Abstract
Planning of combined radiotherapy and hyperthermia treatments should be performed taking the synergistic action between the two modalities into account. This work evaluates the available experimental data on cytotoxicity of combined radiotherapy and hyperthermia treatment and the requirements for integration of hyperthermia and radiotherapy treatment planning into a single planning platform. The underlying synergistic mechanisms of hyperthermia include inhibiting DNA repair, selective killing of radioresistant hypoxic tumour tissue and increased radiosensitivity by enhanced tissue perfusion. Each of these mechanisms displays different dose-effect relations, different optimal time intervals and different optimal sequences between radiotherapy and hyperthermia. Radiosensitisation can be modelled using the linear-quadratic (LQ) model to account for DNA repair inhibition by hyperthermia. In a recent study, an LQ model-based thermoradiotherapy planning (TRTP) system was used to demonstrate that dose escalation by hyperthermia is equivalent to ∼10 Gy for prostate cancer patients treated with radiotherapy. The first step for more reliable TRTP is further expansion of the data set of LQ parameters for normally oxygenated normal and tumour tissue valid over the temperature range used clinically and for the relevant time intervals between radiotherapy and hyperthermia. The next step is to model the effect of hyperthermia in hypoxic tumour cells including the physiological response to hyperthermia and the resulting reoxygenation. Thermoradiotherapy planning is feasible and a necessity for an optimal clinical application of hyperthermia combined with radiotherapy in individual patients.
Introduction
Mild temperature hyperthermia (HT) in the range between 41–45 °C is applied as a radiosensitiser in combination with radiotherapy (RT) with excellent clinical results [Citation1,Citation2]. Treatment planning is essential to optimise the radiation dose and temperature delivered to the tumour while minimising the risk of normal tissue complications. Currently, treatment planning for these two modalities is always performed separately, without taking the synergistic action between the two modalities into account [Citation3–6]. Because of the synergy, treatment outcome strongly depends on the radiosensitising effect of the temperature distribution on the radiotherapy dose distribution. Thus, two separately optimised treatment plans will not necessarily result in an optimal plan for the combined treatment.
There have been efforts to predict clinical outcome of RT + HT treatment schedules using tumour control probability (TCP) data derived from randomised trials comparing RT ± HT. For example, Plataniotis and Dale [Citation7] derived the individual contributions for tumour control of radiotherapy, chemotherapy (CH) and hyperthermia from 11 randomised clinical trials comparing RT ± CH and RT ± HT for cervical cancer and then simply took the sum of these contributions to estimate the expected tumour control probability (TCP) for trimodality RT + CH + HT treatment. An objection to this approach is that it requires independent cell killing contributions from both RT and HT, which is not the case [Citation8]. Moreover, the clinical TCP values used are based on a large group of patients with unknown and variable tumour temperature distributions. This permits, at best, analysis of groups of patients with known average parameters (e.g. temperature during HT), but planning individual patients is not possible using a TCP-based approach.
Realistic clinical thermoradiotherapy planning (TRTP) requires modelling of the synergy between radiotherapy and hyperthermia for individual patients. Ideally all three mechanisms of radiosensitisation by hyperthermia suggested in the literature should be modelled: inhibition of DNA damage repair [Citation9–11], direct cell killing of radioresistant hypoxic tumour cells [Citation11–14] and reoxygenation by increased tissue perfusion [Citation15–19]. The contribution of each of these mechanisms to radiosensitisation is still a subject of debate and due to this complicated mix of synergistic effects the optimal treatment scheme for combined radiotherapy and hyperthermia is difficult to determine based on the present literature. Thus TRTP platforms at this stage utilise biological modelling based on present data but will get more accurate in due time. Advantages of using TRTP include the ability to optimise the combined RT + HT treatment and to predict the optimal treatment scheme. Optimal RT and HT dose distributions achieved by optimising RT and HT separately can differ significantly from RT and HT dose distributions when the combined RT + HT treatment has been optimised.
Adequate modelling of the cytotoxicity of hyperthermia and radiotherapy is a challenge. This work evaluates available experimental data on cytotoxocity of this combined treatment and the requirements for successful integration of hyperthermia and radiotherapy into a single thermoradiotherapy planning (TRTP) platform based on biological modelling.
Radiosensitisation
Biological modelling
Biological modelling is often applied in radiotherapy to calculate equivalent dose distributions and compare different fractionation schemes. For example, calculation of the equivalent dose in 2 Gy fractions for other fractionation schemes. The linear-quadratic (LQ) model is a robust mathematical model used for this purpose. It describes the cell surviving fraction (SF) after treatment:
where d is the fraction dose, D the total dose and α (Gy-1) and β (Gy-2) represent the radiosensitivity [Citation20–22].
The radiosensitisation effect by hyperthermia can be modelled as a temperature-dependent change in the radiosensitivity parameters α and β. In this way, the LQ model can be used to derive a conventional radiation dose d without hyperthermia, which is equivalent in biological effect to the actual effect of the combined radiotherapy and hyperthermia treatment [Citation23]. Given a 3D radiotherapy dose distribution and a 3D temperature distribution, this allows calculation of the 3D equivalent dose distribution for a combination treatment of radiotherapy and hyperthermia. The determination of adequate LQ parameters as a function of temperature quantification of the synergy between radiotherapy and hyperthermia is required.
Synergistic mechanisms of hyperthermia
Three synergistic mechanisms have been identified:
Inhibition of DNA damage repair: HT enhances the effectiveness of radiotherapy by inhibiting repair of DNA damage [Citation9–11],
Direct cell killing: HT selectively kills radioresistant hypoxic tumour cells [Citation11–14],
Reoxygenation: HT increases tissue perfusion resulting in reoxygenation, thereby reducing hypoxia and increasing radiosensitivity [Citation15–19,Citation24,Citation25].
