Abstract
The urinary bladder is a fluid-filled organ. This makes, on the one hand, the internal surface of the bladder wall relatively easy to heat and ensures in most cases a relatively homogeneous temperature distribution; on the other hand the variable volume, organ motion, and moving fluid cause artefacts for most non-invasive thermometry methods, and require additional efforts in planning accurate thermal treatment of bladder cancer. We give an overview of the thermometry methods currently used and investigated for hyperthermia treatments of bladder cancer, and discuss their advantages and disadvantages within the context of the specific disease (muscle-invasive or non-muscle-invasive bladder cancer) and the heating technique used. The role of treatment simulation to determine the thermal dose delivered is also discussed. Generally speaking, invasive measurement methods are more accurate than non-invasive methods, but provide more limited spatial information; therefore, a combination of both is desirable, preferably supplemented by simulations. Current efforts at research and clinical centres continue to improve non-invasive thermometry methods and the reliability of treatment planning and control software. Due to the challenges in measuring temperature across the non-stationary bladder wall and surrounding tissues, more research is needed to increase our knowledge about the penetration depth and typical heating pattern of the various hyperthermia devices, in order to further improve treatments. The ability to better determine the delivered thermal dose will enable clinicians to investigate the optimal treatment parameters, and consequentially, to give better controlled, thus even more reliable and effective, thermal treatments.
Introduction
Bladder cancer is the fourth most common malignancy among Western men [Citation1]. About 75% of patients present with non-muscle-invasive bladder cancer (NMIBC); NMIBC is confined to the mucosa and lamina propria (stages Ta, T1) and includes carcinoma in situ (CIS) [Citation2]. The other 25% of patients present with cancer invading the bladder musculature up to the perivesicular fatty tissue (MIBC). These are distinct diseases with very different outlook, treatment modalities, and hyperthermia requirements. Hyperthermia (HT) has been investigated for decades as a method to improve the results of bladder cancer treatments. Most trials have focussed on the treatment of NMIBC. In order to reduce recurrences, HT is used to enhance the efficacy of chemotherapeutic instillations. For about two decades, HT in NMIBC was performed mostly with the Synergo-system, an intracavitary radio-frequency (RF) device. The last few years, other heating approaches, introduced briefly in the section on delivering thermal dose, have been increasingly used to deliver a controlled thermal dose to the target bladder region.
There have been far fewer trials using HT for MIBC treatment. In this case the heat has to penetrate the entire bladder wall and part of the surrounding tissue, reducing the number of suitable HT devices and increasing the complexity of both the treatment and the determination of the delivered thermal dose. Since bladder and tissue properties are patient-dependent, heterogeneous, and time-varying, some form of thermometry during treatment is mandatory. As outlined in the section on hyperthermia below, which thermometry technique is most suitable depends on both the tumour type and the heating device. The current standard is to use minimally invasive local thermometry, but non-invasive regional thermometry methods are receiving increasing attention. Whereas hyperthermia treatment planning (HTP) and treatment simulation are not usually performed in intracavitary heating methods with shallow heat penetration – which may limit our options to optimise these treatments – multi-physics simulations are paramount to apply reliably deep HT treatments. An introduction to the available HTP systems is given in the section on temperature simulation, with special attention to the particular anatomical and physiological properties of the bladder.
The pros and cons of several options to heat the tumour, measure the temperature, and simulate the treatment are discussed in more general terms in the final section. We then summarise the unique properties of the bladder, in particular its accessibility, and its similarity, in physical terms, to a fluid-filled bag with internal convective flow and no significant heat loss due to blood perfusion. Finally we present recommendations for implementing good thermal dosimetry practices and interesting future research topics.
Bladder cancer
NMIBC and MIBC are clearly different tumour identities from both clinical, therapeutic, and thermal points of view. Whereas superficial tumours have an infiltration depth of less than about 20 cell layers (approximately 0.2 mm) and a very small risk of regional or distant disease, muscle-invasive tumours can infiltrate up to 2 cm from the bladder lumen, and have a high risk of metastasising. Therefore, MIBC generally needs a much more aggressive treatment, and a much greater penetration depth of the HT treatment than NMIBC.
Non-muscle-invasive bladder cancer
Standard treatment for NMIBC is transurethral resection (TUR) of all visible lesions. Progression into a muscle-invasive cancer (see the introduction above) is seen in 20–30%, and recurrence even in 50–75%, depending on the risk group of the tumour [Citation3].
To prevent tumour recurrence following TUR, immunotherapy or intravesical chemotherapy (CH) is given. In many cases, standard of care is immunotherapy with bacillus Calmette–Guérin (BCG), which can also influence the progression rate when given as maintenance therapy [Citation4,Citation5]. Unfortunately BCG is not without severe side effects, both local and systemic, which can even be life threatening [Citation6]. The most commonly used CH agent is mitomycin C (MMC), followed by epirubicin and doxorubicin, which are instilled into the bladder in single, multiple weekly and/or maintenance dose regimens.
To improve this, combination therapies with local HT have been introduced. A synergistic effect is seen in vitro when combining HT and MMC in different bladder cell lines [Citation7]. Phase I and II trials have been conducted with intracavitary RF hyperthermia (explained below). Toxicity seems to be acceptable although a thermal reaction of the mucosa frequently occurs [Citation8]. Only one randomised trial in intermediate/high risk patients has been conducted. After a follow-up of 10 years the disease-free survival was 53% versus 15% in favour of the combined arm with HT [Citation9]. Other local heating techniques have since been explored, such as intravesical conductive heating [Citation10] and locoregional phased array HT systems (see the following section); so far only case reports and pilot studies have been published, but all showed promising results with acceptable toxicity [Citation11,Citation12].
Muscle-invasive bladder cancer
Standard therapy for MIBC is a radical cystectomy [Citation13–15]. Survival and locoregional recurrence rates are, however, strongly dependent on tumour stage and general patient condition [Citation16].
At present, the most common bladder-preserving treatment for MIBC is combined radiotherapy (RT) and CH, which is generally only applied for unresectable tumours or patients medically unfit for cystectomy. However, long-term data show that overall survival is comparable when modern radiation techniques are used [Citation17–19]. The addition of sensitising CH (cisplatin, MMC and/or 5-FU) to RT in MIBC treatment has improved 5-year survival (from 35% to 48%) and reduced 2-year locoregional recurrence rates (from 32% to 18%) [Citation20]. However, considering the toxicity of CH for this generally older patient group, HT is an attractive alternative as RT sensitising agent for patients not eligible for CH. Only one randomised trial evaluated the additive effect of HT to RT in MIBC. Although the addition of deep regional HT increased the complete response rate with no increase of late toxicity, it did not significantly prolong the duration of local control and survival [Citation21]. Achieved intracavitary temperatures, which are not representative of temperatures in the deep infiltrating parts of MIBC, were recorded but not reported.
Furthermore, considering synergistic effects of HT with both ionising radiation and CH agents, a tri-modality treatment could further increase the local response rate of chemoradiation in MIBC. First results of a phase I–II study combining radiochemotherapy with deep regional HT in T1 and T2 bladder carcinoma showed encouraging results with a 3-year local recurrence-free survival of 85% [Citation22,Citation23]. This group uses non-invasive magnetic resonance imaging (MRI)-based temperature measurements (see the following section), but results of the temperature data have not been published.
Hyperthermia
Hyperthermia works in myriad ways, which depend on temperature and treatment duration (thermal dose), combination of treatment modalities, and tumour site. Mechanisms of HT include increased blood perfusion [Citation24–26], altered cell metabolism, activated apoptotic pathways [Citation27], increased drug uptake, inhibited DNA damage repair [Citation28–30], and activation of the immune system [Citation31,Citation32]. Each mechanism has a different thermal dose–effect relation, making thermal dosimetry a complicated parameter.
In some cases, concomitant HT may actually lower drug efficacy, as heating may degrade the drug. Fortunately, this appears not to be the case for MMC [Citation33].
Thermal dose
Thermal dose is a measure of the cumulative exposure of tissue to a specific time and temperature combination. The commonly used measure for thermal dose is cumulative equivalent minutes at 43 °C, or CEM43 [Citation34,Citation35], which is based on an Arrhenius relationship:
(1)
with dt (min) the time spent at temperature T (°C), and the break point R a constant usually taken to be ¼ for T < 43 °C and ½ for T > 43 °C. There are also indications that the actual values for R may be closer to ⅛ and ¾, respectively, and that the break point may be 47 °C rather than 43 °C [Citation36]. Also, it is uncertain over which temperature range this relation holds. Hyperthermia treatment temperatures are typically in the range 39–45 °C, as temperatures lower than 39 °C are mostly ineffective and temperatures higher than 45 °C may cause unwanted toxicities including immediately evident patient pain [Citation37,Citation38]. Note that dose–effect relationships differ for different tissue types: 10 CEM43 for the skin has a very different effect than 10 CEM43 for muscle [Citation39]. Lastly, and most importantly, the dose definition in EquationEquation (1)
(1) is based on direct cell kill, which is generally not the main therapeutic action of HT. For example, blood perfusion appears to initially increase with temperature up until about 43.5 °C, above which perfusion will decrease because of vascular collapse [Citation24,Citation40,Citation41]; this affects reoxygenation and drug delivery, complicating the empirical formulation of a thermal dose. Nevertheless, there is a fair body of evidence that CEM43 works reasonably well as a measure for thermal dose [Citation35]; there is substantial clinical experience with CEM43 and no better substitute is available [Citation42].
Another point to note is that temperature is a local quantity, i.e. a value at a single point, while physiological effects are associated with a regional volumetric scale. To describe temperature throughout a treatment volume, temperatures are typically given as T90, T50, T10, or a combination thereof, which are the temperatures reached in at least 90%, 50%, and 10% of the target volume during treatment, respectively. This implies that tumour temperatures should be known with reasonable accuracy and sampling density throughout the targeted volume; this is currently challenging for both invasive and non-invasive thermometry techniques and consequently T50 can generally be established with more certainty than T90. These measures can be combined with CEM43 to give corresponding thermal dose measures within the tumour volume. The minimal or average T90 or T50 over the treatment or even over all treatment sessions may also be given. Other measures for thermal dose have been suggested but such alternatives have not been widely adopted. For instance, Franckena et al. [Citation43] used Trise, defined as the realised T50 over both tumour and surrounding healthy tissues, time averaged over the intended treatment duration. For cervical cancer Trise, performed comparably to CEM43 T90 in predicting disease specific survival.
On a final note, also the timing of the HT treatment – before, after or concomitant with the primary treatment modality – and the time interval between HT treatments, have noticeable impact on treatment effectiveness and safety. As there is no widely accepted way to include HT timing in the thermal dose definition due to the complex synergy with the primary modalities, it will not be discussed in this paper [Citation44,Citation45].
Quality control
Since even a mild temperature change has significant effect on physiology, temperature control during HT treatments is paramount to assure therapeutic quality independent of target region [Citation38,Citation46,Citation47]. This is especially true for bladder treatments where the device used and the tumour type above all determine thermal distributions. On the one hand the bladder fluid can be heated relatively easily (using a variety of techniques described below) and its temperature will be relatively uniform due to convective mixing. This generally also causes the bladder wall to be heated fairly homogeneously due to conduction (unless the heating pattern is highly heterogeneous, cf the section on intravesical microwave antennae). On the other hand the ion concentration inside the urine can vary greatly between patients, or even between treatments, leading to a high uncertainty in dielectric properties – highly relevant for RF heating – and hence to large inter-treatment and inter-patient variability. Moreover, the flow inlet and outlet of the bladder, and in some cases of the chemotherapeutic solution, can cause local temperature changes.
