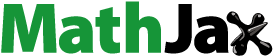
Abstract
Interventional oncology procedures such as thermal ablation are becoming widely used for many tumours in the liver, kidney and lung. Thermal ablation refers to the focal destruction of tissue by generating cytotoxic temperatures in the treatment zone. Hydrodissection – separating tissues with fluids – protects healthy tissues adjacent to the ablation treatment zone to improve procedural safety, and facilitate more aggressive power application or applicator placement. However, fluids such as normal saline and 5% dextrose in water (D5W) can migrate into the peritoneum, reducing their protective efficacy. As an alternative, a thermo-gelable poloxamer 407 (P407) solution has been recently developed to facilitate hydrodissection procedures. We hypothesise that the P407 gel material does not provide convective heat dissipation from the ablation site, and therefore may alter the heat transfer dynamics compared to liquid materials during hydrodissection-assisted thermal ablation. The purpose of this study was to investigate the heat dissipation mechanics within D5W, liquid P407 and gel P407 hydrodissection barriers. Overall it was shown that the gel P407 dissipated heat primarily through conduction, whereas the liquid P407 and D5W dissipated heat through convection. Furthermore, the rate of temperature change within the gel P407 was greater than liquid P407 and D5W. Testing to evaluate the in vivo efficacy of the fluids with different modes of heat dissipation seems warranted for further study.
Introduction
Minimally invasive thermal ablation procedures have become a widely accepted method of treating tumours in the liver, kidney, lungs and bone [Citation1–7]. During these procedures, one or more applicators are inserted into the tumour using medical imaging such as ultrasound or computed tomography (CT) for guidance. The applicator is then used to either cool or heat the surrounding tissue sufficiently to cause focal tissue necrosis. While several ablative modalities have been described, the greatest clinical experiences have utilised radiofrequency (RF) electrical current or microwaves for hyperthermic ablation, and cryoablation for hypothermic ablation [Citation7–11]. Regardless of the energy used, the lack of an ablative margin of normal tissue around the tumour, or proximity to large blood vessels that counteract the desired heating or cooling, are key factors in local recurrence after thermal ablation procedures [Citation4,Citation12]. Therefore, aggressive treatment and larger ablation zones are desirable in most clinical presentations.
One consequence of creating larger ablation zones is an increased likelihood of encountering critical anatomical structures that should not be ablated, such as bile ducts, major blood vessels, diaphragm, body wall, stomach, bowel, nerves, and pancreas [Citation5,Citation6,Citation11,Citation13]. Damage to these tissues can cause complications ranging from minor discomfort and pain to bowel perforation or death [Citation5,Citation6,Citation11]. Therefore, adjunctive techniques such as hydrodissection, gas insufflation, hypoperfusion, and body positioning are now routinely used to reduce the impact of thermal ablation to nearby critical structures [Citation6,Citation13–15]. Such techniques permit more aggressive treatment while improving procedural safety.
The most widely used adjunctive technique is hydrodissection, where fluid is injected between the target zone and susceptible tissues to provide physical displacement, a thermal buffer and, in the case of RF ablation, an electrical barrier. Clinically used liquids include sterile water, 5% dextrose in water (D5W), and normal saline, all of which have been shown to reduce complications from thermal ablation procedures [Citation5,Citation6,Citation11,Citation13,Citation15]. There is also some evidence that these liquid materials act as a heat sink; that is, liquids provide greater heat transfer from the ablation zone due to free convection, which may affect ablation zone size or shape similar to forced convection from blood flow in adjacent vessels (but to a lesser degree) [Citation15–19].
As an alternative, a poloxamer 407 (P407) solution has been recently developed to facilitate hydrodissection procedures. The P407 solution is a thermo-reversible hydrogel that is a low viscosity liquid that can be injected through thin needles at room temperature, but which becomes a highly viscous, semi-solid gel at body temperature [Citation20]. As a result, the gel P407 is more stable, may reduce the volume of material needed, afford greater placement control, and provide longer dwell time than liquid materials [Citation20]. Furthermore, P407 is considered bioabsorbable and is excreted via the kidneys within a few days [Citation20–22]. Chitosan-based gels have also been described and studied as a hydrodissection material [Citation23]. Previous work has suggested that a thicker barrier may be needed with the gel material than with liquid materials such as D5W due to a lack of convective heat transfer in the gel [Citation20]. Therefore, the purpose of this study was to analyse heat dissipation dynamics within liquid and gel hydrodissection barriers, and determine how these dynamics influence thermal protection. This analysis will yield insight into different strategies for implementing hydrodissection during ablation procedures.
