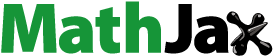
Abstract
Purpose: In this study the effect of PLGA polymeric nanoparticles as a 5-fluorouracil (5-FU) carrier with and without iron oxide core and hyperthermia were investigated on the level of DNA damage in a spheroid culture model of HT-29 colon cancer cell lines by alkaline comet assay.
Materials and methods: First, HT-29 colon cancer cells were cultured in vitro as spheroids with a mean diameter of 100 µm. The spheroids were then treated with different concentrations of 5-FU or nanoparticles as 5-FU carriers with and without an iron oxide core for one volume-doubling time of the spheroids (71 h) and hyperthermia at 43 °C for 1 h. Finally, the effect of treatment on viability and level of DNA damage was examined using trypan blue dye exclusion assay and alkaline comet assay, respectively.
Results: Results showed that hyperthermia in combination with 5-FU or nanoparticles as 5-FU carriers significantly induced the most DNA damage as compared with the control group. The extent of DNA damage following treatment with 5-FU-loaded nanoparticles combined with hyperthermia was significantly more than for 5-FU combined with hyperthermia. In comparison to the effect of 5-FU-loaded nanoparticles with the iron oxide core and 5-FU-loaded nanoparticle without the iron oxide core, the nanoparticles with the iron oxide core combined with hyperthermia induced more DNA damage than the nanoparticles without the iron oxide core.
Conclusions: According to this study, hyperthermia is a harmful agent and nanoparticles are effective delivery vehicles for drugs into colon cancer cells. The iron oxide filled nanoparticles increased the effect of the hyperthermia. All these factors have a significant role in the treatment of colorectal cancer cells.
Introduction
Colon cancer is the third most prevalent cancer and the fourth most common cause of death in both men and women worldwide. Several risk factors, including genetics, age, personal history of adenomatous polyps, nutritional practices and smoking are associated with this cancer [Citation1]. Some of the most common treatment methods include surgery, chemotherapy, radiotherapy and, in some countries, hyperthermia [Citation2]. Hyperthermia alone or in combination with other modalities such as chemotherapy and radiotherapy is a well-established method for treating cancer [Citation3]. The cell-killing potential of some but not all chemotherapeutic agents is substantially enhanced by temperature elevation of even a few degrees. Maeta et al. [Citation4] showed temperature-mediated elevation in the intracellular levels of 5-fluorouracil (5-FU) in Ehrlich and sarcoma-180 tumour cells incubated with 5-FU. They observed that levels of 5-fluorouridine (FUR) and 5-fluoro-2′-deoxyuridine (FdUR), the active metabolites of 5-FU, significantly increased in cells treated with 5-FU and hyperthermia [Citation4].
5-FU, a pyrimidine analogue, is used alone or in combination with other modalities to treat solid tumours such as tumours of the gastrointestinal tract, pancreas, ovaries, liver, brain and breasts. 5-FU is an S-phase-active agent that is not active in other phases of the cell cycle. The resulting position of cells in the cell cycle plays an important role in response to drug therapy [Citation5]. Because of its structure, 5-FU interferes with nucleoside metabolism, converting to 5-fluorouridine triphosphate and 5-fluorodeoxyuridine monophosphate in the cell and incorporating into RNA and DNA. This condition leads to further genetic damage, particularly single- and double-strand breaks in DNA, and, consequently, cell death [Citation6,Citation7].
However, 5-FU is associated with some disadvantages [Citation6]. First, 5-FU is a hydrophilic drug and cannot pass through the cell membrane. Second, it decomposes within the body and results in severe side effects. Third, it undergoes rapid degradation and is required at high doses. Nanoparticles can be used to overcome these limitations. However, the nanoparticles used must be biocompatible and biodegradable. Poly lactic-co-glycolic acid (PLGA) is just such polymer that has no toxic effects and is capable of controlling the drug release from nanoparticles [Citation8,Citation9]. PLGA is degraded by hydrolysis into acid monomers and excreted from the body by the Krebs cycle [Citation10]. Because of their small size and electromagnetic properties, nanoparticles can be useful [Citation11].
Research suggests that chemotherapy in combination with hyperthermia is more effective than chemotherapy alone [Citation12]. Hyperthermia occurs when the body temperature increases to more than 41 °C because of external factors. In this method, which is non-ionising, the probability of treatment increases by approximately 20–30%, causing an increase in blood flow, oxygen, drug delivery and sensitivity of tumours [Citation12,Citation13]. Hyperthermia sensitises tumour cells to drugs and nanoparticles containing the iron oxide core because hyperthermia eliminates drug resistance and heats iron oxide, thereby intensifying the effect of hyperthermia and increasing drug release [Citation14,Citation15].
