ENEA) for his technical support essential to accomplish the experimental work, and S. Nahum Goldberg (Hadassah, Hebrew University, Jerusalem, Israel and Beth Israel Deaconess Medical Center, Boston, MA, USA) for many useful discussions.
Declaration of interest
Nevio Tosoratti, Claudio Amabile and Simone Cassarino are employees of HS Hospital Service SpA, which supported the study through the provision of the MW antennas and generator. The authors alone are responsible for the content and writing of the paper.
References
- Dewhirst MW, Vigilanti BL, Lora-Michiels M, Hanson M, Hoopes PJ. Basic principles of thermal dosimetry and thermal thresholds for tissue damage from hyperthermia. Int J Hyperthermia 2003;19:267–94
- Skinner MG, Lizukay MN, Koliosz MC, Sherary MD. A theoretical comparison of energy sources – microwave, ultrasound and laser – for interstitial thermal therapy. Phys Med Biol 1998;43:3535–47
- Goldberg SN, Gazelle GS, Mueller PR. Thermal ablation therapy for focal malignancy: A unified approach to underlying principles, techniques, and diagnostic imaging guidance. Am J Roentgenol 2000;174:323–31
- Ahmed M, Brace CL, Lee Jr FT, Goldberg SN. Principles of and advances in percutaneous ablation. Radiology 2011;258:351–69
- Zhang H, Nan Q, Liu Y. Thermal distribution of microwave antenna for atrial fibrillation catheter ablation. Int J Hyperthermia 2013;29:582–9
- Hodgson DA, Feldberg IB, Sharp N, Cronin SN, Evans M, Hirschowitz L. Microwave endometrial ablation: Development, clinical trials and outcomes at three years. Br J Obstet Gynaecol 1999;106:684–94
- Haemmerich D, Laeseke PF. Thermal tumour ablation: Devices, clinical applications and future directions. Int J Hyperthermia 2005;21:755–60
- Livraghi T, Meloni F, Solbiati L, Zanus G. Complications of microwave ablation for liver tumors: Results of a multicenter study. Cardiovasc Intervent Radiol 2012;35:868–74
- Li M, Yu X, Liang P, Liu F, Dong B, Zhou P. Percutaneous microwave ablation for liver cancer adjacent to the diaphragm. Int J Hyperthermia 2012;28:218–26
- Callstrom MR, Charboneau JW. Technologies for ablation of hepatocellular carcinoma. Gastroenterology 2008;134:1831–5
- Jones C, Badger SA, Ellis G. The role of microwave ablation in the management of hepatic colorectal metastases. Surgeon 2011;9:33–7
- Wasser EJ, Dupuy DE. Microwave ablation in the treatment of primary lung tumors. Semin Respir Crit Care Med 2008;29:384–94
- Liu F, Liang P, Yu X, Lu T, Cheng Z, Lei C, et al. A three-dimensional visualisation preoperative treatment planning system in microwave ablation for liver cancer: A preliminary clinical application. Int J Hyperthermia 2013;29:671–7
- Chiang J, Wang P, Brace CL. Computational modelling of microwave tumour ablations. Int J Hyperthermia 2013;29:308–17
- Hoffmann R, Rempp H, Erhard L, Blumenstock G, Pereira PL, Claussen CD, et al. Comparison of four microwave ablation devices: An experimental study in ex vivo bovine liver. Radiology 2013;268:89–97
- Brace CL, Laeseke PF, Sampson LA, Frey TM, van der Weide DW, Lee FT Jr. Microwave ablation with a single small-gauge triaxial antenna: In vivo porcine liver model. Radiology 2007;242:435–40
- Pennes HH. Analysis of tissue and arterial blood temperatures in resting forearm. J Appl Physiol 1948;1:93–122
- Wissler EH. Pennes’ 1948 paper revisited. J Appl Phys 1998;85:35–41