Materials and methods
Material formulation
P407 hydrodissection solution was created from 15.4 w/w% P407 (Sigma Aldrich, St Louis, MO) in deionised water [Citation24]. This formulation was described previously and forms a gel at 32 °C [Citation20]. D5W was obtained from Hospira (Lake Forest, IL).
Direct heating of hydrodissection fluids
The first experiment was designed to assess heat transfer in response to an ablation device applied to each fluid. A microwave ablation antenna (PR15, NeuWave Medical, Madison, WI) was placed 7 cm deep into 230 mL of either P407 or D5W fluid (). Fibre-optic temperature probes (Neoptix, Toronto, Canada) were inserted 5.5 cm into the fluid through steel tubes to measure heat generation 16.5 mm and 33 mm around the antenna (). D5W was initially at room temperature (∼20 °C). The P407 formulation was initially at 8 °C to evaluate heat transfer as a liquid, during polymer micellisation at ∼23 °C, after gelation at 32 °C, and after gel melting above 54 °C.
Figure 1. Set-up for fluid heating rate testing: 230 mL of hydrodissection fluid was placed within a plastic container and subjected to MW ablation for 15 or 20 min for D5W and P407, respectively. (A) Top view. Temperature probes were placed 1.65 cm apart from one another and the ablation probe, creating two concentric rings (inner and outer). (B) Side view. Steel tubes were used to maintain temperature probe locations within the fluid. The ablation probe, steel tubes and temperature probes were inserted 7, 5 and 5.5 cm into the fluid, respectively. Temperature readings were obtained every second.
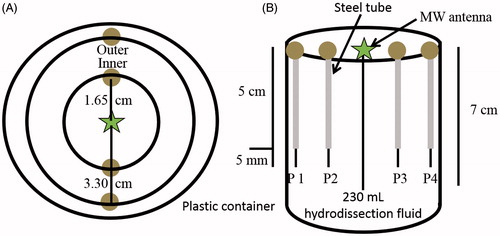
A total of 90 W at 2.45 GHz was continuously applied to the antenna for 15 min in D5W and 20 min in P407. Temperatures were recorded every second. Rates of temperature change were then calculated using a 20-s moving window. Each material was evaluated in triplicate. Raw temperatures and rates of temperature change were compared between materials (D5W, gel P407, and liquid P407) using unpaired two-tailed Student’s t-tests.
In an effort to visualise variations in heat dissipation, this same set-up was used except all temperature probes were removed, leaving only the container, microwave antenna, and solution (230 mL). D5W was used at room temperature (∼20 °C) and drops of blue food colouring were added to visualise developing convection currents. As a control, drops of blue food colouring were added to D5W but without energy application; i.e. to visualise only dye diffusion. One 115-mL aliquot of liquid P407 was left clear, while another 115-mL aliquot was coloured green with two drops of green food colouring. The green liquid P407 was gelled in a 40 °C bath for 1 h. The clear liquid P407 was then poured on top of the green gel P407 and gelled in a 40 °C bath for 1 h; this created a layered P407 gel with the inferior layer being green in colour, and the superior layer being clear and colourless. The microwave antenna was placed, and microwave energy was applied, as described above. Digital video was recorded of each heating cycle to facilitate later analysis.
Heat dissipation from ablations in ex vivo liver
The next experiment was designed to evaluate heat transfer in a hydrodissection barrier during thermal ablation. Two sections of bovine liver were separated by 11 mm of either D5W or P407 hydrodissection material as in a previous study () [Citation20]. Tissue separation was maintained by two fibreglass mesh spacers to allow both heat and material transfer while maintaining parallel surfaces. P407 was tested in gelled form at 32 °C (gel P407) and liquid form at 4 °C (liquid P407). The temperature of the bovine liver sections was identical to the P407 during respective testing; D5W testing used bovine liver sections also at 32 °C. Both phases were tested to evaluate the hypothesis that liquid P407 would exhibit convective heat transfer more similar to D5W, while gel P407 would exhibit mostly conductive heat transfer due to its high viscosity.
Figure 2. (A) Set-up for ex vivo ablation experiments in a plastic container. Two bovine liver pieces are held at a constant distance, d = 11 mm, by two plastic gates. This separation enables the establishment of a hydrodissection fluid barrier. The ablation probe was placed 1 cm away from the hydrodissection fluid. Temperature probes 1–4 were organized in this fashion to examine convective versus conductive heat dissipation within the hydrodissection barrier; temperature readings were obtained every second. (B) Plastic gate construction. A rectangular opening was cut from the middle of a piece of plastic. Screen mesh was secured over the opening using plastic screws. The mesh-covered opening allowed for direct heat transfer from the neighbouring liver pieces to the hydrodissection fluid while maintaining a constant hydrodissection distance.