In this study we have used PLGA-coated nanoparticles as 5-FU carriers with and without the iron oxide core to determine role of the iron oxide core in thermosensitisation of the cells. In this nanostructure, 5-FU as a thermosensitiser drug can load in PLGA as a degradable polymer. PLGA-coated nanoparticles can facilitate the penetration of 5-FU into cells. We therefore examined DNA damage induced by PLGA-coated 5-FU-loaded nanoparticles with or without the iron oxide core in combination with hyperthermia (43 °C for 1 h) in the spheroid model of HT-29 colon cancer cells. Cultured HT-29 cells can grow as a monolayer or as spheroid cultures [Citation16,Citation17]. Multicellular spheroids provide more information on basic tumour biology and respond to different therapeutic agents [Citation18].
Materials and methods
Cell line
Human colon cancer cell line HT-29 was obtained from the Pasteur Institute of Iran, Tehran. The cell line was maintained in RPMI 1640 (Gibco, Grand Island, NY) supplemented with 10% fetal bovine serum (FBS) (PAA, PAA Laboratories Ply Ltd, Australia), 100 µ/mL of penicillin and 100 mg/mL of streptomycin (Sigma).
Monolayer culture
HT-29 cells were cultured as a monolayer at a density of 104 cells/cm2 in T-25 tissue culture flasks (Orange Scientific, Braine-l'Alleud, Belgium). The cultures were maintained at 37 °C in a humidified atmosphere of 5% CO2 Cells were harvested by trypsinising cultures with 1 mM EDTA/0.25% trypsin (w/v) in phosphate buffer saline (PBS).
Spheroid culture
Spheroids were cultured using the liquid overlay technique: 5 × 105 HT-29 cells were seeded into 100-mm culture Petri dishes coated with a thin layer of 1% agar (MERC) with 10 mL of RPMI supplemented with 10% FBS. The plates were incubated at 37 °C in a humidified atmosphere and 5% CO2. Half of the culture medium was replaced with fresh culture medium twice per week.
Synthesis of nanoparticles
Synthesis and characterisation of 5-FU- loaded PLGA-coated nanoparticles with/without iron oxide were based on the method of Dr Khoee et al. [Citation19]. These biodegradable 5-FU-loaded nanoparticles with an iron oxide core are synthesised through modified multiple emulsions–solvent evaporation methods oil in water, oil in water (o/w/o/w) [Citation19]. For preparation of nanoparticles, the first oil-based magnetite nanoparticles were dispersed in dichloromethane (DCM) to prepare a primary organic phase (O1) Then, the inner aqueous solution was prepared by dissolving 5-FU and Tween 60 in double distilled water (W1). The magnetic dispersion (O1) was emulsified in the inner aqueous solution (W1) by ultrasonication to obtain an O1/W1 emulsion (dispersion of magnetite). This primary emulsion was emulsified in an organic solution (O2) of the polymer (PLGA and Span 60 in DCM) by ultrasonication to obtain an O1/W1/O2 double emulsion. Next, this double emulsion was immediately poured into W2 aqueous solution, which was made of Tween 60 dissolved in distilled water and glycerine, and the mixture was ultrasonicated again. The resulting multiple emulsion (O1/W1/O2/W2) was diluted in aqueous solution under mechanical stirring, and the DCM was removed by solvent evaporation. Finally, the nanoparticles obtained were dried by freeze-drying and stored at 4 °C. Additionally, PLGA nanoparticles without drug were prepared in the same way, except that the inner aqueous solution was prepared with the same amount of distilled water and Tween 60, but without 5-FU. 5-FU-loaded nanoparticles without iron oxide core were synthesised through double emulsions (w/o/w) [Citation20]. Drug-free nanoparticles without iron oxide core were synthesised through emulsion inversion (o/w) [Citation21].
Characterisation of nanoparticles
Particle size determination
The mean diameter and size distribution of the aqueous dispersion of nanoparticles were measured by Dynamic Light Scatterer (DLS) (Brookhaven Instruments, Holtsville, NY, USA). The sample was diluted to an appropriate concentration with deionised water, which was filtered previously with a 0.22 µm Millipore filter, to avoid any contamination, and then sonicated for 10 min and finally the size distribution of nanoparticles was measured by DLS.
Zeta potential
Zeta potential is an indicator of surface charge, which determines particle stability in dispersion. Zeta potential of nanoparticles was determined by a zeta potential analyser. The nanoparticle was suspended in distilled water and sonicated for 10 min.
Morphology of nanoparticles
The morphological investigation of nanoparticles was performed using a Zeiss LEO906 (Jena, Germany) transmission electron microscope (TEM). The nanoparticle was suspended in distilled water and sonicated for 10 min. A drop of nanoparticle suspension was placed on a carbon-coated grid. The grid was perfectly dried and observed under the TEM at 100 KeV.