Hyperthermia treatments usually last for 45–90 min (with invasive treatments typically lasting shorter than non-invasive ones), and during this time the volume of the bladder increases linearly [Citation48] or varies due to the constant drain through a urinary catheter. This is an important point for simulations of bladder HT treatments in particular, and consequently for heating methods that require treatment planning. When bladder volume is not controlled through a urinary catheter, treatment parameters as power, phase and amplitude have to be adjusted during treatment to ensure a homogeneous temperature rise in the bladder. Since a change in bladder filling may also cause significant changes to the neighbouring anatomy [Citation49,Citation50] this is also relevant for adjacent hyperthermia treatment sites such as the cervix [Citation21,Citation43].
The bladder has the advantage that intracavitary temperature measurements are feasible. However, it is quite difficult to position temperature probes in the bladder precisely and stationary. In contrast to other treatment sites, during bladder treatments temperature mapping by moving probes through catheters is not usually performed due to the need to clamp the urinary catheter [Citation51]. The temperature probe measures either the temperature of the bladder fluid or of the bladder wall. Therefore temperature measurement methods which measure both bladder wall and bladder fluid are recommended [Citation52,Citation53]. Phantom measurements showed that the temperature of the bladder filling and the bladder wall may differ by more than 1 °C, but this has yet to be verified in vivo.
The available procedures to adjust treatment settings to correct for too low or too high temperatures and ensure a high quality treatment differ considerably between heating devices.
Delivering thermal dose to the bladder
There are various technologies to deliver HT to the bladder. They differ in the way they heat the bladder wall, and consequently they have different heating patterns and different requirements regarding thermal modelling, thermal dosimetry, and treatment control. We briefly introduce the most widely used methods as well as methods currently under investigation. A comprehensive review of heating technology is given elsewhere in this special issue [Citation54].
Heating methods can be divided between invasive (the first two methods below) and non-invasive (the last three) methods. Invasive systems heat only a shallow depth into the bladder wall, limiting their use to non-muscle-invasive disease. Non-invasive RF heating systems extend heating deeper into the bladder wall and surrounding tissues, potentially enabling MIBC treatment and providing an additional challenge for adequate thermometry, but also offering more options for intracavitary thermometry. A combination of two systems, e.g. external phased array RF heating with recirculating warm fluid, may combine some advantages of both systems.
Recirculating heated fluids
The simplest method to deliver heat to the bladder is based on closed-circuit recirculation of an externally heated chemotherapeutic solution. The high flow rates of these systems (200–300 mL/min) ensure good mixing of the bladder contents, resulting in a very homogeneous temperature in the lumen and at the bladder wall surface [Citation55]. Since the temperature of the fluid and the bladder inner surface is well known, there is no real need for treatment planning. However, with this thermal conduction heating approach, there is no direct measurement of the bladder wall temperature, and the estimated penetration depth across the wall is less than 4 mm, limiting its use to NMIBC. A recent porcine trial using the COMBAT system measured the temperature on both the internal and external surfaces of the bladder wall, employing thermistors (see following section). The results demonstrate that spatial distribution of the temperature on the bladder surface is relatively uniform (<0.4 °C), but the thermal gradient across the wall can reach 2–3 °C. The clinical introduction is discussed elsewhere in this special issue [Citation56].
Intravesical microwave antenna
The Synergo system (Medical Enterprises, Amsterdam, the Netherlands), in use since 1991 [Citation57], has the longest track record with respect to HT-enhanced MMC treatments for NMIBC. The system heats the bladder wall via 915 MHz microwave radiation from an intracavitary antenna with recirculating cooled chemotherapeutic solution, cooling the urethra around the hot microwave antenna feedline to prevent thermal toxicities.
Positive results have been shown in both ablative and prophylactic treatments for intermediate and high-risk NMIBC patients [Citation9]. Non-thermal side effects have been reported to be similar to standard MMC [Citation8]. Continuous operator control is required to keep the temperature at the desired level of 42 ± 2 °C [Citation58]. Since the radiation pattern produced by the antenna is non-uniform [Citation57,Citation59], the system may cause hotspots and superficial burns in about 40% of cases [Citation8], or potentially ineffective cold spots. These may not be detected by the limited integrated thermometry which consists of three to five thermocouple sensors (0.2 °C accuracy), pressed against the bladder wall. The reported temperature is usually the average of the three sensors, and variations per sensor during treatment are typically not more than 1.0–1.5 °C. Patient-specific HTP would be extremely challenging and is not applied. Heat penetration into the bladder wall is expected to be deeper than that of recirculation systems, but insufficient for MIBC; a study that investigated the temperature gradient over the bladder wall in a sheep model revealed that the difference between inside and outside temperature was 3.3–5.3 °C [Citation60].
For a more extensive introduction and an overview of all recent trials with this system, the reader is referred to Van Valenberg et al., elsewhere in this special issue [Citation58].
Capacitive deep hyperthermia
Capacitive heating works at relatively low RF frequencies of 5–30 MHz; all reported studies in treating bladder cancer used 8 MHz. Capacitive systems consist of two electrodes, placed on the anterior and posterior sides of the patient. Heating occurs primarily because of dielectric loss. Due to its high resistivity, a subcutaneous fat layer of more than about 2 cm is a contra-indication for treatment [Citation61].
Using electrodes of different sizes localises heating near the smaller electrode, but this form of power steering is very limited. Treatment simulation is still advisable because of the large influence of patient anatomy and bladder filling, and to evaluate possible temperature distributions. Although it is in general not possible to preferentially heat a deep tumour using a capacitive system, preferential heating of the bladder will occur naturally and can be further enhanced by increasing the salinity of the bladder contents to steer electric current through the lossy urine. Published studies show good temperatures obtained and good clinical results; an overview is given elsewhere in this special issue [Citation62].
Phased array deep hyperthermia
There are two commercially available phased array RF hyperthermia systems, operating at 70–120 MHz. These systems use either one or three rings of antennae around the patient. Phased-array systems generate a steerable heat focus, which can be shifted towards the target volume by adjusting power and phase of the signals. Patient-specific HTP is an important tool for the steering of phased array systems, particularly for the more complex multi-ring heating systems [Citation38,Citation63,Citation64].
In treatment planning the power and phase of each channel are optimised to maximise the heat delivery to the target and minimise hotspots above 45 °C in healthy tissue [Citation63,Citation65,Citation66], requiring not only accurate simulations and a treatment planning quality assurance, but also rigorous system characterisation [Citation63]. Power control during HT treatment with these systems is generally based on intraluminal temperature measurements [Citation67]. The BSD2000/3D/MR system uses 3D non-invasive MR-based temperature measurements during therapy [Citation68,Citation69].
Phased array deep HT devices have been used clinically for various treatment sites in the pelvic and abdominal area; recently, the feasibility of using these systems for NMIBC bladder HT has been proven [Citation11,Citation12]. This method has the potential to realise a more homogeneous thermal dose distribution than the intracavitary RF system, which should result in lower heat-related toxicity. Additionally, phased array systems have a much greater penetration depth, depositing energy in the entire bladder wall and the surrounding pelvic tissues, making it possible to induce better quality HT, particularly in MIBC cases. An overview of clinical results is given elsewhere in this special issue [Citation62].
Magnetic nanoparticle fluids
Investigation has begun on the use of ferromagnetic nanoparticle (MNP) solutions that, mixed with CH, can be instilled into the bladder. The MNPs are heated via magnetic induction coupling to an externally applied alternating magnetic field, usually at a frequency between 50–100 kHz to minimise direct heating of the pelvis. Pre-clinical studies have demonstrated that MNPs can produce sufficient heating inside the bladder [Citation70]. However, investigations continue to optimise nanoparticle and surfactant formulations to minimise toxicity of MNP solutions [Citation71].
Heat generation is distributed throughout the fluid, which, combined with convective mixing, helps to homogenise the internal bladder temperature. For magnetic coupled MNP solutions, temperature must be monitored with an intracavitary non-metallic or electromagnetic interference (EMI)-shielded probe. Unlike the circulating externally heated fluid approach, the maximum bladder temperature is not precisely known, as the intracavitary probe may underestimate a localised peak temperature in the unstirred magnetic fluid.
MNP will distribute well within the bladder producing relatively homogeneous temperatures throughout the bladder interior, though temperatures are not as uniform as for circulated fluid systems. Introduction of this technology into clinical practice awaits demonstration of lack of toxicity of the magnetic fluid when delivered into the bladder at a clinically effective concentration.
Temperature measurement
Precision and accuracy
Treatment outcome correlates to thermal dose in the target area for a variety of tumour types [Citation43,Citation72–84]. However, when temperatures exceed 44 °C, heat-related toxicity can occur [Citation37]. Thus, it is important to measure temperature accurately, reliably and in real time throughout and around the target area, i.e. the bladder wall and lumen. An accuracy of 0.2 °C and precision of 0.1 °C is preferable. Temperature should be recorded in real time to be able to adjust the power settings and optimise the temperature distribution during treatment.
It is important to have (invasive) temperature measurements not only in the bladder, but also in neighbouring tissues that receive heat, such as the urethra, and, for capacitive and phased array deep HT, the cervix and rectum. These measurements give more information about the temperature distribution in the pelvic area and provide feedback for steering the power focus into the bladder to maximise target dose and avoid excessive heating of normal tissues [Citation51]. It is important to provide feedback identifying the exact position of the heat focus within the patient [Citation51]; therefore, it is preferable to use either probes with multiple measurement points, multiple single point probes in the target area, to perform thermal mapping along the invasive catheter, or to use high resolution 3D thermal imaging [Citation38,Citation69,Citation85,Citation86].
If microwave or RF heating devices are used, temperature probes that do not interfere with EM fields are preferred (fibre-optic or high resistance lead thermistor probes). For sensors interfering with EM fields (i.e. thermistors and thermocouple probes), the power should be turned off during the temperature measurement [Citation47]. Accurate invasive probes are still required along with MR thermometry in hybrid MR-HT systems (see below).
Thermometry methods
Numerous methods are available to measure temperatures. Recently, a review was published for thermometry during thermal ablation [Citation87]; here, we focus on mild HT with special attention to the bladder. Generally, temperature is recorded most accurately with invasive probes such as thermistors, thermocouples, or fibre-optic sensors inserted into the bladder. For circulating heated fluids, the temperature in the bladder is mostly homogeneous and readily determined by any internal probe. The rapid fall-off of temperature within the bladder wall needs to be predicted by treatment planning or determined experimentally. For heating with an intravesical microwave antenna, the temperature distribution is more complex; thus, three thermocouple sensors are placed at the bladder wall at points of expected high and low field strength, providing a better definition of the heating pattern [Citation88–91]. Since the microwave antenna will deposit power non-uniformly directly in bladder wall tissue as well as the internal fluid, improved dosimetry would include volumetric thermometry techniques to characterise the 3D temperature distribution in a wider volume around the bladder. For heating with external RF systems, significant heating occurs regionally within the pelvis. Such treatments may be monitored with minimally invasive (intracavitary or intraluminal) probes in the bladder, vagina and rectum [Citation12,Citation51], but non-invasive thermometry supplemented by patient-specific simulations would provide more complete feedback on heating in and around the bladder and facilitate better treatment control.