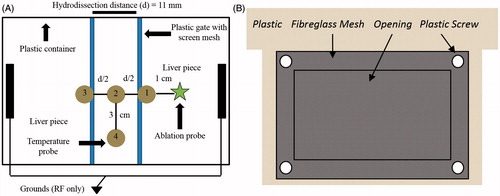
The ablation applicator was placed vertically 4.5 cm into the liver tissue, 1 cm from the fluid barrier. For RF ablations, a 3 cm water-cooled electrode (Covidien, Mansfield, MA) delivered a maximum of 200 W from the RF ablation generator under internal impedance control for 10 min. For microwave ablations, a gas-cooled triaxial antenna delivered 90 W from a 2.45 GHz generator (NeuWave Medical) for 10 min. These settings were used to produce similar ablation zone sizes between RF and microwave ablations based on previous studies [Citation6,Citation11,Citation20].
Temperatures within the hydrodissection fluid were monitored 3.5 cm deep at four locations every second using fibre-optic temperature probes (Neoptix). Probes 1–3 were placed along the line of greatest heat flux away from the ablation applicator, with probe 1 at the edge of the barrier nearest to the applicator, probe 2 in the middle of the barrier, and probe 3 at the edge of the barrier farthest from the applicator. Probe 4 was placed in the middle of the barrier in a plane orthogonal to probes 1–3. With this set-up, temperature increases isolated to probes 1 and 2 were indicative of conductive heat transfer from the ablation site. Temperature increases of similar magnitude at probes 2–4 indicated a greater contribution from free convective heat transfer. Maximum temperature rise was compared between groups using unpaired two-tailed Student’s t-tests.
Results
Direct heating of hydrodissection fluids
Temperatures measured during direct material heating demonstrated trends consistent with greater convective heat transfer in liquid phases with a transition to conductive heat transfer in gelled phases (). No differences were noted in the rate of temperature change of D5W or liquid P407 (3.4 ± 0.9 °C/min and 3.7 ± 0.8 °C/min, respectively; p = 0.30). In addition, little variation in temperatures between the inner and outer locations was noted in D5W and liquid P407 (0.6 ± 0.1 °C and 1.2 ± 0.3 °C, respectively). The rate of temperature change for D5W at the inner and outer locations did not deviate by more than 0.32 °C/min at any specific time. However, during the micellisation and gelling of P407, the rate of temperature change of the inner probes increased to 6.6 ± 0.7 °C/min, while the outer rate of temperature change decreased to 2.1 ± 0.3 °C/min. In the micellisation and gelling phase the largest recorded temperature difference between the inner and outer locations was 17.2 °C, an over 10-fold increase compared to average temperature differences seen in the liquid P407 (1.2 °C). Near the gel P407’s melting temperature (∼54 °C) the inner rate of temperature change dropped to 2.5 ± 0.7 °C/min while the outer rate of temperature change increased substantially to 11.3 ± 2.5 °C/min. Above 60 °C, both inner and outer P407 rates of temperature change converged to 2.0 ± 0.3 °C/min.
Figure 3. (A and B) Temperature versus time for MW ablation in 230 mL of D5W and P407, respectively, using the set-up depicted in . The probe temperatures within D5W were very similar during the entire experiment due to convective heat dissipation, resulting in a rate of temperature change of 3.4 ± 0.9 °C/min. Similarly, the liquid P407 (8 °C) exhibited similar probe temperatures until the P407 started to micellise (at ∼23 °C) and gel (at 32 °C) where a significant difference in temperatures was seen. To further examine the P407 data, dT/dt were calculated and plotted in C and D, showing the rates of temperature change for probes 2 and 3, and probes 1 and 4, respectively. The rate of temperature change for probes 2 and 3 increased drastically as the P407 started to micellise and gel. As a gel the P407 cannot convectively dissipate heat to the outer locations, probes 1 and 4 experienced a decrease and plateau in rate of temperature change. Once the P407 gel melted (at ∼54 °C) convective heat dissipation again became dominant and resulted in the large rate of temperature change experienced for probes 1 and 4. This shows that the P407 gel primarily dissipates heat through conduction, whereas D5W and liquid P407 are convection dominated.
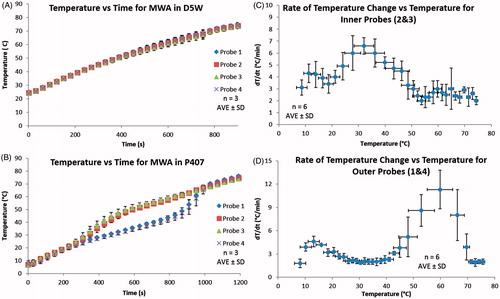
Video images showed visual differences between convective and conductive heat dissipation (). Due to the decrease in density of heated fluid next to the applicator, a strong convection current was created in the D5W experiment that was not seen in the D5W diffusion control. This resulted in the dye being pulled to the surface during the first 13 s instead of falling to the bottom of the container as in the diffusion control at 20 s. As the experiment continued, the blue dye outlined the entire convection cell. Conversely, gel P407 cannot dissipate heat through convection. Instead, conduction transfers heat radially from the source, yielding the green expanding sphere shown in . This also provides insight into the speed of dissipation modes, as convection quickly manoeuvres/dissipates the dye yet conduction does so more slowly.