Determination of drug content and encapsulation efficiency
To evaluate the drug concentration in a nanoparticle, a UV absorption measurement was performed. The 5-FU concentration was calculated by application of Beer’s law. First, the nanoparticles were weighed and re-dissolved in acetone (spectroscopy grade). Next, the insoluble magnetite particles were removed from the solution by magnetic separation. Finally, the 5-FU concentration in the acetone solution was determined by UV absorption at a wavelength of 201 nm (characteristic absorption band of 5-FU) with respect to a previously established calibration curve, and the amount of encapsulated drug in the nanoparticle was calculated. Drug content and encapsulation efficiency were obtained using Equations Equation1(1)
(1) and Equation2
(2)
(2) , respectively:
(1)
(1)
(2)
(2)
In vitro drug release
The in vitro 5-FU release from PLGA nanoparticles was performed in PBS medium using the dialysis method. The dialysis bag (molecular weight cut-off 12,400 Da) was soaked in preheated at 100 degree of centigrade double-distilled water before use. A weighed amount of freeze-dried PLGA nanoparticle samples was suspended in PBS and then transferred into a dialysis bag. Both ends of the bag were fixed with clamps. The bag was placed into 100 mL of preheated at 37 degree of centigrade PBS as a release medium. The release study was performed in an incubator shaker at 37 °C. At selected time intervals, the solution outside the dialysis bag was removed (n = 3) for UV visible analysis and replaced with fresh buffer solution. At fixed time intervals (e.g. 4 days) the medium in the vessel was completely removed and replaced with fresh release medium. The 5-FU concentration was calculated based on the absorbance intensity of 5-FU at 266 nm. In the assessment of drug release behaviour, the cumulative amount of released drug was calculated and the percentages of released drug from PLGA nanoparticles were plotted against time. All experiments were carried out in triplicate.
Cellular uptake of nanoparticles
After preparing spheroids with mean diameter 100 µm, they were treated with nanoparticles for 71 h, were washed by phosphate buffer, fixed with 2.5% glutaraldehyde for 1:30 h at 4 °C, and washed three times in phosphate buffer 0.1%. After that, the cells were stained in 1% osmium tetroxide for 1 hour, washed with phosphate buffer 3 times, then dehydration was carried out with varying degrees of acetone (50%, 70%, 80%, 90%, and 100%). Then samples were moulded in resin and a polymerisation procedure was carried out at 60 °C for 48 h. Sections 30–50 nm were then prepared under stereomicroscope and placed on a carbon-coated grid. Finally, after staining with lead citrate and ammonium acetate, images confirming the entry of nanoparticles into the cell were observed using a Zeiss LEO906 TEM.
Hyperthermia and 5-FU (-free or -loaded PLGA-coated nanoparticles with/without iron oxide core) treatment of spheroid culture
Cells were cultured for multicellular spheroid formation. On day 10, spheroids with mean diameter of 100 µm were treated with 1, 5 or 10 µM of 5-FU (Sigma) [Citation22] or the same concentration of 5-FU released from 5-FU-loaded PLGA-coated nanoparticles with or without the iron oxide core for 71 h. PLGA nanoparticles were used as a control for the drug-loaded nanoparticles treatment. Before heat treatment, the medium was replaced with fresh RPMI 1640 culture medium. Hyperthermia was applied at 43 °C for 60 min in a precision water bath (Memmert, Schwabach, Germany) with ± 0.1 °C accuracy. Control cells were exposed to 37 °C. After heat treatment, the spheroid cells were treated with 300 µL of 1 mM EDTA/0.25% trypsin (w/v) in PBS for 5 min at 37 °C. The single cells were counted and tested for viability using a trypan blue dye exclusion assay. To evaluate the effect of treatments performed, single cell gel electrophoresis (alkaline comet assay) was used.
Trypan blue exclusion assay
A suspension of treated and control single cells from the spheroid culture were mixed with trypan blue at a 9:1 ratio. The resulting mixture was examined within 3–5 min under a light microscope and blue-coloured cells were considered dead. The ratio of unstained cells to total number of cells was reported as the viability percentage for each cell category.
Cell characteristics
The HT-29 colon cancer cell line grows as a monolayer on plastic culture flasks with a population doubling time of approximately 25 ± 0.39 h. The HT-29 cells are also able to form spheroids in liquid overlay cultures with a volume doubling time of approximately 71 ± 3.8 h, which was applied as drug treatment time consequently.
Alkaline comet assay
DNA fragmentation was assessed using single cell gel electrophoresis (Comet) assay, previously optimised by our group [Citation23]. Ordinary microscope slides were coated with 1% normal melting point agarose. Approximately 10,000 cells were suspended in 100 µL of 0.5% low melting point agarose. The cell suspension was rapidly pipetted onto the first agarose layer. The slides were allowed to solidify, then immersed in freshly prepared lysis buffer (2.5 M NaCl, 100 mM EDTA, 10 mM Tris-base with 1% Triton X-100, pH = 10) and incubated for 1 h. From that point on, all the steps were performed at 4 °C. The slides were removed from the lysis buffer and placed in a horizontal gel electrophoresis tank (CSLCOM20, Cleaver Scientific, Rugby, UK) which was filled with fresh cold denaturation buffer (300 mM NaOH, 1 mM EDTA, pH = 13). The slides were left in the solution for 30 min. Electrophoresis was conducted in the same denaturation buffer for 30 min using 1 V/cm voltage and a current of 300 mA. Following electrophoresis, the slides were washed in Tris buffer (0.4 M Tris HCl, pH = 7.5) to neutralise the excess alkali. Finally, the slides were stained with ethidium bromide (20 μg/mL).The individual cells or comets were viewed and photographed using a fluorescent microscope (Zeiss, Axioskop2 plus). The photographs were analysed by Comet Score® software. DNA damage was quantified as an increase in tail moment, the product of the amount of DNA (fluorescence) in the tail, and the distance between the means of the head and tail fluorescence distributions.
