Abstract
Introduction. Although glial cell line-derived neurotrophic factor (GDNF) has a strong clinical potential, little is known of how the posttranslational modifications of GDNF affect its biological activity and therapeutic potential. In mammalian cells GDNF is synthesized as a preproprotein. During secretion GDNF dimerizes, folds with -S-S- bonds, is modified by N-linked glycosylation, and undergoes proteolytic processing. After production in E. coli, unglycosylated GDNF is renaturated in vitro. Nevertheless, GDNF from E. coli was used in Parkinson's disease-related clinical trials.
Material and methods. Constructs encoding variants of human GDNF were generated and expressed in mammalian cells. The proteins were analysed by SDS-PAGE, Western blotting, RET-phosphorylation assays, and N-terminal sequencing. The stability of mammalian GDNF was compared to commercial GDNF produced in E. coli.
Results. Posttranslational processing of mammalian GDNF depends on the expression conditions. Two forms of GDNF with different N-termini are formed. GDNF without a prosequence is secreted and biologically active. GDNF is modified by N-linked glycosylation at one (Asn49) out of two consensus sites. N-linked glycosylation aids proteolytic processing of GDNF. Both glycosylated and unglycosylated GDNF from mammalian cells are more stable than GDNF from E. coli.
Discussion. Posttranslational modifications of GDNF influence its stability, which may be critical for its clinical use.
Key messages
Biologically active glial cell line-derived neurotrophic factor (GDNF) can be secreted without a prosequence. Two forms of mature GDNF with diff erent N-terminal sequences are formed, and modifi cations close to the N-terminus may increase the stability of GDNF.
GDNF is modifi ed by N-linked glycosylation at only one (Asn49) out of two putative consensus sites, and in mammalian cells N-linked glycosylation aids proteolytic processing of GDNF.
GDNF expressed in mammalian cells is more stable than GDNF from E. coli, and the stability varies depending on the extracellular environment.
Introduction
The glial cell line-derived neurotrophic factor (GDNF) was originally found when searching for a neurotrophic factor which would prevent the continued degeneration of midbrain dopaminergic neurons—a hallmark of Parkinson's disease. Although GDNF was identified, cloned, and characterized based on its ability to promote the survival and dopamine uptake of dissociated rat embryonic midbrain cultures (Citation1), this protein soon turned out to be very broadly expressed and crucially important for the development of especially the enteric nervous system and kidney. GDNF is by now known as a multifunctional protein with the capacity to induce cellular survival, proliferation, migration, as well as differentiation (Citation2).
The therapeutic potential of GDNF awoke interest to search for homologous proteins. GDNF soon became the founding member of a small subfamily, GDNF family ligands (GFLs). This family consists of four highly homologous proteins: GDNF, neurturin (NRTN), artemin (ARTN), and persephin (PSPN) (Citation2). It was found that all GFLs signal through the same transmembrane receptor tyrosine kinase, REarranged during Transfection (RET), but that GFLs can activate RET only in the presence of a co- receptor: GDNF family receptor α (GFRα). Specificity arises through the preferential binding of each GFL to one of four GFRα1–4 co-receptors (Citation2). Upon activation, RET is transphosphorylated and triggers complex intracellular signalling cascades (Citation3,Citation4).
With time the use of GDNF (and to some extent also of other GFLs) has been considered for treating not only Parkinson's disease (Citation5), but also chronic pain (Citation6), amyotrophic lateral sclerosis (Citation7), Huntington's disease (Citation8), as well as the regeneration of the sciatic nerve (Citation9). The use of GDNF is also under consideration for treating addiction (Citation10) as well as depression (Citation11). Remarkably, GDNF and NRTN are the only growth factors that have reached phase II clinical trials for patients with Parkinson's disease (Citation12–17).
So far all GDNF-related clinical trials on patients with Parkinson's disease have been carried out with GDNF protein produced in E. coli (Citation12–16). This is problematic since GFLs expressed in E. coli are initially inactive, and the purification involves harsh biochemical methods (denaturation and renaturation) which affect the quality of the protein. Moreover, GDNF is a mammalian protein, and mammalian cells modify secreted proteins differently from bacteria. In mammalian cells GDNF is synthesized as a preproprotein which is proteolytically processed into a mature protein. The presequence guides the protein to the endoplasmic reticulum (ER) for secretion. During secretion GDNF is folded with -S-S- bonds, dimerizes, and furthermore becomes modified by N-linked glycosylation and proteolytic processing (Citation1). Strikingly little is known of how these posttranslational modifications affect the functionality of GDNF. Here we characterize GDNF produced in mammalian cells and furthermore show that it is a more stable protein than GDNF produced in E. coli.