Figure 4. Freeze frame images to visualise heat dissipation. Top row: convection in D5W manipulates the blue dye allowing for visualisation of the convection cell. This contrasts with the control (middle row) where the blue dye sinks and only dissipates via diffusion in D5W. 0’ designates the time point immediately after administration of one blue dye drop. Bottom row: conduction in a layered P407 gel yields a green sphere that increases radially from the active portion of the microwave antenna.
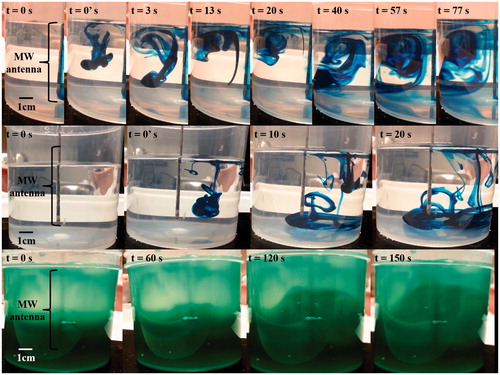
Heat dissipation during RF ablation in ex vivo liver
Temperatures measured in a hydrodissection barrier during RF ablation supported the trends discovered in the direct heating experiments (). Temperature elevations at probe 1 were significantly greater in gel P407 as compared to D5W (31.2 ± 10.7 °C versus 14.1 ± 5.6 °C, respectively; p = 0.05) (), and slightly, but not significantly, greater than liquid P407 (24.5 ± 2.0 °C; p = 0.30). The temperature difference between probes 2 and 4 was also significantly greater in gel P407 compared to D5W and liquid P407 (9.8 ± 3.8 °C versus 1.0 ± 1.1 °C versus 2.2 ± 0.5 °C, respectively; p < 0.02). Liquid P407 showed a significantly higher probe 1 temperature than in D5W (24.5 ± 2.0 °C versus 14.1 ± 5.6 °C, respectively, p = 0.04); however, the temperature difference between probes 2 and 4 was not different between liquid P407 and D5W (2.2 ± 0.5 °C versus 1.0 ± 1.1 °C, respectively, p = 0.50).
Figure 5. (A) 11 mm of D5W, (B) 11 mm of gel P407, and (C) 11 mm of liquid P407. A Cool-Tip RF ablation machine was used to perform RF ablation on liver pieces depicted in the experimental set-up in . Ablations were performed for 10 min and temperatures at the corresponding locations were obtained every second. Temperature readings at every 30 s are plotted. Gel P407 had the highest probe 1 temperature and difference between probes 2–4, while D5W and liquid P407 had lower probe 1 temperatures and more similar values for probes 2–4. These results are due to D5W and liquid P407 being able to convectively dissipate heat from probe 1 throughout the bulk of the fluid, whereas the gel P407 is unable to convectively transfer heat to the periphery.
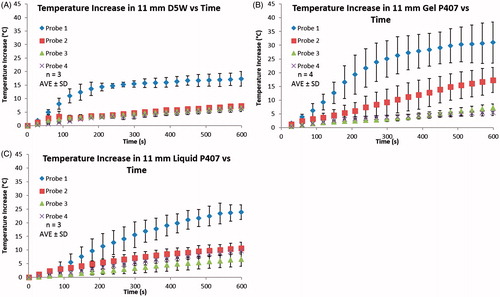
Table 1. Temperature measurements within the hydrodissection barrier after ex vivo RF and microwave using bovine liver. Probe locations as per .
Heat dissipation during microwave ablation in ex vivo liver
Temperature changes in the hydrodissection barrier during microwave ablation were similar to those observed during RF ablation ( and ). Temperature elevations at probe 1 were again significantly greater in gel P407 and liquid P407 as compared to D5W (30.9 ± 7.1 °C, 35.9 ± 7.7 °C, and 14.7 ± 3.3 °C, respectively; p < 0.02). No statistical significance was seen at probe 1 between gel P407 and liquid P407 (p = 0.42). Gel P407 showed a significantly larger temperature difference between probes 2 and 4 than liquid P407 and D5W (10.2 ± 0.5 °C, 1.3 ± 0.8 °C, and 1.1 ± 0.9 °C, respectively; p < 0.0001), but the difference between liquid P407 and D5W was not statistically different (p = 0.65).