All three mechanisms have their respective dose–effect relations, temporal effects and differential effectivity in normal and tumour tissue. These effects can be studied extensively in pre-clinical experiments such as survival curves of tumour cells treated with RT ± HT in in vitro studies and from control rates of experimental tumours treated with RT ± HT in in vivo studies.
Radiosensitisation as a function of thermal dose, sequence and time interval
The next sections evaluate available preclinical data on dose–effect relationships, effects of sequence and time interval between HT and RT on radiosensitisation in tumour and in normal tissue. These properties will be discussed in relation to the different synergistic mechanisms.
Dose–effect relationship
In vitro experiments show a very strong dose–effect relationship for hyperthermia with thermal dose doubling for every 0.5 °C temperature rise between 37 °C and ∼42.5 °C [Citation26–28]. Clinical results confirm the existence of a significant dose–effect relationship [Citation29–32]. The parameter cumulative equivalent minutes at 43 °C (CEM43 °C) was introduced by Sapareto and Dewey to express thermal dose [Citation26]. A review of CEM43 °C is presented by van Rhoon in this issue [Citation33]. A widely used parameter quantifying radiosensitisation by hyperthermia is the thermal enhancement ratio (TER). TER is defined as the ratio of the RT dose for RT alone divided by the RT dose for RT + HT reaching isosurvival for cell survival curves for RT ± HT in in vitro studies. Both in vitro and in vivo tumour models confirm the strong dependence of TER on both temperature level and duration of hyperthermia treatment [Citation34]. Hypoxic tumour tissue is reported to be more sensitive to hyperthermia than normally oxygenated tumours in similar in vitro studies [Citation11,Citation35].
Direct cell killing is responsible for part of the apparent radiosensitisation of hypoxic tumour tissue. A strong dose–effect relationship exists both for direct cytotoxicity of HT and for radiosensitisation due to inhibition of DNA repair by HT [Citation26–28].
The reoxygenation mechanism displays a different dose–effect relationship: blood vessels open up at circa 40 °C and shut down for temperatures >44 °C [Citation15,Citation36,Citation37]. Enhanced radiosensitisation by this mechanism is thus optimal during a mild temperature HT regime. The reoxygenation effect is reported to last for 24–48 h, even after perfusion levels have returned to normal after the end of the HT session [Citation36].
Sequence and time interval
TER is strongly dependent on the sequence and time interval between radiotherapy and hyperthermia in both in vitro and in vivo tumour models, as shown in for an example of a C3H mammary adenocarcinoma implanted in the hind limb of mice [Citation38]. The variation in TER observed in this figure can be explained by the presence of different synergistic mechanisms of hyperthermia.
Figure 1. The effect of sequence and interval between radiotherapy and hyperthermia on the thermal enhancement ratio (TER) for tumour (left) and skin (right) for C3H mammary adenocarcinoma implanted in the hind limb of mice, redrawn from [Citation38]. Part of the thermal enhancement in tumour tissue appears to be due to an additive effect of hyperthermia, this component appears to be absent in the thermal enhancement in normal skin tissue.
![Figure 1. The effect of sequence and interval between radiotherapy and hyperthermia on the thermal enhancement ratio (TER) for tumour (left) and skin (right) for C3H mammary adenocarcinoma implanted in the hind limb of mice, redrawn from [Citation38]. Part of the thermal enhancement in tumour tissue appears to be due to an additive effect of hyperthermia, this component appears to be absent in the thermal enhancement in normal skin tissue.](/cms/asset/9135e2bd-c7eb-4502-9389-228ed9e61626/ihyt_a_1110757_f0001_c.jpg)
Inhibition of DNA damage repair is a mechanism that is only effective when the cell is in the process of repairing DNA damage caused by RT, i.e. up to a few hours after RT. That is confirmed by experimental results which show that a time interval shorter than 4 h results in a significantly higher TER, reaching the highest TER when RT and HT are given simultaneously [Citation27,Citation38]. In the peak TER value for time intervals <4 h ranges between 1.5 and 2.5 for tumour tissue and TER ranges between 1 and 2.5 for skin tissue [Citation38] which has been confirmed for a range of tumours and normal tissues [Citation11,Citation39,Citation40]. Repair of DNA damage takes up to a few hours, so the increase in TER for time intervals <4 h is likely to represent radiosensitisation by blocking DNA repair in both tumour and normal tissue.
Direct cell killing is also visible in : note that the TER ≈1.5 for the tumour when HT is given 24 h after RT. Repair of DNA damage takes only a few hours and reoxygenation 24 h after RT is unlikely to have any effect. Any contribution to TER at this long time interval must therefore represent a mechanism independent of RT, such as killing of hypoxic tumour cells [Citation14]. The extent to which direct cell killing contributes to TER will depend on both the temperature level and the presence and size of the hypoxic fraction, which will vary per tumour type. Variable hypoxic tumour fractions would explain why experiments in some tumour models do not show the presence of such an additive component to the TER, e.g. Horsman et al. [Citation41]. Hypoxia as explanation for this part of the tumour effect could also explain why the TER ≈ 1.0 in skin tissue for RT 24 h before or after HT as hypoxia is generally absent in normal tissue. Note that a time interval of 1–4 h between RT + HT appears to be tumour selective with a significantly higher TER in tumour than in skin, as opposed to simultaneous RT + HT which yields TER ≈ 2.5 in both tumour and skin. Studies for a range of tumours and normal tissues confirmed that sequential RT and HT is more tumour selective than simultaneous RT + HT [Citation42].
Reoxygenation has a different impact on optimal sequence and interval. This mechanism is effective when HT is given prior to RT. Compared to the other two mechanisms the time interval is less critical since the reoxygenation effect is reported to last for 24–48 h, even after perfusion levels have returned to normal after the end of the HT session [Citation36]. Due to the long time over which the reoxygenation mechanism remains effective, a HT session will boost the RT session of the same day but also the RT session 1–2 days thereafter.