Material and methods
Cell lines, transfection, and conditions for expression of GDNF in mammalian cells
HEK 293, CHO, MG87-RET cells were cultivated either in Dulbecco's modified Eagle's medium (DMEM) in the presence of 10% fetal bovine serum (FBS) and penicillin/streptomycin, or in Optimem (Opti-MEM , GIBCO , Carlsbad, CA, USA); 2 µg/mL puromycin was added to the media of MG87-RET cells. PC6 - 3 cells were cultivated in DMEM in the presence of 5% FBS, 10% horse serum, and penicillin/streptomycin. Transfection of CHO and MG87-RET cells was done by TurboFect (Fermentas (Waltham, MA, USA)), while HEK 293 cells were transfected with Lipofectamine 2000 (Invitrogen (Carlsbad, CA, USA)). N-linked glycosylation was inhibited by growing the cells in the presence of tunicamycin (5 µg/mL, Sigma-Aldrich Corporation (St. Louis, MO, USA)) for 2 days after transfection.
The up-scaled production of untagged GDNF was done using proprietary QMCF technology at Icosagen (Tartu, Estonia) (European Patent EP1851319). QMCF expression vectors carry a hybrid replication origin (enhancerless polyomavirus minimal origin of replication and maintenance element provided by Epstein-Barr virus family of repeats). The cells used by Icosagen Ltd are modified suspension CHO cells which express two additional proteins: the mouse polyomavirus large T antigen and Epstein-Barr virus EBNA1 protein. These two proteins provide replication initiation and maintenance function and effective expression of the protein of interest from the vectors in QMCF cells.
Plasmid constructs used to study the impact of mammalian posttranslational modifications on GDNF
The cDNA-encoding human pre(α)proGDNF (Citation18) was used as a template for inverse polymerase chain reaction mutagenesis. The eight differently tagged versions of GDNF are listed in . Point mutations and deletions were introduced as described in .
Table I. Sequence of diff erently tagged versions of human GDNF and primary structure of FLAG-tagged human prepro(α)GDNF.
Partial purification of mammalian GDNF for N-terminal sequencing
For partial purification of GDNF, the media from CHO suspension cells were concentrated using centrifugal filters (Amicon Ultra 10K, Millipore, Billerica, MA, USA) followed by gel filtration chromatography (Superdex 75, GE Healthcare, Little Chalfont, UK). The fractions were analysed by Western blotting, and those containing GDNF were pooled and diluted into 10 mM HEPES buffer (pH 8.0), for subsequent heparin affinity chromatography. HiTrap Heparin HP 1 mL columns (Amersham Biosciences (Uppsala, Sweden)) were equilibrated with 10 mL of HEPES (pH 8.0). The samples were loaded on the column, washed with 5 mL of 10 mM HEPES (pH 8.0), 100 mM NaCl, and eluted using a step gradient with up to 1.5 M NaCl in 10 mM HEPES (pH 8.0). Samples from each fraction were analysed by reducing sodium dodecyl sulfate-polyacrylamide gel electrophoresis (SDS-PAGE), and the gels were stained with Coomassie brilliant blue.
N-terminal sequencing of mammalian GDNF
For N-terminal sequencing, partially purified GDNF was run on a reducing 15% SDS-PAGE, electroblotted onto a polyvinylidene difluoride (PVDF) membrane, and the membrane stained with Coomassie brilliant blue (Citation19). The protein bands of interest were cut out and subjected to N-terminal sequence analysis using an Applied Biosystems Procise 494A HT sequencer (Perkin Elmer, Applied Biosystems Division, Foster City, CA, USA).
Antibodies used to detect GDNF and its receptor RET in Western blotting
Western blotting was done with rabbit antibodies to GDNF (D-20) and goat antibodies to RET (C-20). Both antibodies were from Santa Cruz Biotechnology Inc. (Santa Cruz, CA, USA) and they were diluted 1:500. Mouse antibodies to phosphotyrosine (4G10) were from Millipore/Upstate and they were diluted 1:1500. Rabbit antibodies to the prosequence of GDNF have been described in (Citation18).