Figure 6. (A) 11 mm of D5W, (B) 11 mm of gel P407, and (C) 11 mm of liquid P407. A Neuwave MW ablation machine was used to perform MW ablation on liver pieces depicted in the experimental set-up in . Ablations were performed for 10 min and temperatures at the corresponding locations were obtained every second. Temperature readings at every 30 s are plotted. Gel P407 had the highest temperature difference between probes 2–4 while D5W and liquid P407 have more similar values for probes 2–4. Again, these results are due to D5W and liquid P407 being able to convectively dissipate heat from probe 1 throughout the bulk of the fluid, whereas the gel P407 is unable to convectively dissipate heat. Here liquid P407 behaves like the gel P407 at probe 1 (because this location is a gel) while the liquid P407 behaves like D5W at probes 2–4.
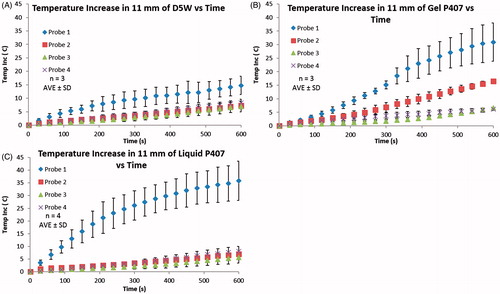
Discussion
The increased development and clinical utilisation of hydrodissection materials during thermal ablation has illuminated the need for a greater understanding of heat transfer mechanisms in those materials. Specifically, there is a gap in knowledge about the relative roles of convective and conductive heat transfer in the hydrodissection barrier itself. In this study it was shown that gel P407 dissipates heat primarily through conduction, which causes greater local heating inside the protective barrier, especially near the ablation heat source (probe 1). Conversely, D5W and liquid P407 allow for free convection, resulting in less local heating and greater heat transfer throughout the fluid bulk (similar temperatures at probes 2–4 and similar temperature difference between probes 2 and 4). As expected, liquid P407 resembles gel P407 at high energy density locations (probe 1) where the liquid P407 is close to gelation, yet resembles D5W in the cooler fluid bulk.
The presence of conduction or convection was best illustrated by direct microwave heating of the material alone. Temperatures increased at a relatively linear rate (R = 0.99) from 25–75 °C in D5W with little variation between the inner and outer measurement locations, which is consistent with expected trends for a high rate of free convection. While a similar trend was noted in liquid P407 below 23 °C, heating shifted towards the antenna during micellisation and gelation (23–32 °C). Since microwave heating is greatest near the antenna, temperatures rose fastest at the inner measurement location. The higher viscosity of the gel P407 restricted fluid movement and effectively eliminated free convection. Consistent with energy conservation, as more energy was retained near the applicator, less energy was transferred to the peripheral space, resulting in a decreased rate of temperature change at the peripheral measurement locations.
Accordingly, the phase of the hydrodissection material substantially influenced the mode of heat transfer within hydrodissection barriers during ex vivo tissue ablation testing. The hypothesis that convection was the dominant mechanism in liquid materials was supported by lower temperatures near the ablation site and less spatial variance within the fluid bulk compared to gelled materials. Conductive heat transfer dominated in the gelled material, as supported by the increased temperature elevation near the ablation site and reduced heat transfer into the adjacent fluid bulk. In particular, we noted that much of the heat from the ablation site was trapped in the adjacent gel due to the relatively slow rate of conduction in these materials. These results appear to explain why a smaller barrier of liquid D5W was required than gel P407 for the same protective effect [Citation20], since convection allows the whole D5W barrier to serve as a heat sink while conduction limits heat transfer to the gel P407 bulk.
In fluid mechanics, the dimensionless Rayleigh number (Ra) can be used to determine whether conductive or convective heat transfer dominates. The Ra number is defined by Equation Equation1(1)
(1) :
(1)
(1)
where g is acceleration due to gravity, β is the fluid’s volumetric thermal expansivity, ΔT is the temperature difference between the fluid and solid boundary, L is the distance between the two locations, ν is the fluid’s kinematic viscosity, and α is the fluid’s thermal diffusivity. At low Ra values, conductive heat transfer dominates within fluids and there is a lack of fluid movement. A switch to convection domination, driven by an increasing buoyancy force, occurs at a critical Ra number of approximately 1700 [Citation25]. Using the ex vivo results for microwave testing (Probes 1 and 4: L = 3.05 cm, ΔT = temperature difference) and known values of β, ν, and α for gel and liquid P407 [Citation20,Citation26] and water (for D5W, [Citation25]) yields Ra values of 9.6e + 05, 1.2, and 1.8e + 06 for liquid P407, gel P407, and D5W, respectively. The calculated Ra numbers infer convective heat transfer in D5W and liquid P407, and conductive heat transfer in gel P407. The variable of greatest effect is kinematic viscosity, as there exists a roughly six order of magnitude difference between viscosities of D5W and liquid P407, compared to gel P407 [Citation20]. Lastly, when 1700 < Ra < 10e + 09, convection currents display laminar flow [Citation25]. This was shown in D5W testing where laminar flow of the blue dye is visible, seen in particular at t = 13 s and t = 77 s where separate layers of blue D5W and clear D5W are visible within the convection current ().