Thermotolerance, a temporary resistance to subsequent HT treatments lasting ∼2 days after hyperthermia is another phenomenon adding to the complexity of timing of HT and relevant for TRTP. The onset, duration and degree of thermotolerance depend among others on temperature and heating time [Citation43–45].
shows a schematic comparison of the differences in dose–effect relationship and optimal sequence with RT for the three mechanisms. Reoxygenation favours a milder temperature than the other two mechanisms. The time interval between HT and RT does not play a role for direct cell killing, but a schedule aiming at optimal synergy should use a short time interval to maximise inhibition of DNA damage repair and achieve optimal radiosensitisation.
Normal tissue thresholds
As discussed in the previous paragraph, hyperthermia blocks DNA repair and causes radiosensitisation in normal tissue depending on the sequence and time interval, but sensitisation is less than in tumour tissue. Since hypoxia is absent in normal tissue, direct cell killing and reoxygenation generally do not play a role. Normal tissue damage by direct cell killing can occur when the temperature exceeds ∼44 °C, therefore normal tissue hotspots should be avoided during clinical hyperthermia. Moritz and Henriques [Citation46] introduced the concept of thermal damage arising after exceeding time–temperature level thresholds based on an Arrhenius formulation to describe the development of skin burns in pigs. Pearce [Citation47] discusses mathematical modelling of the underlying molecular damage processes. The thermal damage threshold concept has been validated in many animal studies [Citation48]. A review given by Yarmolenko et al. [Citation49] lists thermal damage thresholds showing that exposure to 43 °C for 1 h causes no major damage for many relevant normal tissue types. Direct toxicity by HT alone is thus tumour selective, since it occurs for normally oxygenated tissues at high temperatures (T > 44 °C) compared to the mild hyperthermia in the tumour (40 °C < T < 43 °C) used in combination with radiotherapy and chemotherapy. Since the TER in normal tissue strongly depends on the sequence and time interval, the normal tissue thresholds for combined radiotherapy and hyperthermia treatments can be different, and application of these literature values should be handled with caution, particularly when the time interval between RT and HT is very short. This issue requires further research.
Development of thermoradiotherapy planning
Modelling of HT mechanisms using the LQ model
The HT mechanisms from the previous section need to be modelled in a practical way in the LQ model to ensure successful incorporation in TRTP. Part of the necessary quantitative radiosensitisation data can be retrieved from cell survival curves for RT ± HT. TER values from in vitro RT ± HT studies are widely available but are unfortunately not suitable for equivalent dose calculations as they represent just one point of the cell survival curve. We need α and β values at clinically relevant temperature levels and for clinically relevant time intervals, which requires fitting cell survival curves to the LQ model. For normal fractionated RT the LQ model in its standard form can be used and in a semi-empirical fashion to ensure robustness, which means that input of data measured over a wide range of different RT + HT temperatures, sequences and intervals is required. Whereas TER values have been frequently reported, LQ parameters under HT conditions are less readily available and if they are available often just for a single temperature level instead of the full hyperthermic temperature range. Xu et al. found in vitro that hyperthermic radiosensitisation can be modelled as a change in the α parameter [Citation50], and Myerson et al. used this result in a prospective study to analyse the thermal dose escalation for 60 patients treated with simultaneous hyperthermia and radiotherapy [Citation51]. A review of available LQ data under HT conditions is given by Franken et al. [Citation52,Citation53]. These data represent the mechanisms of inhibition of DNA damage repair and of direct cell killing. Note that these data do not allow accurate modelling of reduction of the hypoxic fraction by direct cell killing, since hypoxic cell lines were not included. Furthermore, the effect of reoxygenation on the LQ parameters requires the presence of a physiological response to heat and can therefore only be determined from in vivo experiments.
To prevent normal tissue toxicity the literature provides radiation dose limits and temperature limits. Since the thermal enhancement of normal tissue strongly depends on the sequence and time interval, the equivalent dose for the combined treatment is necessary to evaluate the risk on normal tissue complications. Including normal tissue effects in TRTP also requires in vivo experiments to derive LQ parameters for normal tissue as a function of temperature, sequence and time interval.
When distinguishing between the three mechanisms, blocking DNA repair represents the dominant form of radiosensitisation in normally oxygenated tumour tissues. LQ parameters derived from in vitro experiments generally reflect this mechanism for normally oxygenated tumour cells. Therefore, the first generation of TRTP models should account for this mechanism. The LQ model is routinely used for RT planning with consensus about LQ parameters to be used for normally oxygenated tumour and normal tissue. A TRTP platform using similar normally oxygenated tumour and normal tissue data would thus be a first logical step and will be readily accepted by the RT community ().
Figure 3. Schematic representation of the requirements for thermoradiotherapy planning (TRTP). The first generation of TRTP software should incorporate the DNA repair inhibition mechanism. To improve reliability, later generations should be extended with direct cell killing and reoxygenation.