Statistical analysis
The results are expressed as mean values ± SEM (Standard Error Mean), with ‘n’ denoting the number of experiments. For statistical analysis, one-way ANOVA followed by Turkey’s test as the post-hoc analysis were performed using SPSS version 16. The value of p < 0.05 was considered to be statistically significant.
Results
Characterisation of nanoparticles
Particle size and zeta potential determination
The zeta potential and particle size was strongly influenced by the emulsifier used in the fabrication process of the nanoparticles. The particle size, polydispersity index and zeta potential of the four types of nanoparticles were measured by DLS and are shown in . The nanoparticles formulated in this study were found to be in the size range of 30–100 nm and stable in dispersion state, possessing high absolute values of zeta potential and having negative surface charges.
Table 1. Particle size and Zeta potential of nanoparticles.
Morphological studies
TEM measurement was carried out in order to characterise the morphology of the nanoparticles. shows the photographs of 5-FU-loaded nanoparticles with iron oxide core and shows the photographs free-drug nanoparticles without iron oxide core. It can be seen that most of the nanoparticles had a regular spherical shape, and at optimum conditions the particle size was below 100 nm. We verified that the nanoparticles had a uniform shape and size and were well enough dispersed.
Drug content and encapsulation efficiency of nanoparticles
The drug content and encapsulation efficiency of the nanoparticles with iron oxide core were 11.5% and 71.8% and for the nanoparticles without iron oxide core were 10.1% and 63.1% respectively.
In vitro release profile of 5-FU
shows the cumulative in vitro release profile of 5-FU from the nanoparticle with/without iron oxide core. It shows that the nanoparticle with iron oxide core was released to the extent of 16.5%, but the nanoparticle without iron oxide core was released to the extent of 14.3% within approximately 71 h in phosphate buffer at 37 °C.
Cellular uptake of nanoparticles
After slide preparation as stated in the previous chapter, the images confirming the entry of nanoparticles into the cell were prepared.
shows the TEM images of HT-29 spheroids without any nanoparticles as control samples and shows the uptake of the nanoparticles into HT-29 cells. The yellow arrows indicate some of the nanoparticles distributed in the cell.
Effects of 5-FU/5-FU-loaded PLGA-coated nanoparticles with/without iron oxide core and/or hyperthermia on viability
Immediately after cell treatment with 1, 5 and 10 µM of 5-FU or the same concentration of 5-FU released from 5-FU-loaded PLGA-coated nanoparticles with or without the iron oxide core for 71 h and hyperthermia, spheroid cells were dispersed to single cells. They were then counted and viability was determined using the trypan blue dye exclusion assay.
show the effect of 5-FU and 5-FU-loaded nanoparticles with and without iron oxide core and hyperthermia on the viability of HT-29 cells from spheroid cultures. As can be seen, hyperthermia at 43 °C in combination with 1, 5 and 10 µM 5-FU or the same concentration of 5-FU released from 5-FU-loaded PLGA-coated nanoparticles with or without the iron oxide core for 71 h did not have any effect on the viability of cells in spheroid culture (p > 0.05).
Figure 4. Effects of different concentrations of 5-FU and hyperthermia on (A) 5-FU-loaded PLGA-coated nanoparticles without iron oxide core, (B) 5-FU-loaded PLGA-coated nanoparticles with iron core with hyperthermia, and (C) the viability of HT-29 spheroid culture cells. The viability was measured using trypan blue dye exclusion assay method. Mean ± SEM of three experiments.
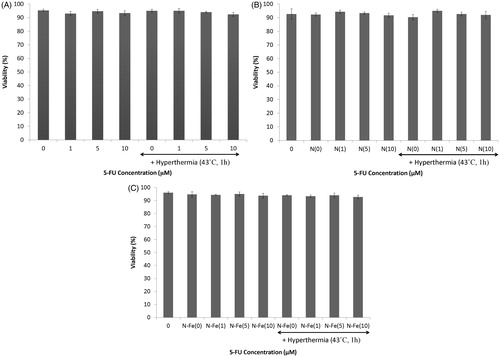
Effects of 5-FU/5-FU-loaded PLGA-coated nanoparticles with/without iron oxide core and/or hyperthermia on induced DNA damage
Viability of HT-29 cells was determined using trypan blue dye exclusion assay. Next, alkaline comet assay was performed to evaluate DNA damage. The average of tail moments in each category of cells was used as an indicator of DNA damage. shows the effect of 1, 5 and 10 µM 5-FU and the same concentration of 5-FU in combination with hyperthermia (43 °C for 1 h). Induction of DNA damage was not significantly different between control cells and cells treated with <10 µM 5-FU because of the low concentration of 5-FU. Induction of DNA damage in cells treated with 5-FU in combination with hyperthermia increased with an increase in the concentration of 5-FU. The extent of DNA damage in cells treated with 5-FU in combination with hyperthermia was significantly higher than that in cells treated with 5-FU alone (P < 0.05). This was because of the synergistic effect of the drug and hyperthermia. Notably, hyperthermia alone did not induce DNA damage in spheroid cultures of HT-29 cells.