To provide reliable feedback, invasive temperature probes (the first three techniques introduced below) must be placed securely at the point of interest, which may be challenging. The bladder is easily accessible via the urethra; however, the exact location of the probe remains difficult to determine. Spatial resolution of either technology may be better than 1 mm in the radial direction in the presence of steep gradients stretching up to about 5 mm in relatively homogeneous configurations, thus providing a point measurement that does not reflect the volumetric temperature pattern. If intravesical heating methods are used, the sensor needs to be incorporated into the (disposable) treatment set, as is the case with the Synergo system and the recirculating fluid external heat exchanger systems. The temperature of fluid inside the bladder is not necessarily the same as that of the bladder wall, and new approaches have been reported to characterise these temperatures. Using an ‘umbrella probe’ that presses sensors against the bladder wall, somewhat similar to the Synergo thermometry system, it has been shown in phantom studies that these indeed measured the bladder wall temperature, which was lower than the temperature of fluid filling the bladder lumen [Citation52]. Although it would be ideal from a dosimetric point of view, especially when treating MIBC, it is impossible to have temperature probes on each side of the bladder wall to ensure accurate characterisation of the thermal distribution, except in animal studies.
summarises the most important properties of each thermometry technique; they are discussed below in more detail.
Table 1. Characteristics of available bladder hyperthermia thermometry systems.
Thermocouples
Thermocouples are based on the Seebeck effect. A temperature gradient over a conductor results in a material-specific electric potential. Joining two different conductors results in a measurable voltage or current from which a temperature difference between both ends of the measurement wire can be deduced. The sensitivity and accuracy depend mostly on the materials used. For HT applications, type T (copper-constantan) thermocouples are widely used, as they have good precision in the HT temperature range. They are generally rugged, reliable, fast, and long-term stable, with a precision of 0.01–0.1 °C and, assuming proper calibration, an accuracy of better than 0.1 °C [Citation60,Citation92,Citation93]. Thermocouples have a fast response time and are generally thinner and less fragile than fibre-optic probes (see below), important in a clinical environment. Spatial resolution along the wire can be a problem [Citation94], especially when several thermocouples are combined in one probe, e.g. multi-sensor thermocouples with up to 14 sensors, spaced 0.5–1 cm apart [12,52,93]. This can be improved by using manganin-constantan probes, as they exhibit the same thermal sensitivity, but less thermal smearing than copper-constantan probes [Citation94]; however, they are less readily available.
When used in RF systems, thermocouples will interact with the EM field [Citation93]. Significant self-heating may occur, therefore the power needs to be switched off for 5 s so that self-heating artefacts can dissipate before each temperature measurement.
Thermistors
Thermistors are resistors whose resistance changes with temperature. The thermistor’s two terminations can be connected in several ways to readout circuitry: the most accurate has a four-wire configuration, two for driving the current and two for measuring the voltage thus compensating for non-negligible wire resistance. Negative temperature coefficient (NTC) thermistors are widely used in medical applications since they are inexpensive, accurate, and robust. Thermistors are generally made of a ceramic or polymer compound, which exhibit a resistance with non-linear temperature dependence. The Steinhart–Hart equation is a widely used third-order approximation [Citation95] valid over a very large range of temperatures (hundreds of °C) and for traditional ceramic sensors. When operated over a small temperature range (i.e. hyperthermia, 34–44 °C), thermistors offer a linear temperature behaviour.
Recent NTC thermistors are silicon (Si)- or germanium (Ge)-based and have significantly improved performance versus conventional ceramic NTC thermistors. Although Si/Ge thermistors have a logarithmic temperature/resistance curve, they have higher thermo-sensitivity and do not need individual calibration in the working temperature range (0.2% interchangeability). Additionally, smaller form factor Si thermistors can have resistance at room temperature of very few MΩ, so self-heating is negligible and the sensor is resilient to negative environmental conditions. A very high resistance wire (e.g. Kanthal 35 AWG, ∼1 Ω/cm) could be added to reduce interference from long wires without shielding or affecting performance. Conventional ceramic/polymer thermistors suffer from poor interchangeability, and self-heating.
Fibre-optic probes
Fibre-optic temperature sensors are based mainly on one of two technologies: fluoroptic and semiconductor band gap (SCBG) technologies. Fluoroptic technology takes advantage of optical properties inherent in phosphorescent materials. The instrument determines the temperature of the sensor by measuring the decay time of its emitted light. This decay time is a persistent sensor property and it varies precisely with temperature. Although fibres are typically reinforced with coating and can bend without affecting measurements significantly, the fluorescence material is often damaged with usage and time, limiting the life of the sensor. Alternatively, SCBG technology provides accurate measurements using the temperature-dependent bandgap shift of a gallium arsenide (GaAs) crystal.
Although fibre-optic sensors are expensive and fragile, they have a fast response and are immune to interference from RF and microwave sources. They are also electrically safe and resistant to chemicals and corrosion. Because of their ease of use (single-point calibration) and good accuracy (0.2 °C), they are used for a variety of thermal medicine applications. In bladder cancer therapy, fixed or moving fibre sensors are utilised for intracavitary monitoring of temperature (rectum, urethra/vagina) mostly in animal studies [Citation96] and as references for MR-guided treatments [Citation97].
Magnetic resonance imaging
Several non-invasive thermometry techniques for HT applications are under investigation, but the only method in clinical use is MR thermometry [Citation86,Citation98]. There are a number of temperature-sensitive MR parameters [Citation99], but MR thermometry in clinical HT is based on the proton resonance frequency shift (PRFS) method because of its wide temperature range, high linearity, good sensitivity and spatio-temporal resolution [Citation100]. The PRFS technique is based on comparing phase maps made during treatment with a baseline made before treatment [Citation101]. PRFS can be used in tissues with high water content (e.g. muscle), not in low water content tissues (e.g. fat and bone) [Citation102]. PRFS measurements have been shown to be an excellent tool to guide HT treatments in the pelvic region [Citation68] and extremities [Citation85].
MR thermometry requires the non-trivial design and installation of RF heating devices in an MR scanner. The first commercial solution, developed at Charité University Medical Center, showed the feasibility to integrate BSD Medical’s Sigma-Eye applicator into a 1.5 T MR scanner (Symphony, Siemens Healthcare, Erlangen, Germany) for treatments of tumours in the pelvis. They showed that quantitative MR measurements during heating are feasible for the pelvic region but also that susceptibility artefacts and the distortions by the applicator must be carefully taken into account for accurate MR measurements [Citation86,Citation103].
Although a very attractive option for dosimetry in the clinic, especially for MIBC, there are no reports concerning MR thermometry monitoring of bladder cancer treatments. There are a number of motion-related challenges to overcome, caused by filling of the bladder and bowel movements, for example. First, misalignment of mid-treatment from initial baseline images causes artefacts in the thermometry maps, which is not easily corrected in post-processing. Second, movement of tissue interfaces leads to changes in the main magnetic (B0) field of the scanner, invalidating some underlying assumptions of the reconstruction algorithm. For slow changes in the B0 field, techniques are under development to filter artefacts [Citation104,Citation105] or use a silicon [Citation106] or fat [Citation107] reference, but fast changes require dedicated motion-robust approaches. Finally, there are motion artefacts from convective currents or externally recirculated fluids inside the bladder that induce image distortion and temperature rise calculation errors. These distortions and errors may be overcome by masking signals from these locations, provided that the (moving) bladder volume is accurately located at all times. For MIBC treatment with RF devices, practical solutions may include catheter-based emptying of the bladder and means that restrict convection in the bladder.
To date, MR thermometry is the only technique that provides clinically proven 3D non-invasive temperature monitoring, but obtaining an accuracy of 0.1 °C requires additional technologies. Still, MR guidance has the added benefit of monitoring not only temperature but also the tumour.
Microwave radiometry
Every object radiates energy proportional to its absolute temperature with frequency distributed across the EM spectrum [Citation108]. Thermal radiation emitted at 0.5–5 GHz microwave frequencies can travel several cm through biological tissue [Citation109]. A microwave radiometer collects safely, painlessly and passively through an antenna, a number of these photons proportional to the tissue composition and absolute temperature. By collecting photons at different frequencies, it is thus possible to reconstruct the thermal profile across several cm of tissue below a sensing antenna placed on the skin [Citation110]. This skin contact requirement is a major drawback when the technique is to be combined with deep hyperthermia methods that require a water bolus between antenna and patient.
In general terms, a microwave radiometer consists of three basic modules: 1) a skin contacting sensor composed of a calibration switch system and a first stage amplifier, 2) a processing unit with high gain amplification, signal filtering and conversion to a digital signal, and 3) calibration, control and temperature reconstruction software [Citation111,Citation112]. The software provides signal processing with temporal averaging, drift compensation, and a signal-to-temperature conversion. Technological advances have resulted in radiometers able to track temperature 5 cm deep in the brain with a sensitivity of 0.4 °C. The same technology was used to demonstrate feasibility of simultaneous warming and temperature monitoring of porcine bladder [Citation113,Citation114].
For a single-band radiometer, there is just one signal and the specific tissue volume being sensed depends on the radiation pattern of the receive antenna and the selected frequency band, allowing temperature of a tissue volume to be accurately estimated at depth [Citation110,Citation115–117]. A temperature depth profile can be reconstructed if radiometer signals are collected over multiple carefully chosen frequency bands [Citation118,Citation119]. An array of radiometric sensors could then provide lateral information and, combined with depth profiles, produce 3D thermal mapping.
However, several challenges limit the use of this technology to lab research and preclinical models. Accuracy of the radiometric readings is highly dependent on antenna calibration, tissue properties, and patient anatomy. The calibrations generally involve a combination of both theoretical modelling and experimental confirmation in known heterogeneous tissue models [Citation110,Citation114,Citation120,Citation121]. Other critical factors for accurate radiometry are the contact reliability between sensor and tissue, motion artefacts, and EMI shielding. When combined with EM heating, accurate readings require turning off the power sources during the measurement period (e.g. 2–5 s power off intervals) potentially introducing a ‘cooling’ error. compares microwave and fibre-optic temperature measurements, showing a large delay for the radiometer.
Figure 1. Radiometric versus fibre-optic temperature. Bladder model: fluid is circulated from a temperature-controlled bath to a 30-mL Foley balloon located at 3.5 cm depth. The radiometer measures the ‘average’ bladder temperature, potentially missing extreme temperatures, whereas the fibre-optic probe measures at just a single point. Note the circa 5 min delay in the volumetric data from the radiometer versus the invasive fibre, located at the beginning of the bladder.
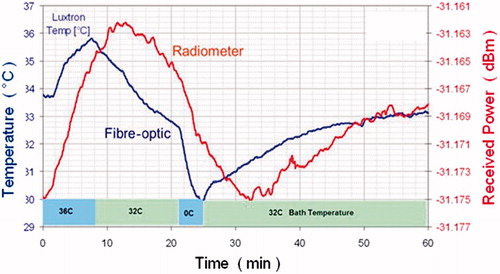
Although it ultimately could be a cost-effective technology, the thermal profile reconstruction is quite complex. Despite the challenges, radiometry has made significant advances in recent years. It may therefore soon play a role by complementing invasive measurements for thermal dosimetry during bladder HT, especially in those clinics where MR or ultrasound systems are not readily available.
Ultrasound
Ultrasound (US) imaging is capable of tissue thermometry using a variety of methods, covered comprehensively in a recent review article by Lewis et al. [Citation122]. US is real time and offers a spatial resolution of 1–2 mm. Challenges with US thermometry include the non-linear response to temperature changes, and a limited range of measurement from body temperature to less than 50 °C. Furthermore, compatibility of US devices with specific HT systems must be considered, both in terms of RF and acoustic interference, and the potential of US probes to disrupt energy transmission into the body. Patient movements and the fluid convection may also contribute to artefacts in the temperature measurements. To date, there are no reports in the literature of using US thermometry to monitor bladder heating, and the few reports of using the method in vivo have been limited to preclinical rodent studies [Citation123–125].