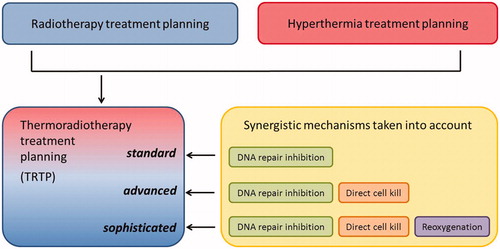
Implementation of the other two mechanisms, direct cell killing and reoxygenation, is more challenging. These mechanisms represent two different ways of reducing and/or sensitising the hypoxic fraction of the tumour by hyperthermia. The most elegant and logical method of modelling the action of both mechanisms is again the LQ model. More comprehensive variants of the LQ model are described in the literature, including the possibility to account for reoxygenation [Citation54]. However, additional parameters increase the complexity and would lead to an impractically large number of experiments needed to derive parameter values for the hyperthermic temperature range and various time intervals with sufficient accuracy. A more practical approach is to characterise radioresistance in hypoxic tissue by altered α and β values, particularly with a significantly lower α. In a review of the literature on 12 different human aerobic and hypoxic prostate cancer cell lines α was reported to range between 0.06 and 0.68 Gy−1 [Citation55]. The effect of hyperthermia can be modelled as a reduction of the hypoxic fraction, that is the fraction with hypoxic α and β. This should be possible if both aerobic and hypoxic LQ parameters are available; the main challenge of modelling these two mechanisms is then a realistic computation of the reduction of the hypoxic fraction. Two compartment models (aerobic and hypoxic) have been proposed for radiotherapy planning [Citation56]. Clinical TCP curves for canine oral carcinoma treated with RT ± HT showed an increased steepness for the TCP curve for RT + HT compared to the TCP curve for RT alone [Citation57]. A logical explanation would be that HT reduces the hypoxic fraction. Modelling reoxygenation involves estimation of the initial size of the hypoxic compartment followed by estimation of the reduction in size after HT. The latter is challenging, as the response of tumour tissue to hyperthermia is likely to vary widely. Imaging of the hypoxic fraction before and after HT will be necessary to provide data on the hypoxic status.
Incorporation of hypoxia in biological modelling is a challenging aspect and even in RT planning this is still the subject of research. Therefore, it is a logical choice to start TRTP with implementation of the first mechanism: inhibition of DNA damage repair. Since inhibition of DNA damage repair is very sensitive to the time interval, inclusion of this mechanism is crucial for optimising combined RT + HT treatment. Moreover, the available data are quantitatively more reliable than for the other mechanisms and will give appropriate results for normally oxygenated tumours and for normal tissue, but may underestimate the effectiveness of hyperthermia in tumours with a sizeable hypoxic population.
Imaging
The imaging and segmentation techniques required for thermoradiotherapy planning are the CT and MR techniques already routinely used in radiotherapy planning and hyperthermia planning. Accurate TRTP requires a correct mapping of the temperature distribution to the radiotherapy dose distribution. A major challenge for TRTP is the need to merge HT and RT imaging sets acquired in different supine/prone patient positions. This requires reliable deformable image registration to match these data sets.
Additional imaging is necessary to visualise the hypoxic fraction in the tumour and include this in TRTP. Hypoxic fractions can be identified by determining the uptake of a hypoxia PET tracer (e.g. F-18 Fluoromisonidazole (FMISO)) [Citation58–60]. Another non-invasive but indirect method to establish hypoxia is blood oxygenation level-dependent (BOLD) MRI, a method based on the direct relationship between changes of deoxyhaemoglobin in capillary and venous blood and the transverse relaxation times T2 and T2* [Citation61]. Signals are relative, and the relationship with intracellular hypoxia is not strong. Variations in the oxygen pressure (pO2) can be determined, which should suffice to visualise the hypoxic fraction of the tumour.
Example
Recently a treatment planning module has been developed based on the approach described above, modelling inhibition of DNA damage repair. This program quantifies hyperthermic radiosensitisation by using the LQ model with temperature-dependent α and β values estimated from the literature to express the thermal dose-dependent radiosensitising effect [Citation23]. Thus, the radiotherapy dose distribution and the temperature distributions can be combined and converted to the equivalent RT-alone dose distribution for individual patients. This first example of a thermoradiotherapy planning system was applied in a simulation study for a group of 15 prostate cancer patients who had been treated with RT without HT. In this study the effect of adding HT to RT was evaluated using the AMC-4 regional HT device and assuming a 1-h time interval between RT and HT. The actual RT distribution given during therapy was integrated with a simulated HT distribution optimised by maximising the temperature in the tumour subject to temperature constraints in normal tissue. An example of RT ± HT planning for two prostate cancer patients is shown in . The conclusion was that adding hyperthermia is equivalent to a radiotherapy dose escalation of about 10 Gy, yielding a mean equivalent dose of 86 Gy compared to a mean dose of 76 Gy given with radiotherapy alone.
Figure 4. Example of a treatment planning with dose distributions for radiotherapy alone and equivalent dose distributions for combined radiotherapy and hyperthermia for two prostate cancer patients with a low (case 1) and a high median temperature (case 2) [Citation23]. The 95% isodose level gives better tumour coverage for RT + HT in both cases, particularly in the region close to the rectum.
![Figure 4. Example of a treatment planning with dose distributions for radiotherapy alone and equivalent dose distributions for combined radiotherapy and hyperthermia for two prostate cancer patients with a low (case 1) and a high median temperature (case 2) [Citation23]. The 95% isodose level gives better tumour coverage for RT + HT in both cases, particularly in the region close to the rectum.](/cms/asset/9828967d-4bed-4563-9402-4a24c4d2f477/ihyt_a_1110757_f0004_c.jpg)
The most recent application of this program was for three cervical cancer patients treated with RT + HT where a T50 ranging between 40.1 °C and 41.1 °C was estimated to result in a hyperthermic dose escalation of 7.3 Gy to 11.9 Gy in addition to the dose of radiotherapy alone consisting of 45 Gy external beam RT to the gross tumour volume + a 24-Gy brachy boost [Citation62].
Further research
The synergistic action of blocking DNA repair is increasingly well understood and can be successfully incorporated into future TRTP systems. A major limitation of TRTP is still the lack of reliable temperature-dependent α and β values, which are presently only partly available in the hyperthermic temperature range and only for a few tumour types. Further research is needed to expand the data set for normally oxygenated tumours and normal tissue to allow reliable TRTP. Furthermore, LQ parameters vary with sequence and time interval, and these data should be available to determine the optimal treatment scheme for the combination treatment of radiotherapy and hyperthermia.