A summary of physical, thermal, and electrical properties can be found in . Using these properties also allows for the calculation of the fluids’ thermal conductivities (k) using Equation Equation2(2)
(2) .
(2)
(2)
where α is the thermal diffusivity, ρ is the density, and Cp is the specific heat. This results in thermal conductivity values of 0.59, 0.43, and 0.31 W/m/K for water, liquid P407 and gel P407, respectively. As the gel P407 has smaller thermal diffusivity and thermal conductivity values than water and liquid P407, it is expected that the gel P407 would provide more of an insulation effect during ex vivo testing, as shown in this manuscript. It should be noted that the fluids’ properties and Ra values will vary with temperature.
Table 2. Physical, thermal, and electrical properties of water, D5W, liquid P407, and gel P407. Gel P407 has smaller thermal diffusivity and thermal conductivity values than water and liquid P407, resulting in its ability to retain heat adjacent to the ablation zone rather than transfer the heat to the periphery.
The results of this study have several clinical implications. First, convective heat transfer in liquid hydrodissection fluids provides a heat sinking effect from the ablation zone, reducing the amount of energy delivered to the boundary tissue region. Specifically, during D5W testing the P1 probe resulted in approximately half the temperature increase of P1 during gel P407 testing for both RF and microwave ablations. Nevertheless, the rapid heat transfer provided by D5W explains why a relatively small 5 mm barrier of D5W was effective in previous laboratory and clinical studies evaluating thermal/electrical protection [Citation5,Citation6,Citation20,Citation31]; however, an analysis of possible recurrence at this boundary region due to the heat sink effect has yet to be performed. Conversely, a gel P407 barrier traps heat at the ablation boundary, due to smaller thermal conductivity and diffusivity values, effectively eliminating any heat sink effect. The application of such a gel may be advantageous when tumours directly abut the capsule of an organ; trapping heat at the tumour/organ boundary may yield lower recurrence rates by ensuring all tumour cells are destroyed within this region. Moreover, it may be easier to create large gel barriers and improve barrier efficacy near critical structures, as the gel will not migrate in vivo. In addition, this study confirmed the previous finding that an 11-mm hydrodissection barrier of any material prevents dangerous temperature elevations within the adjacent protected tissue [Citation20]. Temperature elevations at probe 3 were approximately 5–7 °C in all trials, which would be well tolerated clinically [Citation32,Citation33]. Lastly, as free convection is a phenomenon that occurs against gravity, the convective heat transfer in D5W may result in more heat delivery to tissues at greater elevation than the ablation site. These effects should be considered clinically to further improve hydrodissection and ablation efficacy.
Other potential thermo-gelable hydrodissection alternatives could include poloxamer 188 (P188), methylcellulose, and chitosan. P188 has a similar thermo-reversible property compared to P407; however, a lower concentration of P407 is needed for gelation, and P407 has been shown to offer superior biocompatibility [Citation34–36]. Methylcellulose solutions typically have a greater sol-to-gel transition temperature (40–50 °C] [Citation36,Citation37]. Furthermore, methylcellulose cannot be digested/absorbed very easily, whereas the P407 is readily absorbed and excreted in the urine [Citation21,Citation34]. Chitosan is a popular biomaterial and has been examined for use as a hydrodissection fluid [Citation23]. However, the gelation time of chitosan-based solutions is in the order of minutes, if not longer [Citation38], whereas the P407 solution gels within seconds upon in vivo injection [Citation39]. Thus, the P407 solution may offer significant advantages compared to other potential thermo-gelable alternatives.
A limitation of this study was that the ex vivo set-up likely exacerbated the differences between fluids, particularly during RF ablation experiments. The ablation applicator was placed only 1 cm away from the edge of the liver tissue, which would be a fairly aggressive placement near critical structures. In addition, only two possible electrical current pathways existed in the RF heating studies, which forced more electrical current through the barrier than would likely be experienced in a clinical setting [Citation5,Citation20]. Lastly, the overall set-up was not within a closed thermodynamic system, which limited the ability to quantify heat transfer throughout the entire system. However, given the relatively small surface area at the barrier–air interface, the effect of free convection or radiation from that interface was likely small compared to internal heat transfer and did not diminish the overall conclusions of this study.