Figure 5. Effects of different concentrations of 5-FU with and without hyperthermia on induced DNA damage of HT-29 spheroid culture cells. Mean ± SEM of three experiments.
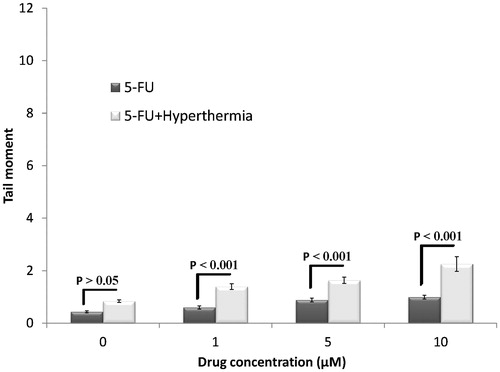
Next, we compared the DNA damage induced by 1, 5 and 10 µM 5-FU and that induced by the same concentrations of 5-FU released from 5-FU-loaded PLGA-coated nanoparticles with or without the iron oxide core for 71 h (). The extent of DNA damage induced by 5-FU released from PLGA nanoparticles with or without the iron oxide core was significantly higher than that by the same concentration of 5-FU (p < 0.001). Moreover, no significant difference was observed in the extent of DNA damage induced by the drug released from nanoparticles with the iron oxide core and that released from nanoparticles without the iron oxide core (p > 0.05). This result indicated that the iron oxide core had no effect on the induction of DNA damage.
Figure 6. Effects of different concentrations of 5-FU or 5-FU-loaded nanoparticles with and without iron core on induced DNA damage of HT-29 spheroid culture cells. Mean ± SEM of three experiments.
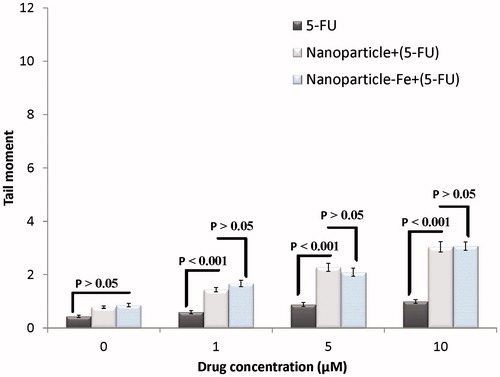
shows the effect of treatment with 1, 5 and 10 µM 5-FU released from 5-FU-loaded PLGA-coated nanoparticles without the iron oxide core and that released from 5-FU-loaded PLGA-coated nanoparticles without the iron oxide core in combination with hyperthermia for 71 h. Induction of DNA damage in HT-29 cells was higher with the combination treatment and increased with an increase in the concentration of 5-FU. However, the extent of DNA damage induced by the combination treatment of 5-FU-loaded nanoparticles without the iron oxide core and hyperthermia was significantly higher than that induced by 5-FU-loaded nanoparticles without the iron oxide core alone (P < 0.001). Moreover, free nanoparticles without the iron oxide core had no significant effect on the induction of DNA damage compared with control (p > 0.05).
Figure 7. Effects of 5-FU-loaded PLGA-coated iron-free nanoparticles with and without hyperthermia on induced DNA damages of HT-29 spheroid culture cells. Mean ± SEM of three experiments.
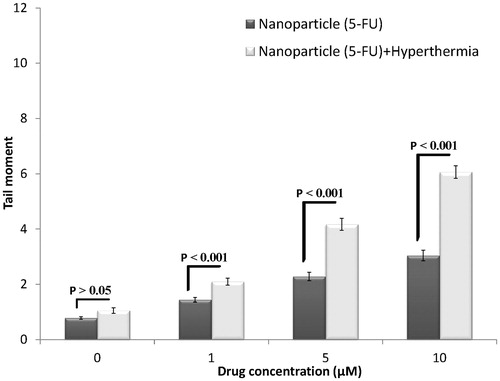
shows the effect of treatment with 1, 5 and 10 µM 5-FU released from 5-FU-loaded PLGA-coated nanoparticles with the iron oxide core and that released from 5-FU-loaded PLGA nanoparticles with the iron oxide core in combination with hyperthermia for 71 h. confirms the results in , with the exception that nanoparticles contain the iron oxide core in .
Figure 8. Effects of 5-FU-loaded PLGA-coated iron oxide nanoparticles with and without hyperthermia on induced DNA damage of HT-29 spheroid culture cells. Mean ± SEM of three experiments.