For TRTP in more hypoxic tumours, direct cell killing and reoxygenation should be incorporated as well. The temperature-dependent α and β values used so far were derived from in vitro data under well oxygenated conditions and therefore underestimate the effect of hyperthermia in hypoxic tumour cells. Cell survival data from hypoxic cell lines subjected to RT ± HT can solve that issue. Modelling the physiological response to hyperthermia and the resulting reoxygenation is a very challenging aspect of TRTP. This will require in vivo experiments to derive LQ parameters. Moreover, clinical application then requires the distinguishing of the hypoxic fraction of the tumour by additional PET or MRI imaging to assign appropriate LQ parameters to hypoxic and aerobic fractions.
A challenge for hyperthermia treatment planning is that the energy absorption is very tissue-type dependent, the absorption distribution is thus more heterogeneous than in radiotherapy. Fortunately reliable simulation of energy absorption has become feasible by using well validated high resolution computational methods [Citation5] and by using reliable patient-specific input data based on tissue properties acquired by MR electrical properties tomography [Citation63]. A second challenge for hyperthermia treatment planning is that the resulting temperature also depends on heat removal, which is dominated by blood flow which fluctuates during treatment in response to hyperthermia [Citation6]. As this spatio-temporal fluctuation is hard to predict reliably in prospective planning, the concepts of online adaptive planning and retrospective planning have been introduced [Citation64]. An advantage of hyperthermia is that the thermal dose delivered is regularly measured during the 1-h treatment using temperature probes or with non-invasive MR thermometry. This permits online re-planning yielding adapted settings correcting the thermal dose distribution during the session itself [Citation65–68]. It also permits retrospective reconstruction of the given thermal dose distribution during each session, followed by re-planning the optimal thermal dose distribution for the remaining sessions based on the cumulative dose already given. Online and retrospective use of TRTP is thus expected to give more accurate results.
Future prospects and perspective
When TRTP has been realised it will prove useful in various clinical applications. One is optimisation of the RT and HT treatment scheme, i.e. the sequence, time interval and number of HT sessions and the fractionation schedule. HT sessions are normally added to an existing RT fractionation schedule which may not result in optimal synergy. Perhaps the RT dose should be different on the day HT is given. TRTP can also be used to achieve more robust dose margins for RT by using HT to enhance the effective RT dose in tumour tissue without enhancing toxicity to adjacent normal tissue, thereby reducing the risk of normal tissue complications. When the reoxygenation mechanism has been integrated in TRTP it becomes possible to focus heat delivery to hypoxic areas, which efficiently optimises the combined treatment.
TRTP may also prove useful in the clinical realisation of simultaneous RT + HT. This form of RT + HT is associated with much higher thermal enhancement ratios and tumour control than sequential RT + HT and thus makes optimal clinical use of the benefits of HT [Citation14]. Devising HT equipment capable of performing simultaneous RT + HT is challenging, as simultaneous RT + HT is associated with high TER values in normal tissue as well, potentially resulting in normal tissue damage around the tumour. Because the effect of HT is no longer tumour selective when given simultaneously with RT, an increase in therapeutic ratio can only be obtained when the volume where RT and HT are given simultaneously can be geometrically restricted to the macroscopic tumour itself. Superficial and intracavitary HT equipment suitable for simultaneous RT + HT has become available [Citation69–72] and is clinically applied [Citation73], but such a good delineation is challenging for HT at deep-seated tumour locations as heating in normal tissue in the vicinity of the heated tumour region cannot be avoided due to heat conduction and blood flow, even when well-controlled high resolution HT techniques are used. The tumour delineation must therefore come primarily from RT and image-guided radiotherapy techniques which permit a very precise dose control and TRTP could be used to devise an optimal combination of RT and HT treatment plans achieving high TER in tumour tissue while keeping TER in normal tissue low, even when RT and HT are given simultaneously.
The basic form of the LQ model has been criticised and therefore other radiobiological models have been proposed that incorporate radiosensitisation at a more fundamental level [Citation74,Citation75]. These more comprehensive radiobiological models could be incorporated in future TRTP systems; however, the strength of the robust design of the TRTP system described herein is that it utilises semi-empirical input parameters from the LQ model. This means that future versions can in principle be extended to include other combinations with radiotherapy too, e.g. chemotherapy, halogenated pyrimidines [Citation76] or poly(ADP-ribose) polymerase-1 (PARP1) inhibitors [Citation77]. The combination of hyperthermia and proton therapy [Citation78] can also easily be incorporated. Immune response is also known to be triggered by RT and by HT [Citation79]. Inclusion of this phenomenon in TRTP is worthwhile but challenging, as this effect also depends strongly on combination with other agents, and more research is needed to provide the dosimetry data needed for incorporation in TRTP.
Reliable TRTP may also guide further clinical studies in hyperthermia. In studies where dose escalation is proposed hyperthermia might prove to be a good alternative. The potential differences in effectiveness of HT-mediated vs. straightforward dose escalation can be estimated using TRTP. Furthermore, when reliable temperature-dependent LQ parameters are available for a variety of tumour sites, TRTP can be very useful to investigate the possible effectiveness of HT for different patient cohorts and thereby aid the design of promising feasibility studies to further expand the role of HT in clinical oncology. The ultimate goal would be estimation of TCP and normal tissue complication probability (NTCP) based on the computed dose escalation. However, this will be a great challenge since also in radiotherapy treatment planning reliable TCP and NTCP modelling is still the subject of research.
Conclusions
Thermoradiotherapy planning is feasible, and a necessity for a clinically optimal application of hyperthermia combined with radiotherapy in individual patients. The first planning tools have been developed to model the combined effect of radiotherapy and hyperthermia accounting for inhibition of DNA damage repair. Further realisation of a clinical TRTP system requires radiobiological data for a range of tumour types, temperatures, sequences and oxygenation conditions, as well as integration of the two other synergistic mechanisms: direct cell killing and reoxygenation. When reliable thermoradiotherapy planning has been realised, it will be of great clinical importance to optimise combined radiotherapy and hyperthermia treatments and to guide further clinical studies.