Conclusion
The results of this study illustrate that free convection is the dominant mode of heat transfer in liquid hydrodissection fluids. Free convection can rapidly dissipate heat away from the ablation site and protect neighbouring tissues by distributing heat energy within the fluid. As a result, smaller barriers are required for liquid materials. Conductive heat transfer is more dominant in gel materials, resulting in more local heat storage and slower transfer. However, convection is more significant below the gelation temperature and above the melting temperature even in gel materials. Overall, the phase of the hydrodissection fluid is the main determinant for convective versus conductive heat transfer within the fluid. Further study into the heat transfer and thermal properties of hydrodissection fluids is necessary to help determine the optimal use of each material.
Declaration of interest
Chris Brace is a shareholder and consultant for NeuWave Medical, Inc., which develops interventional oncology techniques. We acknowledge the University of Wisconsin – Madison Graduate School’s Innovation and Economic Development Research Program for funding and use of facilities and instrumentation supported by the US National Science Foundation-funded University of Wisconsin Materials Research Science and Engineering Center (DMR-1121288). The authors alone are responsible for the content and writing of the paper.
References
- Permpongkosol S, Link RE, Kavoussi LR, Solomon SB. Temperature measurements of the low-attenuation radiographic ice ball during CT-guided renal cryoablation. Cardiovasc Intervent Radiol 2008;31:116–21
- Yoon J, Cho J, Kim N, Kim DD, Lee E, Cheon C, et al. High frequency microwave ablation method for enhanced cancer treatment with minimized collateral damage. Int J Cancer 2010;129:1970–8
- Rossi S, Di Stasi M, Buscarini E, Quaretti P, Garbagnati F, Squassante L, et al. Percutaneous RF interstitial thermal ablation in the treatment of hepatic cancer. Am J Roentgenol 1996;167:759–68
- Lubner MG, Brace CL, Hinshaw JL, Lee FT Jr. Microwave tumor ablation: Mechanism of action, clinical results, and devices. J Vasc Interv Radiol 2010;21(8):S192–203
- Hinshaw JL, Laeseke PF, Winter TC III, Kliewer MA, Fine JP, Lee FT Jr. Radiofrequency ablation of peripheral liver tumors: Intraperitoneal 5% dextrose in water decreases postprocedural pain. Am J Roentgenol 2006;186(Suppl 5):S306
- Brace CL, Laeseke PF, Prasad V, Lee FT Jr, editors. Electrical isolation during radiofrequency ablation: 5% dextrose in water provides better protection than saline. Conf Proc IEEE Eng Med Biol Soc, 2006;1:5021–4
- Paganini AM, Rotundo A, Barchetti L, Lezoche E. Cryosurgical ablation of hepatic colorectal metastases. Surg Oncol 2007;16:137–40
- Callstrom MR, Kurup AN. Percutaneous ablation for bone and soft tissue metastases – why cryoablation? Skeletal Radiol 2009;38:835–9
- Dodd GD, Soulen MC, Kane RA, Livraghi T, Lees WR, Yamashita Y, et al. Minimally Invasive treatment of malignant hepatic tumors: At the threshold of a major breakthrough. Radiographics 2000;20:9
- Kuang M, Lu MD, Xie XY, Xu HX, Mo LQ, Liu GJ, et al. Liver cancer: Increased microwave delivery to ablation zone with cooled-shaft antenna – experimental and clinical studies 1. Radiology 2007;242:914–24
- Laeseke PF, Sampson LA, Brace CL, Winter TC III, Fine JP, Lee FT Jr. Unintended thermal injuries from radiofrequency ablation: Protection with 5% dextrose in water. Am J Roentgenol 2006;186(Suppl 5):S249
- Gervais DA, McGovern FJ, Arellano RS, McDougal WS, Mueller PR. Renal cell carcinoma: Clinical experience and technical success with radio-frequency ablation of 42 tumors 1. Radiology. 2003;226:417–24
- Lee SJ, Choyke LT, Locklin JK, Wood BJ. Use of hydrodissection to prevent nerve and muscular damage during radiofrequency ablation of kidney tumors. J Vasc Interv Radiol 2006;17:1967–9
- Buy X, Tok C-H, Szwarc D, Bierry G, Gangi A. Thermal protection during percutaneous thermal ablation procedures: Interest of carbon dioxide dissection and temperature monitoring. Cardiovasc Intervent Radiol 2009;32:529–34