There are two US thermometry techniques with potential suitability for monitoring bladder treatments. The first is the use of speckle tracking or thermal strain imaging to detect speed of sound changes during heating [Citation126,Citation127]. This technique offers a temperature resolution of approximately 1 °C and is amenable to 2D B-scan imaging. Unaddressed issues include the robustness of the temperature calculations on a layered structure such as the bladder. Further, thermal strain methods are sensitive to bulk motion, requiring motion compensation or efforts to keep the bladder volume constant. The other promising approach is shear wave thermometry [Citation128], which measures the speed of propagation of a shear wave generated in the target tissue. Shear stiffness changes could be used to identify thermal dose levels as estimation of tissue damage [Citation129]. Moreover, this technique does not have the motion sensitivity of thermal strain imaging, making it more practical for bladder treatments.
Reliable clinical US systems are available in most of the urology clinics that perform cancer treatments. Advantages of US thermometry are portability, accessibility of the bladder to US, and ability to image the bladder wall clearly from outside the body or from transurethral systems. The fluid in the bladder is not likely to cause major artefacts; however, temperature measurements within the fluid are not feasible with US. Moreover, the motion of the bladder as it fills needs to be taken into account. Finally, depending on the heating method, the placement of the probe may be problematic. US thermometry is not yet standard on clinical systems, therefore applications in the near future are limited to research studies with prototype devices.
Temperature simulation
No HT treatment planning system currently in use accurately models the urinary bladder including convective flow, although some steps have been made to correct for this [Citation48]. Instead, the entire bladder, including contents, is typically modelled as a solid muscle. Thus two errors are introduced regarding the bladder content, since it is neither a solid, nor does it have muscle-like tissue properties. The resulting errors do not only affect the bladder itself, but may also have an impact on surrounding tissues. The presence of an air bubble within the bladder also appears to affect bladder wall temperature homogeneity [Citation130].
Clinical simulation methods
Hyperthermia treatment planning (HTP) for bladder treatments is used clinically almost exclusively for phased array systems (see above). For many years, HTP has been used mainly retrospectively [Citation131–133], but nowadays HTP is used more often before and during clinical HT to improve treatment quality [Citation51,Citation64,Citation134,Citation135]. The clinical relevance of HTP is emphasised in the recent quality assurance guidelines for clinical application of locoregional HT, specifying the requirements for HTP [Citation47]. The need for adequate treatment planning has led to a number of dedicated treatment planning software packages that can be used for clinical applications [Citation63,Citation66,Citation135].
All systems follow the same basic principle. Based on a segmented patient model, they first compute the EM field based on a catalogue of dielectric and thermal tissue properties, and calculate the resulting energy absorption, or specific absorption rate (SAR). Using this SAR distribution, they may compute a temperature distribution based on Pennes’ bio-heat equation [Citation136], which relates temperature change to energy uptake, thermal diffusion, and heat loss due to blood perfusion. The chosen modelled perfusion rate usually reflects the increased level expected at elevated temperatures. Many systems support temperature-dependent perfusion rates to reflect increases in perfusion during treatments, but these are not commonly used as they do not substantially improve the steady-state solution and negatively impact calculation times. Phase/amplitude optimisation can be done based on SAR or temperature objectives.
When locoregional heating is performed using one of the BSD Sigma systems [Citation137], the commercially available HTP system Sigma-HyperPlan (Dr Sennewald Medizintechnik, Munich, Germany) can be used. Besides the standard segmentation method, various other methods are available to generate a patient model based on a CT scan [Citation138]. E-field and temperature calculations can be performed using either the finite element method with tetrahedral grids or the finite difference time domain (FDTD) method [Citation139]. The FDTD method can be applied on a tetrahedral grid generated using segmented regions as well as on a voxel grid generated using the CT Hounsfield Units [Citation131].
SEMCAD X and Sim4life (SPEAG, Zurich, Switzerland) are commercially available multi-physics simulation platforms that are more flexible and allow modelling of various applicator configurations as well as MRI guidance and interactions. Add-on segmentation tool iSEG, which contains several fully automatic and interactive segmentation techniques, can be used to create patient models. Specific work flows are available for standardised HTP. Calculations are based on finite difference and finite element methods. Some extensions to the Pennes model are available, including user-defined anisotropic thermal conductivity, temperature dependent parameters and pseudo-1D boundary conditions to model the impact of vasculature and blood flow.
The AMC hyperthermia treatment planning system (Academisch Medisch Centrum, Amsterdam, the Netherlands) is a flexible in-house-developed FDTD-based HTP system that allows for various applicator systems [Citation140–142]. Tissue segmentation is based on Hounsfield Units, which can be combined with manual delineations and corrections. A unique feature of this planning system is the discrete vasculature (DIVA) thermal model, which allows realistic modelling of blood flow [Citation143,Citation144]. The DIVA model subdivides the model geometry into a voxelised tissue space and a grid-independent vessel space describing vasculature by 3D curves compatible with CT/MRI angiography software.
In addition to these dedicated planning software platforms, COMSOL, ANSYS HFSS high frequency structural simulator or CST STUDIO SUITE also allow for E-field calculations and basic thermal simulations for HTP. These packages provide flexibility in applicator modelling, but require linking to other software for tissue segmentation, advanced thermal modelling and optimisation of SAR or temperature distributions.
The software packages used to perform clinical HTP for bladder treatments differ between institutions, and the choice may depend on individual preferences. Nevertheless, additional software tools will be required for specific or relatively new applications, such as adaptive treatment optimisation.
Dielectric tissue properties
The accuracy of SAR and subsequent thermal modelling is dependent on the accuracy of the dielectric and thermal patient model, and thus on the quality of the tissue segmentation. In current HTP systems, the liquid contents of the bladder are assigned the same electrical and thermal properties as the bladder wall. The investigation of human autopsy samples of Gabriel et al. [Citation145,Citation146] resulted in a low permittivity of bladder wall tissue (ɛr,wall = 20) as well as a very low conductivity (σwall = 0.27 S/m) at 37 °C. This is much lower than the values typically used for muscle, to which the bladder wall is generally assumed to be similar. For instance, the database of Sigma HyperPlan lists a permittivity of ɛr,filled = 78 for bladder and a conductivity of σfilled = 0.6 S/m (both wall and contents) at a frequency of 90 MHz.
However, the dielectric properties of the fluids inside the bladder differ strongly from those of the bladder wall. Yuan et al. [Citation48] measured the dielectric properties of the treated bladder fluid (urine and MMC) at a frequency of 90 MHz and reported a permittivity equal to HyperPlan (ɛr,fluid = 78) but a much higher conductivity (σfluid = 1.81 S/m), similar to results in a porcine study [Citation147]. Given the ease of procedure, this type of measurement should be done more often to obtain a clearer insight into conductivity and permittivity value and variation. For tissues, however, human in vivo electric property measurements are scarce, due to practical and ethical reasons, and therefore MR based methods such as electric properties tomography (EPT) for non-invasive measurement have received increased attention [Citation148–152]. The resolution of such methods is currently inadequate to distinguish the bladder wall, but the bladder contents can be measured. A recent study using EPT in cervical cancer patients found a large interpatient variation: σfluid = 1.80 ± 0.54 S/m [Citation152], which had a major impact on HTP [Citation153]. The impact on the bladder is shown in .
Figure 2. Impact of tissue properties and fluid modelling on treatment simulation. (a) Current state of the art: current tissue properties, no convection. (b) With updated dielectric properties, but no convection. (c) With convective modelling, but current tissue properties. (d) With updated dielectric properties and convective modelling. All cases show the simulation using the AMC hyperthermia treatment planning system of a bladder hyperthermia treatment using clinically applied equipment settings, after 15 min of heating. Case (a) shows a moderately heated bladder with a slightly heterogeneous temperature distribution, based on the currently used simulation settings treating the bladder as solid muscle. Because of the higher electric conductivity, more power is deposited inside the bladder, leading to a higher temperature for case (b). Cases (c) and (d) show more homogeneous but lower bladder temperatures because of the more effective method of heat conduction, resulting in faster removal of heat from the bladder to the surrounding musculature, even for the higher power uptake in case (d). The difference in dielectric properties (left versus right) is explained in the dielectric tissue properties section, the important effects of convection (top versus bottom) in the section on thermophysical bladder properties.
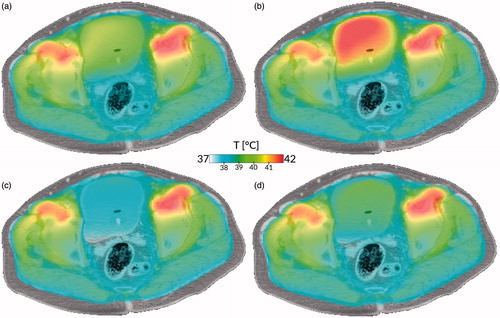
Moreover, electrical conductivity is temperature dependent; in a study with various freshly harvested tissues, the conductivity of bladder wall increased from 0.95 to 1.01 S/m and that of muscle from 1.03 to 1.13 S/m when the temperature was increased from 37 to 45 °C [Citation154]. Additionally, it has been shown that the electrical impedance increase of bladder wall tissue is affected by the presence of tumour tissue, an effect that does not occur in certain other tissues such as cervical tissue [Citation155].
There have been very few studies concerning the impact of using different dielectric values for bladder HTP. One group studied the combined effect of updated values of both dielectric and thermal parameters on HTP accuracy [Citation48]. A treatment planning study on cervical cancer patients found a large impact on HTP outcome using in vivo dielectric properties and bladder volume [Citation153]. In one patient model with a full bladder, the difference between optimisation with literature values and EPT values for the T90 was 1.37 °C; for patient models with practically empty bladders the temperature decrease was less than 0.57 °C [Citation153]. Although results for the cervix cannot be directly translated to the bladder, it suggests treatment with a full bladder may be beneficial.
Thermophysical bladder properties
Apart from the dielectric properties mentioned in the previous section there are two principal differences between a bladder properly modelled as a fluid-filled organ, and a bladder modelled as a solid muscle: the absence of perfusion, and the presence of convection. In local or locoregional HT, blood perfusion of tissues is a very important factor for temperature simulations [Citation156], and it is typically modelled as a heat sink. In the bladder interior there is no perfusion, and therefore no heat sink; instead, the only heat removal is through conduction to the (perfused) bladder wall.
Secondly, in an unevenly heated fluid, convection will occur under the influence of gravity. This is a much more effective heat transport mechanism than thermal conduction, and consequently it tends to homogenise temperature throughout the fluid. Additionally, there will be a net heat transport against the gravitational pull.
A first step to mimic the effects of convection is a model with highly increased effective heat transfer [Citation48]. This large effective heat transfer property should preferably be anisotropic, but not all HTP systems allow for that. A drawback of this approach is that the effective value for heat transfer depends on many factors and is not easily derived. Thus it is necessary to fit the effective conductivity to measured temperatures, making the method empirical and available only from the second treatment session onwards.
More recently, a physically more sophisticated and computationally more intensive approach was reported in a study investigating the effects on the temperatures of convection in the bladder, using a thermophysical fluid model extension to the AMC HTP system, the results of which are shown in [Citation130]. However, for good precision such a model requires the measurement of physical properties that are not routinely determined at this time, such as the viscosity of urine [Citation157]. While modelling convection is particularly important for HT treatments of the bladder (both for MIBC and NMIBC), it is also relevant for treatments of nearby organs such as the cervix, rectum and prostate, as significant temperature differences may occur in tissues up to 1.5 cm distance from the bladder [Citation48,Citation130].