Declaration of interest
This research was supported by the Dutch Cancer Society grants UVA 2012-5393 and UVA 2012-5540. The authors alone are responsible for the content and writing of the paper.
References
- Datta NR, Gomez Ordonez S, Gaipl US, Paulides MM, Crezee H, Gellermann J, et al. Local hyperthermia combined with radiotherapy and/or chemotherapy: Recent advances and promises for the future. Cancer Treat Rev 2015;41:742–753
- Cihoric N, Tsikkinis A, van Rhoon G, Crezee H, Aebersold DM, Bodis S, et al. Hyperthermia-related clinical trials on cancer treatment within the ClinicalTrials.gov registry. Int J Hyperthermia 2015;31:609–14
- Kok HP, Wust P, Stauffer PR, Bardati F, van Rhoon GC, Crezee J. Current state of the art of regional hyperthermia treatment planning: A review. Radiat Oncol 2015;10:196
- Dobbs J, Barrett A, Morris SL, Roques T. Practical Radiotherapy Planning, 4th ed. London: Hodder Arnold, 2007
- Paulides MM, Stauffer PR, Neufeld E, Maccarini PF, Kyriakou A, Canters RA, et al. Simulation techniques in hyperthermia treatment planning. Int J Hyperthermia 2013;29:346–57
- Kok HP, Gellermann J, Van den Berg CA, Stauffer PR, Hand JW, Crezee J. Thermal modelling using discrete vasculature for thermal therapy: A review. Int J Hyperthermia 2013;29:336–45
- Plataniotis GA, Dale RG. Use of the concept of equivalent biologically effective dose (BED) to quantify the contribution of hyperthermia to local tumor control in radiohyperthermia cervical cancer trials, and comparison with radiochemotherapy results. Int J Radiat Oncol Biol Phys 2009;73:1538–44
- Crezee J, Barendsen GW, Westermann AM, Hulshof MC, Haveman J, Stalpers LJ, et al. Quantification of the contribution of hyperthermia to results of cervical cancer trials in regard to Plataniotis and Dale (Int J Radiat Oncol Biol Phys 2009;73:1538–1544). Int J Radiat Oncol Biol Phys 2009;75:634
- Kampinga HH, Dynlacht JR, Dikomey E. Mechanism of radiosensitization by hyperthermia (> or =43 °C) as derived from studies with DNA repair defective mutant cell lines. Int J Hyperthermia 2004;20:131–9
- Krawczyk PM, Eppink B, Essers J, Stap J, Rodermond H, Odijk H, et al. Mild hyperthermia inhibits homologous recombination, induces BRCA2 degradation, and sensitizes cancer cells to poly (ADP-ribose) polymerase-1 inhibition. Proc Natl Acad Sci USA 2011;108:9851–6
- Horsman MR, Overgaard J. Hyperthermia: A potent enhancer of radiotherapy. Clin Oncol (R Coll Radiol) 2007;19:418–26
- Nielsen OS. Effect of fractionated hyperthermia on hypoxic cells in vitro. Int J Radiat Biol 1981;39:73–80
- Suit HD, Gerweck LE. Potential for hyperthermia and radiation therapy. Cancer Res 1979;39:2290–8
- Overgaard J. The heat is (still) on – the past and future of hyperthermic radiation oncology. Radiother Oncol 2013;109:185–7
- Vujaskovic Z, Song CW. Physiological mechanisms underlying heat-induced radiosensitization. Int J Hyperthermia 2004;20:163–74
- Vujaskovic Z, Poulson JM, Gaskin AA, Thrall DE, Page RL, Charles HC, et al. Temperature-dependent changes in physiologic parameters of spontaneous canine soft tissue sarcomas after combined radiotherapy and hyperthermia treatment. Int J Radiat Oncol Biol Phys 2000;46:179–85
- Winslow TB, Eranki A, Ullas S, Singh AK, Repasky EA, Sen A. A pilot study of the effects of mild systemic heating on human head and neck tumour xenografts: Analysis of tumour perfusion, interstitial fluid pressure, hypoxia and efficacy of radiation therapy. Int J Hyperthermia 2015;31:693–701
- Brizel DM, Scully SP, Harrelson JM, Layfield L, Dodge RK, Chales HC, et al. Radiation therapy and hyperthermia improve the oxygenation of human soft tissue sarcomas. Cancer Res 1996;56:5347–50
- Sun X, Xing L, Ling CC, Li GC. The effect of mild temperature hyperthermia on tumour hypoxia and blood perfusion: Relevance for radiotherapy, vascular targeting and imaging. Int J Hyperthermia 2010;26:224–31
- Fowler JF. Development of radiobiology for oncology – a personal view. Phys Med Biol 2006;51:R263–86
- Fowler JF. The linear-quadratic formula and progress in fractionated radiotherapy. Br J Radiol 1989;62:679–94
- Barendsen GW. Dose fractionation, dose rate and iso-effect relationships for normal tissue responses. Int J Radiat Oncol Biol Phys 1982;8:1981–97
- Kok HP, Crezee J, Franken NA, Stalpers LJ, Barendsen GW, Bel A. Quantifying the combined effect of radiation therapy and hyperthermia in terms of equivalent dose distributions. Int J Radiat Oncol Biol Phys 2014;88:739–45
- Viglianti BL, Lora-Michiels M, Poulson JM, Lan L, Yu D, Sanders L, et al. Dynamic contrast-enhanced magnetic resonance imaging as a predictor of clinical outcome in canine spontaneous soft tissue sarcomas treated with thermoradiotherapy. Clin Cancer Res 2009;15:4993–5001
- Jones EL, Prosnitz LR, Dewhirst MW, Marcom PK, Hardenbergh PH, Marks LB, et al. Thermochemoradiotherapy improves oxygenation in locally advanced breast cancer. Clin Cancer Res 2004;10:4287–93
- Sapareto SA, Dewey WC. Thermal dose determination in cancer therapy. Int J Radiat Oncol Biol Phys 1984;10:787–800
- Sapareto SA, Hopwood LE, Dewey WC. Combined effects of X irradiation and hyperthermia on CHO cells for various temperatures and orders of application. Radiat Res 1978;73:221–33
- Dewey WC, Hopwood LE, Sapareto SA, Gerweck LE. Cellular responses to combinations of hyperthermia and radiation. Radiology 1977;123:463–74
- Dewhirst MW, Sim DA, Sapareto S, Connor WG. Importance of minimum tumor temperature in determining early and long-term responses of spontaneous canine and feline tumors to heat and radiation. Cancer Res 1984;44:43–50
- Oleson JR, Samulski TV, Leopold KA, Clegg ST, Dewhirst MW, Dodge RK, et al. Sensitivity of hyperthermia trial outcomes to temperature and time: Implications for thermal goals of treatment. Int J Radiat Oncol Biol Phys 1993;25:289–97