Monitoring of the stability of GDNF variants by incubation in media of mammalian cell lines
For incubations without cells, media from CHO cells transfected with plasmids encoding either GDNF variants or green fluorescent protein (GFP) were collected 2 days after transfection. GDNF produced in E. coli (ProSpec (East Brunswick, NJ, USA)) was diluted to a final concentration of 1 µg/mL in medium from CHO cells expressing GFP. GDNF-containing media were incubated on cell culture plates for 0–8 hours in a cell culture incubator at 37°C before analysis by Western blotting.
For incubations on cells, media from transiently transfected CHO cells were collected as above. After a 1:5 dilution into Opti-MEM it was transferred onto untransfected CHO cells, MG87-RET cells, or PC6 - 3 cells. Samples were taken from the media after 1, 6, and 24 hours’ incubation and analysed by Western blotting.
Monitoring of the biological activity of GDNF variants with RET phosphorylation assays
MG87-RET cells were transiently transfected with plasmids encoding rat GFRα1 as previously described [20]. On the following day the cells were starved for 4 hours in serum-free DMEM at 37°C and then stimulated for 10 min at 37°C with media from CHO cells transiently transfected 2 days earlier with plasmids encoding the GDNF variants or GFP. The medium from cells expressing GFP was used in the presence or absence of commercial GDNF from E. coli (100 ng/mL, ProSpec). For phosphorylation assays with soluble GFRα1 (R&D Systems, Minneapolis, MN, USA), untransfected MG87-RET cells were starved as above, before induction with soluble GFRα1 (1 μg/mL) in the media of respective CHO cells. Medium from GFP transfected cells was used as controls as above. When using soluble GFRα1 the induction time was 1 h. All cell lysates were prepared as described in (Citation20), and for the phosphorylation assays antibodies to RET were added to the postnuclear supernatant. The immunocomplexes were collected with Protein G–Sepharose (Amersham Biosciences) and analysed by Western blotting with antibodies to phosphotyrosine. Equal loading was verified by re-staining the filters with antibodies to RET.
Results
Tagging of GDNF
In many cases tagging of a protein is beneficial, for instance for distinguishing exogenously added protein from endogenous protein, for precipitation of interacting proteins, or for development of sensitive detection methods. In human GDNF, one of the seven -S-S- bonds involves a cysteine residue which is located at the very C-terminus as the second last amino acid (Citation21). To avoid possible interference with the formation of this C-terminal -S-S- bond, we added a tag at the N-terminus of the mature protein, with or without a short linker (GG). Two types of tags were added, a FLAG-tag and a His-tag. The tags were introduced into constructs encoding human GDNF with or without the prosequence. The eight constructs () were transiently expressed in CHO cells. The media were collected and analysed by reducing SDS-PAGE and subsequent Western blotting with antibodies to GDNF. Addition of the His-tag impaired the secretion of preproGDNF, while it was less harmful for the secretion of the truncated construct lacking the prosequence (). Addition of a FLAG-tag did not impair the secretion of GDNF. The banding pattern of secreted GDNF is complex (), and as reported previously proGDNF (highest band present only after expression of constructs with the prosequence) is detectable in the media after overexpression in mammalian cells (Citation18). The nature of the two lower bands is discussed later ().
Figure 1. Characterization of the human prepro(α)GDNF variants. A: Characterization of differently tagged versions of GDNF. CHO cells were transiently transfected with eight constructs encoding different variants of human GDNF or empty vector. The constructs are numbered as in and shown on top of the panel. Two days after transfection, samples were taken from the media and analysed by Western blot using antibodies to GDNF. B: Comparison of cell lines and expression time. PreproFLAG-GG-GDNF (construct number 1, prepro) and preFLAG-GG-GDNF (construct number 5, pre) were chosen for further studies. The two constructs were expressed for 1 and 2 days after transfection (DAT) in HEK 293 and CHO cells. Media were collected and analysed as in (A). C: Comparison of expression media. CHO cells were transfected with preproGDNF (*, no tag), preproFLAG-GG-GDNF (construct number 1, prepro), or preFLAG-GG-GDNF (construct number 5, pre). These constructs were expressed for 2 days in CHO cells grown in DMEM with 10% FBS (DMEMFBS) or Opti-MEM (OM). The media as well as cell lysates (cells lysed in a volume equal to the collected media) were collected and analysed as in (A). D: RET-P activity assay with human prepro(α)GDNF variants. CHO cells were transfected with GFP or the GDNF variants. Constructs number 1–2 (prepro F), number 5–6 (pre F) and number 7–8 (pre H) are numbered according to . Two days after transfection the media were collected and used to stimulate the phosphorylation of RET in MG87-RET cells. Medium from GFP expressing CHO cells was used as a negative control (–). As a positive control, GDNF from E. coli (100 ng/mL) was added to the media from GFP-expressing CHO cells (GDNF/E. coli). All inductions were done in the presence of soluble GFRα1 (1 μg/mL) for 1 hour.