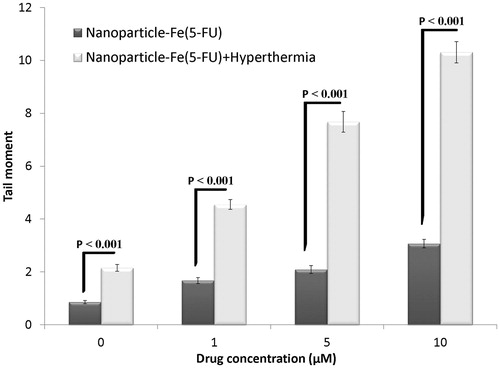
The tail moment of each group
The effects of 5-FU-loaded nanoparticles with the iron oxide core and 5-FU-loaded nanoparticles without the iron oxide core in combination with hyperthermia are shown in . Induction of DNA damage in the spheroid cultures of HT-29 cells increased with an increase in the concentration of 5-FU released from both types of nanoparticles in the presence of hyperthermia. However, the extent of DNA damage induced by 5-FU-loaded nanoparticles with the iron oxide core in combination with hyperthermia was significantly higher than that induced by 5-FU-loaded nanoparticles without the iron oxide core in combination with hyperthermia (p < 0.001). These results highlighted the importance of the iron oxide core in the induction of DNA damage at high temperature and indicated that the iron oxide core intensified the effect of hyperthermia.
Discussion
Colon cancer is a common cancer worldwide. This study used spheroid cultures of human colon cancer cell line HT-29 because studies have shown that spheroid cultures are more similar to in vivo conditions than other cultures [Citation24]. 5-FU, a halogenated pyrimidine analogue that replaces thymine in DNA and changes the core functions of cells, is used for treating colon cancer [Citation5]. However, the indiscriminate nature of 5-FU is a major limitation for its use. To overcome this, new therapies such as those involving nanoparticles are used to release drugs into tumour cells at a molecular level, thereby increasing the concentration of these drugs within tumour cells and in turn the sensitivity of the tumour to the drugs. Use of hyperthermia during chemotherapy increases the temperature of tumour cells and intensifies the effects of drugs and nanoparticles [Citation12].
In the present study no significant differences were observed in the induction of DNA damage between control cells and cells treated with <10 µM 5-FU (). This result was consistent with the results of a study by Salti et al. [Citation25] in which HT-29 cells were treated with genistein and were examined using the comet assay. A study by Fairbairn et al. showed that hyperthermia alone did not result in significant DNA damage [Citation26]. However, significant differences were observed in the induction of DNA damage by 5-FU monotherapy and 5-FU and hyperthermia combination therapy in the present study, suggesting a synergistic effect of the drug and hyperthermia. This result was consistent with that reported by Kido et al. [Citation27] who showed that the percentage of V-79 cells in the S-phase increased after treatment with 5-FU. Because cells in the S-phase are sensitive to heat, significant genotoxic damage was observed after the application of hyperthermia.
Nanoparticles differ in size, drug loading capacity, drug stability, drug release, targeting ability and capsule construction material and methods, which influence the effectiveness of treatment. Compared to more recent studies involving PLGA-based nanoparticles [Citation19,Citation28], we used structurally desirable nanoparticles that could enter the cells of the digestive system for treating colon cancer.
To evaluate the genotoxic effects of the nanoparticles, we determined whether these nanoparticles could enter cells by using TEM imaging (). Black grains, indicated by arrows, represent nanoparticles present in the spheroid cultures of HT-29 cells. This method was also used by in Cherukuri et al. [Citation15].
Because 5-FU is hydrophilic, it cannot easily cross the cell membrane, which is composed of phospholipids. However, loading of this drug into polymeric nanoparticles facilitates its entry into cells through phagocytosis. The subsequent release of the drug into the cells increases its concentration within the cells, thereby increasing DNA damage. The extent of DNA damage induced by 5-FU released by 5-FU-loaded nanoparticles with or without the iron oxide core was significantly higher than that by the same concentration of 5-FU alone (). Therefore, the resultant DNA damage can be attributed to the ability of nanoparticles to release a large amount of the drug into tumour cells. The present study is consistent with that reported by to Mohammadi et al. [Citation29] and Nair et al. [Citation28] by comet assay and colony assay, respectively. Free PLGA-coated nanoparticles with or without the iron oxide core had no significant effect on the induction of DNA damage (). This is because PLGA is a biocompatible polymer and its use is approved by the US Food and Drug Administration [Citation30]. In addition, no significant difference was observed in the tail moment of either type of nanoparticles (with or without the iron oxide core), indicating that the iron oxide core did not induce DNA damage.
As stated previously, hyperthermia plays an important role in increasing the sensitivity of cells and level of DNA damage ( and ). The extent of DNA damage increased with an increase in the amount of drug released from the nanoparticles. Moreover, the extent of DNA damage induced by 5-FU-loaded nanoparticles in combination with hyperthermia was significantly higher than that induced by 5-FU-loaded nanoparticles alone, indicating a synergistic effect of the nanoparticles and hyperthermia. Khoei et al. [Citation31] investigated the effect of quercetin-loaded PEG-polyester-PEG nanoparticles in combination with hyperthermia in DU-145 cells and provided confirmatory evidence.