Discussion
An increasing number of clinical studies using HT in the treatment of bladder cancer are being performed and results demonstrate very promising improvement in clinical outcomes. Thermal dosimetry is important to safeguard these treatments and to determine dose–effect relations. In this paper we describe the methods that are currently employed or that are being studied to determine thermal dose.
The urinary bladder is unique in the human body in that natural convection can occur in a large volume that affects temperature distribution in the surrounding pelvic region. In the context of HT treatment for bladder cancer, this has a number of advantages and disadvantages. Convection enhances homogenisation of temperature in the bladder contents and results in a similarly homogeneous thermal dose to the inner bladder wall. Treatment simulations [Citation130], phantom measurements [Citation52], and clinical experience [Citation158] all demonstrate a temperature variation of less than 0.5 °C along the bladder wall. This supports the concept that measuring a limited number of points inside the bladder is sufficient to obtain a good impression of the temperature of the entire bladder wall surface. An exception occurs when air is present in the bladder, shielding part of the bladder wall from contact with the bladder fluid, altering the local EM field, and potentially affecting the accuracy of non-invasive thermometry.
Another exception occurs during treatments with an intravesical antenna, in which the heterogeneous power deposition produces definite hot and cold spots within the bladder with temperature differences of several °C [Citation60,Citation91,Citation159,Citation160]. In the case of thermal conduction-based intravesical heating methods (e.g. recirculating heated fluids or MNPs), a steep temperature gradient occurs across the bladder wall. External heating methods have the potential to produce the most homogeneous temperature distribution and deepest heat penetration of the bladder wall, but may not produce equally high temperatures [Citation11,Citation12], especially when the patient anatomy is unfavourable [Citation51]. An interesting option is to combine an internal and external heating method, e.g. combining phased array RF heating with closed-circuit recirculation, especially in the context of MIBC where effective heat penetration and high thermal dose are essential.
However, convection currents or forced circulation of the bladder fluids have some important drawbacks for certain thermometry techniques, especially since the most promising non-invasive volumetric thermometry method, MRI, is very sensitive to motion. While restricting convection or emptying the bladder is, of course, impossible for NMIBC treatments with chemotherapeutic solutions, it could be an option for MIBC treatments that are combined with RT alone. However, in that case, one would also lose the preferential heating of the bladder and its homogenising effect on temperatures in the region.
Generally speaking, invasive thermometry technologies are more accurate and further developed than non-invasive methods. The accuracy and stability of thermocouple, thermistor, and fibre-optic-based probes is well established and the techniques are fully tested in a wide range of clinical applications. There are reports of promising clinical applications for emerging microwave radiometry [Citation110,Citation118,Citation120,Citation161], but most of the literature to date involves system development and feasibility testing. MR thermometry is more mature and is in clinical use, but accuracy is still insufficient for use as a stand-alone technique. For characterisation of bladder temperature during clinical HT, it is currently more logical to combine invasive sensors inside the bladder with external volumetric monitoring to improve knowledge of the temperature distribution extending through the bladder wall and potentially invading surrounding pelvic tissue. Given the current lack of knowledge of the temperature outside the bladder lumen, such combinations of accurate invasive methods, and more spatially informative non-invasive methods are essential.
Given recent progress in thermal modelling [Citation48], one can imagine further improved characterisation of pelvic temperature distribution using a combination of local thermometry, volumetric thermometry and patient specific simulations, as demonstrated for head and neck cancer [Citation162], for example, where measurements and simulations are combined into estimated temperature maps [Citation163]. One example of a hybrid simulation/optical probe approach recently demonstrated accurate assessment of the T50, based on sparse invasive probe measurements [Citation164], but the fusion of MRI and invasive measurements in the same coordinate space is still challenging [Citation165]. For bladder cancer, this technique might provide a good option to reconstruct the temperature within the bladder wall. Moreover, MR could potentially be used to map changes in tissue perfusion and dielectric properties, to be used as input for real-time treatment simulation, but this cannot currently be combined with MR thermometry.
Naturally, thermometry options are often limited by the specific heating technique used. In the case of recirculated fluids or intravesical microwave heating, the invasive thermometry options are determined by the manufacturer. In these cases, ultrasound or radiometric thermometry may provide additional information on the temperature distribution throughout the bladder wall. In the case of external heating methods, outside access to the patient may be limited by the presence of the HT device; but there is unrestricted access to the bladder through the urethra. In many cases, interference between the HT device and thermometry system may be an issue, but for most thermometry techniques this can be relatively easily solved by proper shielding or by temporarily turning the power deposition off during measurements. The exception is MRI thermometry, which typically requires a redesign of the devices with which it is combined.
Notwithstanding the technological challenges, accurate thermal dosimetry is highly relevant, both scientifically to gain insight into the heating properties of various HT devices and to study thermal dose–effect relations, and clinically to ensure good treatment quality.
Conclusion
For NMIBC treatment using thermal conduction HT devices, simple measurement of the fluid temperature is likely to be sufficient to control the delivered thermal dose, provided the penetration depth is well known and clinical efficacy has been proven in randomised controlled trials. For more complex treatment techniques, and for MIBC treatment, more elaborate methods are required to determine and control the thermal dose. Currently, none of the thermometry methods can by itself offer the required accuracy and spatial information. Although invasive probes are reliable and accurate, they deliver very limited spatial information; on the other hand non-invasive regional measurements provide volumetric thermal distribution data but suffer from various artefacts while failing to obtain the required accuracy. Finally, while multi-physics simulations provide important insight into possible temperature distributions from the various heating approaches, they need more accurate input in order to deal with inter-patient and inter-treatment variability. Therefore, combinations of at least two, but preferably all three dosimetry approaches are essential.
The fact that the urinary bladder is a fluid-filled organ is both a boon and a bane. On the one hand it makes the inside bladder wall relatively easy to heat and ensures in most cases a relatively homogeneous internal wall temperature distribution; on the other hand, the variable volume, organ motion, and moving fluid cause artefacts for most non-invasive thermometry methods, and necessitates additional physics considerations for accurate treatment planning.
Acknowledgements
The authors wish to thank Hans van Valenberg for his valuable input on the Synergo system. A.S.E. is a clinical adviser for Combat Medical Ltd. M.M.P. is a consultant and has a financial interest in Sensius BV. G.S. acknowledges financial support from the KWF Dutch Cancer Society [grant UVA 2012-5539]. M.M.P. acknowledges financial support from the KWF Dutch Cancer Society [grant EMCR2012-5472] and Technology Foundation Stichting Technische Wetenschappen (STW).
Disclosure statement
The authors alone are responsible for the content and writing of the paper.
References
- Ferlay J, Soerjomataram I, Dikshit R, Eser S, Mathers C, Rebelo M, et al. Cancer incidence and mortality worldwide: Sources, methods and major patterns in GLOBOCAN 2012. Int J Cancer 2015;136:E359–86.
- Babjuk M, Burger M, Zigeuner R, Shariat SF, van Rhijn BWG, Compérat E, et al. EAU guidelines on non–muscle-invasive urothelial carcinoma of the bladder: Update 2013. Eur Urol 2013;64:639–53.
- Heney NM, Ahmed S, Flanagan MJ, Frable W, Corder MP, Hafermann MD, et al. Superficial bladder cancer: Progression and recurrence. J Urol 1983;130:1083–6.
- Sylvester RJ, van der Meijden APM, Lamm DL. Intravesical bacillus Calmette–Guérin reduces the risk of progression in patients with superficial bladder cancer: A meta-analysis of the published results of randomized clinical trials. J Urol 2002;168:1964–70.
- Ehdaie B, Sylvester R, Herr HW. Maintenance bacillus Calmette–Guérin treatment of non–muscle-invasive bladder cancer: A critical evaluation of the evidence. Eur Urol 2013;64:579–85.
- Lamm DL, van der Meijden APM, Morales A, Brosman SA, Catalona WJ, Herr HW, et al. Incidence and treatment of complications of bacillus Calmette–Guérin intravesical therapy in superficial bladder cancer. J Urol 1992;147:596–600.
- van der Heijden AG, Jansen CFJ, Verhaegh G, O’Donnell MA, Schalken JA, Witjes JA. The effect of hyperthermia on mitomycin-C induced cytotoxicity in four human bladder cancer cell lines. Eur Urol 2004;46:670–4.
- Lammers RJM, Witjes JA, Inman BA, Leibovitch I, Laufer M, Nativ O, et al. The role of a combined regimen with intravesical chemotherapy and hyperthermia in the management of non-muscle-invasive bladder cancer: A systematic review. Eur Urol 2011;60:81–93.
- Colombo R, Salonia A, Leib Z, Pavone-Macaluso M, Engelstein D. Long-term outcomes of a randomized controlled trial comparing thermochemotherapy with mitomycin-C alone as adjuvant treatment for non-muscle-invasive bladder cancer (NMIBC). BJU Int 2011;107:912–18.
- Sousa A, Inman BA, Piñeiro I, Monserrat V, Pérez A, Aparici V, et al. A clinical trial of neoadjuvant hyperthermic intravesical chemotherapy (HIVEC) for treating intermediate and high-risk non-muscle-invasive bladder cancer. Int J Hyperthermia 2014;30:166–70.
- Inman BA, Stauffer PR, Craciunescu OA, Maccarini PF, Dewhirst MW, Vujaskovic Z. A pilot clinical trial of intravesical mitomycin-C and external deep pelvic hyperthermia for non-muscle-invasive bladder cancer. Int J Hyperthermia 2014;30:171–5.
- Geijsen ED, de Reijke TM, Koning CC, Vörde Sive Vörding PJ Zum, de la Rosette JJ, Rasch CR, et al. Combining mitomycin C and regional 70 MHz hyperthermia in patients with nonmuscle invasive bladder cancer: A pilot study. J Urol 2015;194:1202–8.
- Stein JP, Lieskovsky G, Cote R, Groshen S, Feng AC, Boyd S, et al. Radical cystectomy in the treatment of invasive bladder cancer: Long-term results in 1,054 patients. J Clin Oncol 2001;19:666–75.
- Shariat SF, Karakiewicz PI, Palapattu GS, Lotan Y, Rogers CG, Amiel GE, et al. Outcomes of radical cystectomy for transitional cell carcinoma of the bladder: A contemporary series from the Bladder Cancer Research Consortium. J Urol 2006;176:2414–22; discussion 2422.
- Ghoneim MA, Abdel-Latif M, el-Mekresh M, Abol-Enein H, Mosbah A, Ashamallah A, et al. Radical cystectomy for carcinoma of the bladder: 2,720 consecutive cases 5 years later. J Urol 2008;180:121–7.
- Visser O, Nieuwenhuijzen JA, Horenblas S, Members of the Urological Oncology Working Group of the Comprehensive Cancer Centre Amsterdam. Local recurrence after cystectomy and survival of patients with bladder cancer: a population based study in greater Amsterdam. J Urol 2005;174:97–102.
- Munro NP, Sundaram SK, Weston PMT, Fairley L, Harrison SCW, Forman D, et al. A 10-year retrospective review of a nonrandomized cohort of 458 patients undergoing radical radiotherapy or cystectomy in Yorkshire, UK. Int J Radiat Oncol Biol Phys 2010;77:119–24.
- Arcangeli G, Strigari L, Arcangeli S. Radical cystectomy versus organ-sparing trimodality treatment in muscle-invasive bladder cancer: a systematic review of clinical trials. Crit Rev Oncol Hematol 2015;95:387–96.
- Ploussard G, Daneshmand S, Efstathiou JA, Herr HW, James ND, Rödel CM, et al. Critical analysis of bladder sparing with trimodal therapy in muscle-invasive bladder cancer: A systematic review. Eur Urol 2014;66:120–37.