- Kitchin D, Lubner M, Ziemlewicz T, Hinshaw JL, Alexander M, Brace CL, et al. Microwave ablation of malignant hepatic tumours: Intraperitoneal fluid instillation prevents collateral damage and allows more aggressive case selection. Int J Hyperthermia 2014;30:299–305
- Wu C-C, Chen W-S, Ho M-C, Huang K-W, Chen C-N, Yen J-Y, et al. Minimizing abdominal wall damage during high-intensity focused ultrasound ablation by inducing artificial ascites. J Acoust Soc Am 2008;124:674–9
- Rhim H, Lim HK, Kim Y-S, Choi D. Percutaneous radiofrequency ablation with artificial ascites for hepatocellular carcinoma in the hepatic dome: Initial experience. Am J Roentgenol 2008;190:91–8
- Lu DS, Raman SS, Vodopich DJ, Wang M, Sayre J, Lassman C. Effect of vessel size on creation of hepatic radiofrequency lesions in pigs: Assessment of the ‘heat sink’ effect. Am J Roentgenol 2002;178:47–51
- Chiang J, Wang P, Brace CL. Computational modelling of microwave tumour ablations. Int J Hyperthermia 2013;29:308–17
- Johnson A, Sprangers A, Cassidy P, Heyrman S, Hinshaw JL, Lubner M, et al. Design and validation of a thermoreversible material for percutaneous tissue hydrodissection. J Biomed Mater Res B: Appl Biomater 2013;101:1400–9
- Yu L, Ding J. Injectable hydrogels as unique biomedical materials. Chem Soc Rev 2008;37:1473–81
- Pec E, Wout Z, Johnston T. Biological activity of urease formulated in poloxamer 407 after intraperitoneal injection in the rat. J Pharm Sci 1992;81:626–30
- Zhang D, Xie D, Wei X, Zhang D, Chen M, Yu X, et al. Microwave ablation of the liver abutting the stomach: Insulating effect of a chitosan-based thermosensitive hydrogel. Int J Hyperthermia 2014;30:126–33
- Schmolka I. Artificial skin I. Preparation and properties of pluronic F-127 gels for treatment of burns. J Biomed Mat Res 1972;6:571–82
- Bird S. Transport Phenomena, revised 2nd ed. Chichester: Wiley, 2007
- Johnson A. Evaluation of Poloxamer 407’s Efficacy as a Hydrodissection Fluid. University of Wisconsin thesis, Madison, WI, 2013
- Shubert D, Leyba J. Chemistry and Physics for Nurse Anesthesia: A Student-centered Approach. New York: Springer, 2013
- Blumm J, Lindemann A. Characterization of the thermophyscial properties of molten polymers and liquids using the flash technique. High Temp High Press 2003;35:627–32
- De la Cruz G, Gurevich Y. Thermal diffusion of a two-layer system. Phys Rev B 1995;51:2188
- Wanka G, Hoffman H, Ulbricht W. Phase diagrams and aggregation behavior of poly (oxyethylene)- poly (oxypropylene)- poly (oxyethylene) triblock copolymers in aqueous solutions. Macromolecules 1994;27:4145–59
- Kim Y-S, Rhim H, Choi D, Lim HK. Does artificial ascites induce the heat-sink phenomenon during percutaneous radiofrequency ablation of the hepatic subcapsular area? an in vivo experimental study using a rabbit model. Korean J Radiol 2009;10:43–50
- Dewhirst M, Viglianti B, Lora-Michiels M, Hanson M, Hoopes P. Basic principles of thermal dosimetry and thermal thresholds for tissue damage from hyperthermia. Int J Hyperthermia 2003;19:267–94
- Nikfarjam M, Muralidharan V, Christophi C. Mechanisms of focal heat destruction of liver tumors. J Surg Res 2005;127:208–23
- Dumortier G, Grossiord JL, Agnely F, Chaumeil JC. A review of poloxamer 407 pharmaceutical and pharmacological characteristics. Pharm Res 2006;23:2709–28
- Jeong B, Kim SW, Bae YH. Thermosensitive sol-gel reversible hydrogels. Adv Drug Deliv Rev 2002;54:37–51
- Ruel-Gariépy E, Leroux J-C. In situ-forming hydrogels – review of temperature-sensitive systems. Euro J Pharm Biopharm 2004;58:409–26
- Sarkar N. Thermal gelation properties of methyl and hydroxypropyl methylcellulose. J Applied Polymer Sci 1979;24:1073–87
- Ruel-Gariepy E, Chenite A, Chaput C, Guirguis S, Leroux J-C. Characterization of thermosensitive chitosan gels for the sustained delivery of drugs. Int J Pharm 2000;203:89–98
- Moreland AJ, Lubner MG, Ziemlewicz TJ, Kitchin DR, Hinshaw JL, Johnson AD, et al. Evaluation of a thermoprotective gel for hydrodissection during percutaneous microwave ablation: In vivo results. Cardiovasc Intervent Radiol 2014;1–9. In press