Results of the effect of nanoparticles with and without the iron oxide core in combination with hyperthermia are shown in . Significant differences were observed in the two types of nanoparticles at all concentrations of 5-FU. The extent of DNA damage induced by 5-FU-loaded nanoparticles with the iron oxide core in combination with hyperthermia was higher than that induced by 5-FU-loaded nanoparticles without the iron oxide core in combination with hyperthermia, suggesting a synergistic effect of the iron oxide core and hyperthermia in inducing DNA damage. The iron oxide alone had no significant effect on the induction of DNA damage; however, it showed a synergistic effect in combination with hyperthermia. Jordan et al. [Citation32] compared treatment with magnetic fluid hyperthermia (MFH) and water bath hyperthermia and showed that the use of ferrofluid during treatment with water bath hyperthermia or MFH reduced the shoulder of dose–response curves of cells pre-incubated with a normal medium at 43 °C. Freeman et al. found that l mM iron that is not cytotoxic to CHO cells at 37 °C became cytotoxic as the temperature increased to 43 °C (relative toxicity factor, 2–4), which is roughly in accordance with our results () (difference between water bath hyperthermia with or without the iron oxide core). This thermosensitising effect of the iron oxide core at 43 °C may be because of oxidative stress induced by ferric ions, which promotes the formation of hydroxyl radicals in aqueous solution [Citation33].
Shakeri-Zadeh et al. [Citation34] investigated the effect of increasing temperature on the effectiveness of nanoparticles with the iron oxide core. Application of ultrasound increased the temperature of the nanoparticles by approximately 2.7 °C. This result is consistent with the results of the present study.
Conclusion
Our results demonstrate that hyperthermia is a sensitiser agent and nanoparticles can readily facilitate the cellular entrance of 5-FU into cancer cells. Our results clearly show that the iron oxide core of nanoparticles can act as a thermosensitiser and increase the genotoxicity of hyperthermia.
Acknowledgements
This work was supported by grant No. 21533 from the School of Medicine, Iran University of Medical Sciences (IUMS).
Declaration of interest
The authors report no conflicts of interest. The authors alone are responsible for the content and writing of the paper.
References
- Haggar FA, Boushey RP. Colorectal cancer epidemiology: Incidence, mortality, survival, and risk factors. Clin Colon Rectal Surg 2009;22:191–7
- Labianca R, Beretta G, Gatta G, de Braud F, Wils J. Colon cancer. Crit Rev Oncol Hematol 2004;51:145–70
- Takahashi I, Emi Y, Hasuda S, Kakeji Y, Maehara Y, Sugimachi K. Clinical application of hyperthermia combined with anticancer drugs for the treatment of solid tumors. Surgery 2002;131:S78–84
- Maeta M, Sawata T, Kaibara N. Effects of hyperthermia on the metabolism of 5-fluorouracil in vitro. Int J Hyperthermia 1993;9:105–13
- De Angelis PM, Svendsrud DH, Kravik KL, Stokke T. Cellular response to 5-fluorouracil (5-FU) in 5-FU-resistant colon cancer cell lines during treatment and recovery. Mol Cancer 2006;5:20
- Arias JL. Novel strategies to improve the anticancer action of 5-fluorouracil by using drug delivery systems. Molecules 2008;13:2340–69
- Berrada M, Yang Z, Lehnert S. Tumor treatment by sustained intratumoral release of 5-fluorouracil: Effects of drug alone and in combined treatments. Int J Radiat Oncol Biol Phys 2002;54:1550–7
- Figueiredo M, Esenaliev R. PLGA nanoparticles for ultrasound-mediated gene delivery to solid tumors. J Drug Deliv 2012;2012:767839
- Fernandez-Carballido A, Pastoriza P, Barcia E, Montejo C, Negro S. PLGA/PEG-derivative polymeric matrix for drug delivery system applications: Characterization and cell viability studies. Int J Pharmaceut 2008;352:50–7
- Rabiskova M, Song J, Opawale FO, Burgess DJ. The influence of surface properties on uptake of oil into complex coacervate microcapsules. J Pharm pharmacol 1994;46:631–5
- Ivkov R. Magnetic nanoparticle hyperthermia: A new frontier in biology and medicine? Int J Hyperthermia 2013;29:703–5
- Kakehi M, Ueda K, Mukojima T, Hiraoka M, Seto O, Akanuma A, et al. Multi-institutional clinical studies on hyperthermia combined with radiotherapy or chemotherapy in advanced cancer of deep-seated organs. Int J Hyperthermia 1990;6:719–40