- James ND, Hussain SA, Hall E, Jenkins P, Tremlett J, Rawlings C, et al. Radiotherapy with or without chemotherapy in muscle-invasive bladder cancer. N Engl J Med 2012;366:1477–88.
- van der Zee J, González González D, van Rhoon GC, van Dijk JD, van Putten WL, Hart AA, et al. Comparison of radiotherapy alone with radiotherapy plus hyperthermia in locally advanced pelvic tumours: a prospective, randomised, multicentre trial. Lancet 2000;355(9210):1119–25.
- Ott OJ, Rödel C, Weiss C, Wittlinger M, St Krause F, Dunst J, et al. Radiochemotherapy for Bladder Cancer. Clin Oncol 2009;21:557–65.
- Wittlinger M, Rödel CM, Weiss C, Krause SF, Kühn R, Fietkau R, et al. Quadrimodal treatment of high-risk T1 and T2 bladder cancer: Transurethral tumor resection followed by concurrent radiochemotherapy and regional deep hyperthermia. Radiother Oncol 2009;93:358–63.
- Griffin RJ, Dings RPM, Jamshidi-Parsian A, Song CW. Mild temperature hyperthermia and radiation therapy: Role of tumour vascular thermotolerance and relevant physiological factors. Int J Hyperthermia 2010;26:256–63.
- Song CW, Shakil A, Osborn JL, Iwata K. Tumour oxygenation is increased by hyperthermia at mild temperatures. Int J Hyperthermia 2009;25:91–5.
- Song CW, Park HJ, Lee CK, Griffin R. Implications of increased tumor blood flow and oxygenation caused by mild temperature hyperthermia in tumor treatment. Int J Hyperthermia 2005;21:761–7.
- Oei AL, van Leeuwen CM, ten Cate R, Rodermond HM, Buist MR, Stalpers LJA, et al. Hyperthermia selectively targets human papillomavirus in cervical tumors via p53-dependent apoptosis. Cancer Res 2015;75:5120–9.
- Oei AL, Vriend LEM, Crezee J, Franken NAP, Krawczyk PM. Effects of hyperthermia on DNA repair pathways: One treatment to inhibit them all. Radiat Oncol 2015;10:1–13.
- Bergs JWJ, Krawczyk PM, Borovski T, ten Cate R, Rodermond HM, Stap J, et al. Inhibition of homologous recombination by hyperthermia shunts early double strand break repair to non-homologous end-joining. DNA Repair 2013;12:38–45.
- Krawczyk PM, Eppink B, Essers J, Stap J, Rodermond H, Odijk H, et al. Mild hyperthermia inhibits homologous recombination, induces BRCA2 degradation, and sensitizes cancer cells to poly (ADP-ribose) polymerase-1 inhibition. Proc Natl Acad Sci USA 2011;108:9851–6.
- Toraya-Brown S, Fiering S. Local tumour hyperthermia as immunotherapy for metastatic cancer. Int J Hyperthermia 2014;30:531–9.
- Arends TJH, Falke J, Lammers RJM, Somford DM, Hendriks JCM, de Weijert MCA, et al. Urinary cytokines in patients treated with intravesical mitomycin-C with and without hyperthermia. World J Urol 2015 Oct;33:1411–17.
- Paroni R, Salonia A, Lev A, Da Pozzo LF, Cighetti G, Montorsi F, et al. Effect of local hyperthermia of the bladder on mitomycin C pharmacokinetics during intravesical chemotherapy for the treatment of superficial transitional cell carcinoma. Br J Clin Pharmacol 2001;52:273–8.
- Sapareto SA, Dewey WC. Thermal dose determination in cancer therapy. Int J Radiat Oncol Biol Phys 1984;10:787–800.
- Dewhirst MW, Abraham J, Viglianti B. Evolution of thermal dosimetry for application of hyperthermia to treat cancer. In: Sparrow EM, Abraham JP, Gorman JM, editor. Advances in heat transfer. Waltham, MA: Academic Press; 2015 p. 397–421.
- Dewhirst MW, Viglianti BL, Lora-Michiels M, Hanson M, Hoopes PJ. Basic principles of thermal dosimetry and thermal thresholds for tissue damage from hyperthermia. Int J Hyperthermia 2003;19:267–94.
- Lagendijk JJ, Van Rhoon GC, Hornsleth SN, Wust P, De Leeuw AC, Schneider CJ, et al. ESHO quality assurance guidelines for regional hyperthermia. Int J Hyperthermia 1998;14:125–33.
- Bruggmoser G, Bauchowitz S, Canters R, Crezee H, Ehmann M, Gellermann J, et al. Quality assurance for clinical studies in regional deep hyperthermia. Strahlenther Onkol 2011;187: 605–10.
- Yarmolenko PS, Moon EJ, Landon C, Manzoor A, Hochman DW, Viglianti BL, et al. Thresholds for thermal damage to normal tissues: An update. Int J Hyperthermia 2011;27:320–43.
- Nah BS, Choi IB, Oh WY, Osborn JL, Song CW. Vascular thermal adaptation in tumors and normal tissue in rats. Int J Radiat Oncol Biol Phys 1996;35:95–101.
- Lin JC, Song CW. Influence of vascular thermotolerance on the heat-induced changes in blood flow, pO2, and cell survival in tumors. Cancer Res 1993;53:2076–80.
- Van Rhoon GC. Is CEM43 still a relevant thermal dose parameter for hyperthermia treatment monitoring? Int J Hyperthermia 2016;32:50–62.
- Franckena M, Fatehi D, de Bruijne M, Canters RAM, van Norden Y, Mens JW, et al. Hyperthermia dose–effect relationship in 420 patients with cervical cancer treated with combined radiotherapy and hyperthermia. Eur J Cancer 2009;45:1969–78.
- Thrall DE, Maccarini P, Stauffer P, Macfall J, Hauck M, Snyder S, et al. Thermal dose fractionation affects tumour physiological response. Int J Hyperthermia 2012;28:431–40.
- Crezee J, van Leeuwen CM, Oei AL, Stalpers LJ, Bel A, Franken NA, et al. Thermoradiotherapy planning: Integration in routine clinical practice. Int J Hyperthermia 2016;32:41–9.
- Bruggmoser G. Some aspects of quality management in deep regional hyperthermia. Int J Hyperthermia 2012;28:562–9.
- Bruggmoser G, Bauchowitz S, Canters R, Crezee H, Ehmann M, Gellermann J, et al. Guideline for the clinical application, documentation and analysis of clinical studies for regional deep hyperthermia: Quality management in regional deep hyperthermia. Strahlenther Onkol 2012;188(Suppl2):198–211.
- Yuan Y, Cheng K-S, Craciunescu OI, Stauffer PR, Maccarini PF, Arunachalam K, et al. Utility of treatment planning for thermochemotherapy treatment of nonmuscle invasive bladder carcinoma. Med Phys 2012;39:1170–1181.
- Bondar ML, Hoogeman MS, Mens JW, Quint S, Ahmad R, Dhawtal G, et al. Individualized nonadaptive and online-adaptive intensity-modulated radiotherapy treatment strategies for cervical cancer patients based on pretreatment acquired variable bladder filling computed tomography scans. Int J Radiat Oncol Biol Phys 2012;83:1617–23.
- van de Schoot AJAJ, Schooneveldt G, Wognum S, Hoogeman MS, Chai X, Stalpers LJA, et al. Generic method for automatic bladder segmentation on cone beam CT using a patient-specific bladder shape model. Med Phys 2014;41:031707.
- Juang T, Stauffer PR, Craciunescu OA, Maccarini PF, Yuan Y, Das SK, et al. Thermal dosimetry characteristics of deep regional heating of non-muscle invasive bladder cancer. Int J Hyperthermia 2014;30:176–83.
- Cordeiro ER, Geijsen DE, Vörde Sive Vörding PJ Zum, Schooneveldt G, Sijbrands J, Hulshof MC, et al. Novel multisensor probe for monitoring bladder temperature during locoregional chemohyperthermia for nonmuscle-invasive bladder cancer: Technical feasibility study. J Endourol 2013;27:1504–9.
- Schooneveldt G, Kok HP, Geijsen ED, van Ommen F, Bakker A, Westendarf Zanartu MA, et al. Improved temperature monitoring and treatment planning for loco-regional hyperthermia treatments of non-muscle invasive bladder cancer (NMIBC). In: Jaffray DA, editor. World Congress on Medical Physics and Biomedical Engineering, June 7–12, 2015, Toronto, Canada. Cham: Springer International, 2015, pp. 1691–4.
- Stauffer PR, Van Rhoon GC. Overview of bladder heating technology. Matching capabilities with clinical requirements. Int J Hyperthermia. 2016;32.
- Sousa A, Piñeiro, I, Aparici V, Neira P, Monserrat V, Uribarri C. Análisis del impacto presupuestario del tratamiento mediante quimiohipertermia neoadyuvante en el cáncer vesical no músculo invasivo [Analysis of the budgetary impact of neo-adjuvant chemohyperthermia treatment of non-muscle invasive bladder cancer]. Urol Oncológica 2015;68:482–492.
- Sousa Escandon A. Conductive hyperthermia for bladder cancer. Int J Hyperthermia 2016;32.
- Rigatti P, Lev A, Colombo R. Combined intravesical chemotherapy with mitomycin C and local bladder microwave-induced hyperthermia as a preoperative therapy for superficial bladder tumors. A preliminary clinical study. Eur Urol 1991;20:204–10.
- van Valenberg H, Colombo R, Witjes JA. Intravesical radiofrequency induced hyperthermia for non-muscle-invasive bladder cancer. Int J Hyperthermia 2016;32.
- Colombo R, Salonia A, Da Pozzo LF, Naspro R, Freschi M, Paroni R, et al. Combination of intravesical chemotherapy and hyperthermia for the treatment of superficial bladder cancer: Preliminary clinical experience. Crit Rev Oncol Hematol 2003;47:127–39.
- Rath-Wolfson L, Moskovitz B, Dekel Y, Kugel V, Koren R. Combined intravesical hyperthermia and mitomycin chemotherapy: A preliminary in vivo study. Int J Exp Pathol 2003;84:145–52.
- Abe M, Hiraoka M, Takahashi M, Egawa S, Matsuda C, Onoyama Y, et al. Multi-institutional studies on hyperthermia using an 8-MHz radiofrequency capacitive heating device (Thermotron RF-8) in combination with radiation for cancer therapy. Cancer 1986;58:1589–95.
- Longo TA, Gopalakrishna A, Tsivian M, van Noord M, Inman BA, Geijsen ED. A systematic review of regional hyperthermia therapy in bladder cancer. Int J Hyperthermia 2016;32.
- Paulides MM, Stauffer PR, Neufeld E, Maccarini PF, Kyriakou A, Canters RAM, et al. Simulation techniques in hyperthermia treatment planning. Int J Hyperthermia 2013;29:346–57.
- Kok HP, Ciampa S, de Kroon-Oldenhof R, Steggerda-Carvalho EJ, van Stam G, Vörde Sive Vörding PJ Zum, et al. Toward online adaptive hyperthermia treatment planning: Correlation between measured and simulated specific absorption rate changes caused by phase steering in patients. Int J Radiat Oncol Biol Phys 2014;90:438–45.
- Canters RAM, Paulides MM, Franckena MF, van der Zee J, van Rhoon GC. Implementation of treatment planning in the routine clinical procedure of regional hyperthermia treatment of cervical cancer: an overview and the Rotterdam experience. Int J Hyperthermia 2012;28:570–81.
- Kok H, Wust P, Stauffer P, Bardati F, van Rhoon G, Crezee J. Current state of the art of regional hyperthermia treatment planning: a review. Radiat Oncol 2015;10:196.