- Simo KA, Tsirline VB, Sindram D, McMillan MT, Thompson KJ, Swan RZ, et al. Microwave ablation using 915-MHz and 2.45-GHz systems: What are the differences? HPB 2013;15:991–6
- Sommer CM, Koch V, Pap B, Bellemann N, Holzschuh M, Gehrig T, et al. Effect of tissue perfusion on microwave ablation: Experimental in vivo study in porcine kidneys. J Vasc Interv Radiol 2011;22:1751–7
- Lin JC, Bernardi P, Pisa S, Cavagnaro M, Piuzzi E. Antennas for medical therapy and diagnostics. In: Balanis C, editors. Modern antenna handbook. New York: Wiley; 2008. pp 1377–428
- Bertram JM, Yang D, Converse MC, Webster JG, Mahvi DM. A review of coaxial-based interstitial antennas for hepatic microwave ablation. Crit Rev Biomed Eng 2006;34:187–213
- Cavagnaro M, Amabile C, Bernardi P, Pisa S, Tosoratti N. A minimally invasive antenna for microwave ablation therapies: Design, performances, and experimental assessment. IEEE Trans Biomed Eng 2011;58:949–59
- Longo I. C.N.R. Industrial Patent N. PI/2001/A/000 006. 2001
- Pisa S, Cavagnaro M, Bernardi P, Lin JC. A 915-MHz antenna for microwave thermal ablation treatment: Physical design, computer modeling and experimental measurement. IEEE Trans Biomed Eng 2001;48:599–601
- Bernardi P, Cavagnaro M, Lin JC, Pisa S, Piuzzi E. Distribution of SAR and temperature elevation induced in a phantom by a microwave cardiac ablation catheter. IEEE Trans Microw Theory Techn 2004;52:1978–86
- Bernardi P, Cavagnaro M, Pisa S, Piuzzi E. A graded-mesh FDTD code for the study of human exposure to cellular phones equipped with helical antennas. ACES J 2001;16:90–96
- Lopresto V, Pinto R, Lodato R, Lovisolo GA, Cavagnaro M. Design and realisation of tissue-equivalent dielectric simulators for dosimetric studies on microwave antennas for interstitial ablation. Phys Med 2012;28:245–53
- Cavagnaro M, Franco S, Lopresto V, Pinto R. Characterization of a microwave thermal ablation process. Proc XIX Riunione Nazionale di Elettromagnetismo, Rome, Italy, 10–14 September 2012, pp. 661–4
- Ji Z, Brace CL. Expanded modeling of temperature-dependent dielectric properties for microwave thermal ablation. Phys Med Biol 2011;56:5249–64
- Lopresto V, Pinto R, Lovisolo GA, Cavagnaro M. Changes in the dielectric properties of ex vivo bovine liver during microwave thermal ablation at 2.45 GHz. Phys Med Biol 2012;57:2309–27
- Lopresto V, Pinto R, Cavagnaro M. Experimental characterisation of the thermal lesion induced by microwave ablation. Int J Hyperthermia 2014;30:110–18
- Yang D, Converse MC, Mahvi DM, Webster JG. Expanding the bioheat equation to include tissue internal water evaporation during heating. IEEE Trans Biomed Eng 2007;54:1382–88
- Cavagnaro M, Lopresto V, Pinto R. On the modelling of the temperature increase obtained in a microwave thermal ablation process. Paper presented at the BioEM2013 Joint Meeting of the Bioelectromagnetics Society and the European BioElectromagnetics Association, Thessaloniki, Greece, 10–14 June 2013
- Cavagnaro M, Pinto R, Lopresto V. Numerical models of microwave thermal ablation procedures. Proc 44th European Microwave Conference, Rome, Italy, 5–10 October 2014
- Welty JR, Wicks CE, Wilson RE, Rorrer GL. Fundamentals of momentum, heat, and mass transfer. 5th ed. New York: Wiley; 2008. p 208
- Rohsenow WM, Hartnett JP, Ganić EN, editors. Handbook of heat transfer fundamentals. New York: McGraw-Hill; 1985