- Jones EL, Oleson JR, Prosnitz LR, Samulski TV, Vujaskovic Z, Yu D, et al. Randomized trial of hyperthermia and radiation for superficial tumors. J Clin Oncol 2005;23:3079–85
- Overgaard J, Gonzalez GD, Hulshof MC, Arcangeli G, Dahl O, Mella O, et al. Hyperthermia as an adjuvant to radiation therapy of recurrent or metastatic malignant melanoma. A multicentre randomized trial by the European Society for Hyperthermic Oncology. Int J Hyperthermia 1996;12:3–20
- van Rhoon GC. Is CEM43 still a relevant thermal dose parameter for hyperthermia treatment monitoring? Int J Hyperthermia 2015. (in press)
- Overgaard J. Formula to estimate the thermal enhancement ratio of a single simultaneous hyperthermia and radiation treatment. Acta Radiol Oncol 1984;23:135–9
- Kim SH, Kim JH, Hahn EW. The radiosensitization of hypoxic tumor cells by hyperthermia. Radiology 1975;114:727–8
- Song CW, Park H, Griffin RJ. Improvement of tumor oxygenation by mild hyperthermia. Radiat Res 2001;155:515–28
- Song CW. Effect of local hyperthermia on blood flow and microenvironment: A review. Cancer Res 1984;44:S4721–30
- Overgaard J. Simultaneous and sequential hyperthermia and radiation treatment of an experimental tumor and its surrounding normal tissue in vivo. Int J Radiat Oncol Biol Phys 1980;6:1507–17
- Myers R, Field SB. The response of the rat tail to combined heat and X rays. Br J Radiol 1977;50:581–6
- Field SB, Bleehen NM. Hyperthermia in the treatment of cancer. Cancer Treat Rev 1979;6:63–94
- Horsman MR. Therapeutic potential of using the vascular disrupting agent OXi4503 to enhance mild temperature thermoradiation. Int J Hyperthermia 2015;31:453–9
- Marino C, Cividalli A. Combined radiation and hyperthermia: Effects of the number of heat fractions and their interval on normal and tumour tissues. Int J Hyperthermia 1992;8:771–81
- Nielsen OS. Fractionated hyperthermia and thermotolerance. Experimental studies on heat-induced resistance in tumour cells treated with hyperthermia alone or in combination with radiotherapy. Dan Med Bull 1984;31:376–90
- Nielsen OS, Overgaard J, Kamura T. Influence of thermotolerance on the interaction between hyperthermia and radiation in a solid tumour in vivo. Br J Radiol 1983;56:267–73
- Overgaard J, Nielsen OS. The importance of thermotolerance for the clinical treatment with hyperthermia. Radiother Oncol 1983;1:167–78
- Moritz AR, Henriques FC. Studies of thermal injury: II. The relative importance of time and surface temperature in the causation of cutaneous burns. Am J Pathol 1947;23:695–720
- Pearce JA. Comparative analysis of mathematical models of cell death and thermal damage processes. Int J Hyperthermia 2013;29:262–80
- Nadobny J, Klopfleisch R, Brinker G, Stoltenburg-Didinger G. Experimental investigation and histopathological identification of acute thermal damage in skeletal porcine muscle in relation to whole-body SAR, maximum temperature, and CEM43 °C due to RF irradiation in an MR body coil of birdcage type at 123 MHz. Int J Hyperthermia 2015;31:309–20
- Yarmolenko PS, Moon EJ, Landon C, Manzoor A, Hochman DW, Viglianti BL, et al. Thresholds for thermal damage to normal tissues: An update. Int J Hyperthermia 2011;27:320–43
- Xu M, Myerson RJ, Straube WL, Moros EG, Lagroye I, Wang LL, et al. Radiosensitization of heat resistant human tumour cells by 1 hour at 41.1 °C and its effect on DNA repair. Int J Hyperthermia 2002;18:385–403
- Myerson RJ, Roti Roti JL, Moros EG, Straube WL, Xu M. Modelling heat-induced radiosensitization: Clinical implications. Int J Hyperthermia 2004;20:201–12
- Franken NA, Oei AL, Kok HP, Rodermond HM, Sminia P, Crezee J, et al. Cell survival and radiosensitisation: Modulation of the linear and quadratic parameters of the LQ model (Review). Int J Oncol 2013;42:1501–15
- Franken NA, Hovingh S, Rodermond H, Stalpers L, Barendsen GW, Crezee J. Radiosensitization with chemotherapeutic agents and hyperthermia: Effects on linear-quadratic parameters of radiation cell survival curves. J Cancer Sci Ther 2011;S5:002
- Brenner DJ, Hlatky LR, Hahnfeldt PJ, Hall EJ, Sachs RK. A convenient extension of the linear-quadratic model to include redistribution and reoxygenation. Int J Radiat Oncol Biol Phys 1995;32:379–90
- Nahum AE, Movsas B, Horwitz EM, Stobbe CC, Chapman CC. Incorporating clinical measurements of hypoxia into tumor local control modeling of prostate cancer: Implications for the alpha/beta ratio. Int J Radiat Oncol Biol Phys 2003;57:391–401
- Scott OC. Mathematical models of repopulation and reoxygenation in radiotherapy. Br J Radiol 1990;63:821–3
- Gillette EL, McChesney SL, Dewhirst MW, Scott RJ. Response of canine oral carcinomas to heat and radiation. Int J Radiat Oncol Biol Phys 1987;13:1861–7
- Wijsman R, Kaanders JH, Oyen WJ, Bussink J. Hypoxia and tumor metabolism in radiation oncology: Targets visualized by positron emission tomography. Q J Nucl Med Mol Imaging 2013;57:244–56
- Li F, Joergensen JT, Hansen AE, Kjaer A. Kinetic modeling in PET imaging of hypoxia. Am J Nucl Med Mol Imaging 2014;4:490–506
- Lin A, Hahn SM. Hypoxia imaging markers and applications for radiation treatment planning. Semin Nucl Med 2012;42:343–52
- Niendorf T, Pohlmann A, Arakelyan K, Flemming B, Cantow K, Hentschel J, et al. How bold is blood oxygenation level-dependent (BOLD) magnetic resonance imaging of the kidney? Opportunities, challenges and future directions. Acta Physiol (Oxf) 2015;213:19–38
- Crezee J, van Leeuwen CM, Oei AL, van Heerden LE, Bel A, Stalpers LJ, et al. Biological modeling of the radiation dose escalation effect of regional hyperthermia in cervical cancer. Radiat Oncol 2015;10 (in press).