- Fatehi D, van der Zee J, Notenboom A, van Rhoon GC. Comparison of intratumor and intraluminal temperatures during locoregional deep hyperthermia of pelvic tumors. Strahlenther Onkol 2007;183:479–86.
- Gellermann J, Włodarczyk W, Hildebrandt B, Ganter H, Nicolau A, Rau B, et al. Noninvasive magnetic resonance thermography of recurrent rectal carcinoma in a 1.5 Tesla hybrid system. Cancer Res 2005;65:5872–80.
- Gellermann J, Hildebrandt B, Issels R, Ganter H, Włodarczyk W, Budach V, et al. Noninvasive magnetic resonance thermography of soft tissue sarcomas during regional hyperthermia: Correlation with response and direct thermometry. Cancer 2006;107:1373–82.
- Oliveira TR, Stauffer PR, Lee C-T, Landon CD, Etienne W, Ashcraft KA, et al. Magnetic fluid hyperthermia for bladder cancer: A preclinical dosimetry study. Int J Hyperthermia 2013;29:835–44.
- Landon C, Lee C-T, Ashcraft KA, Oliveira TR, Boss M, Stauffer PR, et al. Toxicity concerns regarding the use of coated iron oxide magnetic particles for intravesicular bladder heating. Int J Hyperthermia in press.
- Cox RS, Kapp DS. Correlation of thermal parameters with outcome in combined radiation therapy–hyperthermia trials. Int J Hyperthermia 1992;8:719–32.
- Hand JW, Machin D, Vernon CC, Whaley JB. Analysis of thermal parameters obtained during phase III trials of hyperthermia as an adjunct to radiotherapy in the treatment of breast carcinoma. Int J Hyperthermia 1997;13:343–64.
- Kapp DS, Cox RS. Thermal treatment parameters are most predictive of outcome in patients with single tumor nodules per treatment field in recurrent adenocarcinoma of the breast. Int J Radiat Oncol Biol Phys 1995;33:887–99.
- Leopold KA, Dewhirst MW, Samulski TV, Dodge RK, George SL, Blivin JL, et al. Cumulative minutes with T90 greater than Tempindex is predictive of response of superficial malignancies to hyperthermia and radiation. Int J Radiat Oncol Biol Phys 1993;25:841–7.
- Oleson JR, Samulski TV, Leopold KA, Clegg ST, Dewhirst MW, Dodge RK, et al. Sensitivity of hyperthermia trial outcomes to temperature and time: Implications for thermal goals of treatment. Int J Radiat Oncol Biol Phys 1993;25:289–97.
- Sherar M, Liu FF, Pintilie M, Levin W, Hunt J, Hill R, et al. Relationship between thermal dose and outcome in thermoradiotherapy treatments for superficial recurrences of breast cancer: Data from a phase III trial. Int J Radiat Oncol Biol Phys 1997;39:371–80.
- Wust P, Stahl H, Dieckmann K, Scheller S, Löffel J, Riess H, et al. Local hyperthermia of N2/N3 cervical lymph node metastases: Correlation of technical/thermal parameters and response. Int J Radiat Oncol Biol Phys 1996;34:635–46.
- van der Zee J, van Rhoon GC, Wike-Hooley JL, Reinhold HS. Clinically derived dose effect relationship for hyperthermia given in combination with low dose radiotherapy. Br J Radiol 1985;58(687):243–50.
- Overgaard J, González González D, Hulshof MCCM, Arcangeli G, Dahl O, Mella O, et al. Hyperthermia as an adjuvant to radiation therapy of recurrent or metastatic malignant melanoma. a multicentre randomized trial by the European Society for Hyperthermic Oncology. 1996. Int J Hyperthermia 2009;25:323–34.
- Dinges S, Harder C, Wurm R, Buchali A, Blohmer J, Gellermann J, et al. Combined treatment of inoperable carcinomas of the uterine cervix with radiotherapy and regional hyperthermia. Results of a phase II trial. Strahlenther Onkol 1998;174:517–21.
- Sreenivasa G, Hildebrandt B, Kümmel S, Jungnickel K, Cho CH, Tilly W, et al. Radiochemotherapy combined with regional pelvic hyperthermia induces high response and resectability rates in patients with nonresectable cervical cancer > or = FIGO IIB ‘bulky’. Int J Radiat Oncol Biol Phys 2006;66:1159–67.
- Rau B, Wust P, Hohenberger P, Löffel J, Hünerbein M, Below C, et al. Preoperative hyperthermia combined with radiochemotherapy in locally advanced rectal cancer: A phase II clinical trial. Ann Surg 1998;227:380–9.
- Rau B, Wust P, Tilly W, Gellermann J, Harder C, Riess H, et al. Preoperative radiochemotherapy in locally advanced or recurrent rectal cancer: Regional radiofrequency hyperthermia correlates with clinical parameters. Int J Radiat Oncol Biol Phys 2000;48:381–91.
- Craciunescu OI, Stauffer PR, Soher BJ, Wyatt CR, Arabe O, Maccarini P, et al. Accuracy of real time noninvasive temperature measurements using magnetic resonance thermal imaging in patients treated for high grade extremity soft tissue sarcomas. Med Phys 2009;36:4848–58.
- Gellermann J, Włodarczyk W, Feussner A, Fähling H, Nadobny J, Hildebrandt B, et al. Methods and potentials of magnetic resonance imaging for monitoring radiofrequency hyperthermia in a hybrid system. Int J Hyperthermia 2005;21:497–513.
- Saccomandi P, Schena E, Silvestri S. Techniques for temperature monitoring during laser-induced thermotherapy: An overview. Int J Hyperthermia 2013;29:609–19.
- Colombo R. Multicentric study comparing intravesical chemotherapy alone and with local microwave hyperthermia for prophylaxis of recurrence of superficial transitional cell carcinoma. J Clin Oncol 2003;21:4270–6.
- Colombo R, Moschini M. Ruolo della chemio-ipertermia endocavitaria a microonde nel trattamento delle neoplasie vescicali non muscolo invasive. Una revisione sistematica della letteratura [Role of the combined regimen with local chemotherapy and microwave-induced hyperthermia for non-muscle invasive bladder cancer management. A systematic review]. Urologia 2013;80:112–19.
- Gofrit O., Shapiro A, Pode D, Sidi A, Nativ O, Leib Z, et al. Combined local bladder hyperthermia and intravesical chemotherapy for the treatment of high-grade superficial bladder cancer. Urology 2004;63:466–71.
- van der Heijden A, Kiemeney L, Gofrit O, Nativ O, Sidi A, Leib Z, et al. Preliminary European results of local microwave hyperthermia and chemotherapy treatment in intermediate or high risk superficial transitional cell carcinoma of the bladder. Eur Urol 2004;46:65–72.
- Schena E, Majocchi L. Assessment of temperature measurement error and its correction during Nd:YAG laser ablation in porcine pancreas. Int J Hyperthermia 2014;30:328–34.
- de Leeuw AA, Crezee J, Lagendijk JJ. Temperature and SAR measurements in deep-body hyperthermia with thermocouple thermometry. Int J Hyperthermia 1993;9:685–97.
- Dickinson RJ. Thermal conduction errors of manganin-constantan thermocouple arrays. Phys Med Biol 1985;30:445–53.
- Steinhart JS, Hart SR. Calibration curves for thermistors. Deep Sea Res Oceanogr Abstr 1968;15:497–503.
- Salahi S, Maccarini PF, Rodrigues DB, Etienne W, Landon CD, Inman BA, et al. Miniature microwave applicator for murine bladder hyperthermia studies. Int J Hyperthermia 2012;28:456–65.
- Stauffer P, Craciunescu O, Maccarini P, Wyatt C, Arunachalam K, Arabe O, et al. Clinical utility of magnetic resonance thermal imaging (MRTI) for realtime guidance of deep hyperthermia. Proc SPIE Int Soc Opt Eng 2009;7181.
- van Rhoon GC, Wust P. Introduction: Non-invasive thermometry for thermotherapy. Int J Hyperthermia 2005;21:489–95.
- Rieke V, Butts Pauly K. MR thermometry. J Magn Reson Imaging 2008;27:376–90.
- Quesson B, de Zwart JA, Moonen CT. Magnetic resonance temperature imaging for guidance of thermotherapy. J Magn Reson Imaging 2000;12:525–33.
- Lüdemann L, Włodarczyk W, Nadobny J, Weihrauch M, Gellermann J, Wust P. Non-invasive magnetic resonance thermography during regional hyperthermia. Int J Hyperthermia 2010;26:273–82.
- Kim Y. Advances in MR image-guided high-intensity focused ultrasound therapy. Int J Hyperthermia 2015;31:225–32.
- Gellermann J, Faehling H, Mielec M, Cho CH, Budach V, Wust P. Image artifacts during MRT hybrid hyperthermia – causes and elimination. Int J Hyperthermia 2008;24:327–35.
- Schmitt A, Mougenot C, Chopra R. Spatiotemporal filtering of MR-temperature artifacts arising from bowel motion during transurethral MR-HIFU. Med Phys 2014;41:113302.
- Zachiu C, Denis de Senneville B, Moonen C, Ries M. A framework for the correction of slow physiological drifts during MR-guided HIFU therapies: Proof of concept. Med Phys 2015;42:4137–48.
- Gellermann J, Weihrauch M, Cho CH, Włodarczyk W, Fähling H, Felix R, et al. Comparison of MR-thermography and planning calculations in phantoms. Med Phys 2006;33:3912–20.
- Hofstetter LW, Yeo DTB, Dixon WT, Kempf JG, Davis CE, Foo TK. Fat-referenced MR thermometry in the breast and prostate using IDEAL. J Magn Reson Imaging 2012;36:722–32.
- Kirchhoff G. Ueberdas Verhältniss zwischen dem Emissionsvermögen und dem Absorptionsvermögen der Körper für Wärme und Licht [On the relation between the radiating and absorbing powers of different bodies for light and heat]. Ann Phys Chem 1860;185:275–301.
- Hand JW. Physical techniques for delivering microwave energy to tissues. Br J Cancer Suppl 1982;5:9–15.
- Stauffer PR, Snow BW, Rodrigues DB, Salahi S, Oliveira TR, Reudink D, et al. Non-invasive measurement of brain temperature with microwave radiometry: Demonstration in a head phantom and clinical case. Neuroradiol J 2014;27:3–12.
- Klemetsen O, Birkelund Y, Jacobsen SK, Maccarini PF, Stauffer PR. Design of medical radiometer front-end for improved performance. Prog Electromagn Res B 2011;27:289–306.
- Stauffer PR, Rodriques DB, Salahi S, Topsakal E, Oliveira TR, Prakash A, et al. Stable microwave radiometry system for long term monitoring of deep tissue temperature. Proc SPIE Int Soc Opt Eng 2013;85840R.
- Snow BW, Arunachalam K, De Luca V, Maccarini PF, Klemetsen O, Birkelund Y, et al. Non-invasive vesicoureteral reflux detection: Heating risk studies for a new device. J Pediatr Urol 2011;7:624–30.
- Birkelund Y, Klemetsen O, Jacobsen SK, Arunachalam K, Maccarini PF, Stauffer PR. Vesicoureteral reflux in children: A phantom study of microwave heating and radiometric thermometry of pediatric bladder. IEEE Trans Biomed Eng 2011;58: 3269–78.
- Arunachalam K, Maccarini PF, De Luca V, Bardati F, Snow BW, Stauffer PR. Modeling the detectability of vesicoureteral reflux using microwave radiometry. Phys Med Biol 2010;55:5417–35.