- Wust P, Hildebrandt B, Sreenivasa G, Rau B, Gellermann J, Riess H, et al. Hyperthermia in combined treatment of cancer. Lancet Oncol 2002;3:487–97
- Hautot D, Pankhurst QA, Khan N, Dobson J. Preliminary evaluation of nanoscale biogenic magnetite in Alzheimer’s disease brain tissue. Proc Biol Sci 2003;270:S62–4
- Cherukuri P, Glazer ES, Curley SA. Targeted hyperthermia using metal nanoparticles. Adv Drug Deliv Rev 2010;62:339–45
- McLoughlin P, Roengvoraphoj M, Gissel C, Hescheler J, Certa U, Sachinidis A. Transcriptional responses to epigallocatechin-3 gallate in HT 29 colon carcinoma spheroids. Genes Cells 2004;9:661–9
- Weiswald LB, Guinebretiere JM, Richon S, Bellet D, Saubamea B, Dangles-Marie V. In situ protein expression in tumour spheres: Development of an immunostaining protocol for confocal microscopy. BMC Cancer 2010;10:106
- Mueller-Klieser W. Multicellular spheroids. A review on cellular aggregates in cancer research. J Cancer Res Clin Oncol 1987;113:101–22
- Ashjari M, Khoee S, Mahdavian AR. A multiple emulsion method for loading 5-fluorouracil into a magnetite-loaded nanocapsule: A physicochemical investigation. Polym Int 2012;61:850–9
- Khoee S, Yaghoobian M. An investigation into the role of surfactants in controlling particle size of polymeric nanocapsules containing penicillin-G in double emulsion. Eur J Med Chem 2009;44:2392–9
- Khoee S, Hassanzadeh S, Goliaie B. Effects of hydrophobic drug–polyesteric core interactions on drug loading and release properties of poly(ethylene glycol)-polyester-poly(ethylene glycol) triblock core-shell nanoparticles. Nanotechnology 2007;18:175602
- Rao GH, Liu HM, Li BW, Hao JJ, Yang YL, Wang MR, et al. Establishment of a human colorectal cancer cell line P6C with stem cell properties and resistance to chemotherapeutic drugs. Acta Pharmacol Sinica 2013;34:793–804
- Fazeli GR, Khoei S, Nikoofar AR, Goliaei B. Reduced DNA damage in tumor spheroids compared to monolayer cultures exposed to ionizing radiation. Iran J Radiat Res 2007;5:63–9
- Sutherland AE, Calarco PG, Damsky CH. Expression and function of cell surface extracellular matrix receptors in mouse blastocyst attachment and outgrowth. J Cell Biol 1988;106:1331–48
- Salti GI, Grewal S, Mehta RR, Das Gupta TK, Boddie AW, Jr Constantinou AI. Genistein induces apoptosis and topoisomerase II-mediated DNA breakage in colon cancer cells. Eur J Cancer 2000;36:796–802
- Fairbairn JJ, Khan MW, Ward KJ, Loveridge BW, Fairbairn DW, O’Neill KL. Induction of apoptotic cell DNA fragmentation in human cells after treatment with hyperthermia. Cancer Lett 1995;89:183–8
- Kido Y, Kuwano H, Maehara Y, Mori M, Matsuoka H, Sugimachi K. Increased cytotoxicity of low-dose, long-duration exposure to 5-fluorouracil of V-79 cells with hyperthermia. Cancer Chemother Pharmacol 1991;28:251–4
- Nair KL, Jagadeeshan S, Nair SA, Kumar GS. Biological evaluation of 5-fluorouracil nanoparticles for cancer chemotherapy and its dependence on the carrier, PLGA. Int J Nanomed 2011;6:1685–97
- Mohammadi S, Khoei S, Mahdavi SR. The combination effect of poly (lactic-co-glycolic acid) coated iron oxide nanoparticles as 5-fluorouracil carrier and X-ray on the level of DNA damages in the DU 145 human prostate carcinoma cell line. J Bionanosci 2012;6:23–7
- Zhu L, Ma J, Jia N, Zhao Y, Shen H. Chitosan-coated magnetic nanoparticles as carriers of 5-fluorouracil: Preparation, characterization and cytotoxicity studies. Colloids Surf B Biointerfaces 2009;68:1–6
- Khoei S, Azarian M, Khoee S. Effect of hyperthermia and triblock copolymeric nanoparticles as quercetin carrier on DU145 prostate cancer cells. Curr Nanosci 2012;8:690–6
- Jordan A, Wust P, Scholz R, Tesche B, Fahling H, Mitrovics T, et al. Cellular uptake of magnetic fluid particles and their effects on human adenocarcinoma cells exposed to AC magnetic fields in vitro. Int J Hyperthermia 1996;12:705–22
- Freeman ML, Spitz DR, Meredith MJ. Does heat shock enhance oxidative stress? Studies with ferrous and ferric iron. Radiat Res 1990;124:288–93
- Shakeri-Zadeh A, Khoei S, Khoee S, Sharifi AM, Shiran M-B. Combination of ultrasound and newly synthesized magnetic nanocapsules affects the temperature profile of CT26 tumors in BALB/c mice. J Med Ultrason 2015;42:9–16