- Balidemaj E, van Lier AL, Crezee H, Nederveen AJ, Stalpers LJ, Van den Berg CA. Feasibility of electric property tomography of pelvic tumors at 3T. Magn Reson Imaging 2015;73:1505–13
- Roemer RB, Cetas TC. Applications of bioheat transfer simulations in hyperthermia. Cancer Res 1984;44:S4788–98
- Weihrauch M, Wust P, Weiser M, Nadobny J, Eisenhardt S, Budach V, et al. Adaptation of antenna profiles for control of MR guided hyperthermia (HT) in a hybrid MR-HT system. Med Phys 2007;34:4717–25
- Stakhursky VL, Arabe O, Cheng KS, Macfall J, Maccarini P, Craciunescu O, et al. Real-time MRI-guided hyperthermia treatment using a fast adaptive algorithm. Phys Med Biol 2009;54:2131–45
- Rijnen Z, Bakker JF, Canters RA, Togni P, Verduijn GM, Levendag PC, et al. Clinical integration of software tool VEDO for adaptive and quantitative application of phased array hyperthermia in the head and neck. Int J Hyperthermia 2013;29:181–93
- Kok HP, Ciampa S, de Kroon-Oldenhof R, Steggerda-Carvalho EJ, van Stam G, Zum Vorde Sive Vording PJ, et al. Towards on-line adaptive hyperthermia treatment planning: Correlation between measured and simulated SAR changes caused by phase steering in patients. Int J Radiat Oncol Biol Phys 2014;90:438–45
- Moros EG, Penagaricano J, Novak P, Straube WL, Myerson RJ. Present and future technology for simultaneous superficial thermoradiotherapy of breast cancer. Int J Hyperthermia 2010;26:699–709
- Kosterev VV, Kramer-Ageev EA, Mazokhin VN, van Rhoon GC, Crezee J. Development of a novel method to enhance the therapeutic effect on tumours by simultaneous action of radiation and heating. Int J Hyperthermia 2015;31:443–52
- Stauffer PR, Arunachalam K, Craciunescu O, Diederich C, Juang T, Rossetto F, et al. Conformal microwave array (CMA) applicators for hyperthermia of diffuse chest wall recurrence. Int J Hyperthermia 2010;26:686–98
- Diederich CJ, Khalil IS, Stauffer PR, Sneed PK, Phillips TL. Direct-coupled interstitial ultrasound applicators for simultaneous thermobrachytherapy: A feasibility study. Int J Hyperthermia 1996;12:401–19
- Myerson RJ, Straube WL, Moros EG, Emami BN, Lee HK, Perez CA, et al. Simultaneous superficial hyperthermia and external radiotherapy: Report of thermal dosimetry and tolerance to treatment. Int J Hyperthermia 1999;15:251–66
- Vassiliev ON. Formulation of the multi-hit model with a non-Poisson distribution of hits. Int J Radiat Oncol Biol Phys 2012;83:1311–16
- Scheidegger S, Fuchs HU, Zaugg K, Bodis S, Fuchslin RM. Using state variables to model the response of tumour cells to radiation and heat: A novel multi-hit-repair approach. Comput Math Methods Med 2013;2013:587543
- Franken NA, Barendsen GW. Enhancement of radiation effectiveness by hyperthermia and incorporation of halogenated pyrimidines at low radiation doses as compared with high doses: Implications for mechanisms. Int J Radiat Biol 2014;90:313–17
- Eppink B, Krawczyk PM, Stap J, Kanaar R. Hyperthermia-induced DNA repair deficiency suggests novel therapeutic anti-cancer strategies. Int J Hyperthermia 2012;28:509–17
- Datta NR, Puric E, Schneider R, Weber DC, Rogers S, Bodis S. Could hyperthermia with proton therapy mimic carbon ion therapy? Exploring a thermo-radiobiological rationale. Int J Hyperthermia 2014;30:524–30
- Lauber K, Brix N, Ernst A, Hennel R, Krombach J, Anders H, et al. Targeting the heat shock response in combination with radiotherapy: Sensitizing cancer cells to irradiation-induced cell death and heating up their immunogenicity. Cancer Lett 2015;368:209–29