- Jacobsen S, Stauffer PR. Can we settle with single-band radiometric temperature monitoring during hyperthermia treatment of chestwall recurrence of breast cancer using a dual-mode transceiving applicator? Phys Med Biol 2007;52:911–28.
- Stauffer PR, Maccarini PF, Arunachalam K, De Luca V, Salahi S, Boico A, et al. Microwave radiometry for non-invasive detection of vesicoureteral reflux (VUR) following bladder warming. Proc SPIE Int Soc Opt Eng 2011;79010V
- Hand JW, van Leeuwen GM, Mizushina S, van de Kamer JB, Maruyama K, Sugiura T, et al. Monitoring of deep brain temperature in infants using multi-frequency microwave radiometry and thermal modelling. Phys Med Biol 2001;46:1885–903.
- Jacobsen S, Stauffer P. Non-invasive temperature profile estimation in a lossy medium based on multi-band radiometric signals sensed by a microwave dual-purpose body-contacting antenna. Int J Hyperthermia 2002;18:86–103.
- Arunachalam K, Maccarini P, De Luca V, Tognolatti P, Bardati F, Snow B, et al. Detection of vesicoureteral reflux using microwave radiometry-system characterization with tissue phantoms. IEEE Trans Biomed Eng 2011;58:1629–36.
- Rodrigues DB, Maccarini PF, Salahi S, Oliveira TR, Pereira PJS, Limao-Vieira P, et al. Design and optimization of an ultra wideband and compact microwave antenna for radiometric monitoring of brain temperature. IEEE Trans Biomed Eng 2014;61:2154–60.
- Lewis MA, Staruch RM, Chopra R. Thermometry and ablation monitoring with ultrasound. Int J Hyperthermia 2015;31:163–81.
- Lai C-Y, Kruse DE, Caskey CF, Stephens DN, Sutcliffe PL, Ferrara KW. Noninvasive thermometry assisted by a dual-function ultrasound transducer for mild hyperthermia. IEEE Trans Ultrason Ferroelectr Freq Control 2010;57:2671–84.
- Foiret J, Ferrara KW. Spatial and temporal control of hyperthermia using real time ultrasonic thermal strain imaging with motion compensation, phantom study. PLoS One 2015;10:e0134938.
- Bayat M, Ballard JR, Ebbini ES. In vivo ultrasound thermography in presence of temperature heterogeneity and natural motions. IEEE Trans Biomed Eng 2015;62:450–7.
- Seip R, Ebbini ES. Noninvasive estimation of tissue temperature response to heating fields using diagnostic ultrasound. IEEE Trans Biomed Eng 1995;42:828–39.
- Maass-Moreno R, Damianou CA. Noninvasive temperature estimation in tissue via ultrasound echo-shifts. Part I. Analytical model. J Acoust Soc Am 1996;100:2514–21.
- Arnal B, Pernot M, Tanter M. Monitoring of thermal therapy based on shear modulus changes: I. shear wave thermometry. IEEE Trans Ultrason Ferroelectr Freq Control 2011;58:369–78.
- Brosses ES, Pernot M, Tanter M. The link between tissue elasticity and thermal dose in vivo. Phys Med Biol 2011;56:7755–65.
- Schooneveldt G, Kok HP, Balidemaj E, Geijsen ED, van Ommen F, Bakker A, et al. Thermal dosimetry for loco-regional radiofrequency hyperthermia treatment of the bladder: Accurate fluid modelling. 2016;[In preparation].
- Gellermann J, Wust P, Stalling D, Seebass M, Nadobny J, Beck R, et al. Clinical evaluation and verification of the hyperthermia treatment planning system hyperplan. Int J Radiat Oncol Biol Phys 2000;47:1145–56.
- Sreenivasa G, Gellermann J, Rau B, Nadobny J, Schlag P, Deuflhard P, et al. Clinical use of the hyperthermia treatment planning system HyperPlan to predict effectiveness and toxicity. Int J Radiat Oncol Biol Phys 2003;55:407–19.
- van Haaren PMA, Kok HP, van den Berg CAT, Vörde Sive Vörding PJ Zum, Oldenborg S, Stalpers LJA, et al. On verification of hyperthermia treatment planning for cervical carcinoma patients. Int J Hyperthermia 2007;23:303–14.
- Franckena M, Canters R, Termorshuizen F, van der Zee J, van Rhoon G. Clinical implementation of hyperthermia treatment planning guided steering: A cross over trial to assess its current contribution to treatment quality. Int J Hyperthermia 2010;26:145–57.
- Rijnen Z, Bakker JF, Canters RAM, Togni P, Verduijn GM, Levendag PC, et al. Clinical integration of software tool VEDO for adaptive and quantitative application of phased array hyperthermia in the head and neck. Int J Hyperthermia 2013;29:181–93.
- Pennes HH. Analysis of tissue and arterial blood temperatures in the resting human forearm. J Appl Physiol 1948;1:93–122.
- Turner PF. Regional hyperthermia with an annular phased array. IEEE Trans Biomed Eng 1984;31:106–14.
- Wust P, Gellermann J, Beier J, Wegner S, Tilly W, Tröger J, et al. Evaluation of segmentation algorithms for generation of patient models in radiofrequency hyperthermia. Phys Med Biol 1998;43:3295–307.
- Wust P, Seebass M, Nadobny J, Deuflhard P, Mönich G, Felix R. Simulation studies promote technological development of radiofrequency phased array hyperthermia. Int J Hyperthermia 1996;12:477–94.
- Kok HP, Van Haaren PMA, van de Kamer JB, Wiersma J, Van Dijk JDP, Crezee J. High-resolution temperature-based optimization for hyperthermia treatment planning. Phys Med Biol 2005;50:3127–41.
- Wiersma J, van Maarseveen RAM, van Dijk JDP. A flexible optimization tool for hyperthermia treatments with RF phased array systems. Int J Hyperthermia 2002;18:73–85.
- de Greef M, Kok HP, Correia D, Borsboom P-P, Bel A, Crezee J. Uncertainty in hyperthermia treatment planning: The need for robust system design. Phys Med Biol 2011;56:3233–50.
- Kotte AN, van Leeuwen GM, Lagendijk JJ. Modelling the thermal impact of a discrete vessel tree. Phys Med Biol 1999;44:57–74.
- Kok HP, van den Berg CAT, Bel A, Crezee J. Fast thermal simulations and temperature optimization for hyperthermia treatment planning, including realistic 3D vessel networks. Med Phys 2013;40:103303.
- Gabriel S, Lau RW, Gabriel C. The dielectric properties of biological tissues: II. Measurements in the frequency range 10 Hz to 20 GHz. Phys Med Biol 1996;41:2251–69.
- Gabriel C, Gabriel S. Compilation of the dielectrical properties of body tissues at RF and microwave frequencies. Report no.: AL/OE-TR-1996-0037. Brooks Air Force Base Texas: Armstrong Laboratory, 1996.
- Peyman A, Gabriel C. Dielectric properties of porcine glands, gonads and body fluids. Phys Med Biol 2012;57:N339–44.
- Katscher U, Voigt T, Findeklee C, Vernickel P, Nehrke K, Dossel O. Determination of electric conductivity and local SAR via B1 mapping. IEEE Trans Med Imaging 2009;28:1365–74.
- Liu J, Zhang X, Schmitter S, Van de Moortele P-F, He B. Gradient-based electrical properties tomography (gEPT): A robust method for mapping electrical properties of biological tissues in vivo using magnetic resonance imaging. Magn Reson Med 2015;74:634–46.
- van Lier ALHMW, Raaijmakers A, Voigt T, Lagendijk JJW, Luijten PR, Katscher U, et al. Electrical properties tomography in the human brain at 1.5, 3, and 7T: A comparison study. Magn Reson Med 2014;71:354–63.
- Balidemaj E, van Lier ALHMW, Crezee H, Nederveen AJ, Stalpers LJA, van den Berg CAT. Feasibility of electric property tomography of pelvic tumors at 3 T. Magn Reson Med 2015;73:1505–13.
- Balidemaj E, de Boer P, van Lier ALHMW, Remis RF, Stalpers LJA, Westerveld GH, et al. In vivo electric conductivity of cervical cancer patients based on B1+ maps at 3 T MRI. Phys Med Biol 2016;61:1596–1607 accepted.
- Balidemaj E, Kok HP, Schooneveldt G, van Lier ALHMW, Remis RF, Stalpers LJA, et al. Hyperthermia treatment planning for cervical cancer patients based on electric conductivity tissue properties acquired in vivo with EPT at 3 T MRI. Int J Hyperthermia 2016; accepted.
- Fu F, Xin SX, Chen W. Temperature- and frequency-dependent dielectric properties of biological tissues within the temperature and frequency ranges typically used for magnetic resonance imaging-guided focused ultrasound surgery. Int J Hyperthermia 2014;30:56–65.
- Walker DC, Smallwood RH, Keshtar A, Wilkinson BA, Hamdy FC, Lee JA. Modelling the electrical properties of bladder tissue – quantifying impedance changes due to inflammation and oedema. Physiol Meas 2005;26:251–68.
- Lagendijk JJ, Hofman P, Schipper J. Perfusion analyses in advanced breast carcinoma during hyperthermia. Int J Hyperthermia 1988;4:479–95.
- Inman BA, Etienne W, Rubin R, Owusu RA, Oliveira TR, Rodriques DB, et al. The impact of temperature and urinary constituents on urine viscosity and its relevance to bladder hyperthermia treatment. Int J Hyperthermia 2013;29:206–10.
- Fatehi D, van der Zee J, Wielheesen DHM, van Wieringen WN, van Rhoon GC. Intra-luminal thermometry: Is tissue type assignment a necessity for thermal analysis? Int J Hyperthermia 2006;22:463–73.
- Kiss B, Schneider S, Thalmann GN, Roth B. Is thermochemotherapy with the Synergo system a viable treatment option in patients with recurrent non-muscle-invasive bladder cancer? Thermochemotherapy for superficial BC. Int J Urol 2015;22:158–62.
- Colombo R. Combined treatment with local thermo-chemotherapy for non muscle invasive bladder cancer. The present role in the light of acquired data and preliminary cumulative clinical experiences. Arch Ital Urol Androl 2008;80:149–56.
- Dubois L, Sozanski J-P, Tessier V, Camart J-C, Fabre J-J, Pribetich J, et al. Temperature control and thermal dosimetry by microwave radiometry in hyperthermia. IEEE Trans Microw Theory Tech 1996;44:1755–61.
- Numan WCM, Hofstetter LW, Kotek G, Bakker JF, Fiveland EW, Houston GC, et al. Exploration of MR-guided head and neck hyperthermia by phantom testing of a modified prototype applicator for use with proton resonance frequency shift thermometry. Int J Hyperthermia 2014;30:184–91.
- Verhaart RF, Rijnen Z, Fortunati V, Verduijn GM, van Walsum T, Veenland JF, et al. Temperature simulations in hyperthermia treatment planning of the head and neck region: Rigorous optimization of tissue properties. Strahlenther Onkol 2014;190:1117–24.
- Verhaart RF, Verduijn GM, Fortunati V, Rijnen Z, van Walsum T, Veenland JF, et al. Accurate 3D temperature dosimetry during hyperthermia therapy by combining invasive measurements and patient-specific simulations. Int J Hyperthermia 2015;31:686–92.
- Tarasek MR, Pellicer R, Hofstetter LW, Numan WCM, Bakker JF, Kotek G, et al. Validation of MR thermometry: Method for temperature probe sensor registration accuracy in head and neck phantoms. Int J Hyperthermia 2014;30:142–9.