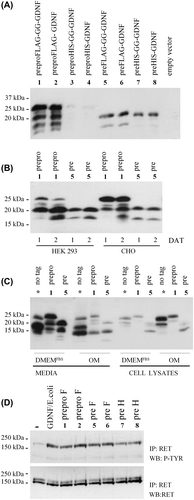
Figure 2. Characterization of N-terminal sequences. Coomassie-stained PVDF membrane of human GDNF electroblotted from reducing SDS-PAGE for N-terminal sequencing. Untagged preproGDNF (-FLAG) as well as preproFLAG-GG-GDNF (+ FLAG) were expressed in CHO cells grown in suspension. The proteins were partially purified, and fractions (Citation1–6) from the heparin column were run on a 15% reducing SDS-PAGE, blotted onto PVDF, and stained with Coomassie brilliant blue. The strong band (I) in lane 3 of the untagged sample, and the two strongest bands (II and III) in lane 3 of the FLAG-tagged sample were excised and the N-terminal sequences of the proteins were determined by Edman degradation.
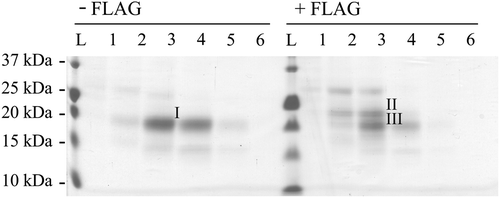
Effect of expression conditions on the posttranslational processing of GDNF
For further studies we used two of the FLAG-tagged constructs (number 1, preproFLAG-GG-GDNF, and number 5, preFLAG-GG-GDNF). These constructs were transiently transfected into CHO or HEK 293 cells. After 1 and 2 days, samples from the media were analysed as above. The concentration of the ligand increases with time, and the proteolytic processing of proGDNF might be slightly more efficient in HEK 293 cells than in CHO cells ().
Because different laboratories use different conditions to verify and characterize the expression of secreted proteins, we also tested the effect of different media on the expression and modification of GDNF. Many mammalian cells are normally grown in DMEM supplemented with 10% serum, but for characterization of secreted proteins the high serum concentrations can be problematic. Therefore the media is often changed into Opti-MEM which contains low concentrations of growth factors and thus minimizes the need of added serum. The two FLAG-tagged constructs were transfected into CHO cells, and after transfection the media were changed into either DMEM with 10% FBS, or Opti-MEM without FBS. Analysis of the media shows that both the amount and processing of GDNF indeed depend on the composition of the media (). Analyses of the cell lysates (the volume of lysis buffer equals the volume of the medium) show that the medium affects the secretion efficiency of GDNF. Although the choice of the media may seem to be a very trivial matter, the results obtained are highly dependent on this parameter. In conclusion, the results show that the tagging, choice of cells and media, as well as the time point for taking the samples affect both the yield and the processing pattern of secreted GDNF. These issues should therefore not be neglected when different GDNF constructs are prepared and characterized for clinical use.
Biologically active GDNF can be secreted without a prosequence
One of the classical functions of prosequences of secreted proteins is to aid folding. If the prosequence of GDNF were needed for folding, truncated GDNF without a prosequence would remain unfolded, and the quality control mechanisms of the secretory system in eukaryotic cells would degrade it. In –C we show that GDNF lacking the prosequence is secreted into the media, but maybe at a lower level than the wild-type protein. The result indicates that the prosequence may be involved in, but is not absolutely required for, secretion and folding of GDNF. The biological activity of GDNF expressed from plasmids with or without the prosequence was verified using RET-phosphorylation assays ().
Identification of a novel N-terminus of mature GDNF
To characterize the complex processing pattern of GDNF secreted from mammalian cells, CHO suspension cells were used for high expression of preproFLAG-GG-GDNF and preproGDNF. After partial purification, GDNF was subjected to reducing SDS-PAGE, electroblotted onto a PVDF membrane, and subsequently visualized by Coomassie staining. Protein bands from both tagged and untagged GDNF were excised from the membrane and subjected to N-terminal amino acid sequence analysis by Edman degradation ().
Analysis of the band (, band I) isolated from the sample of untagged GDNF (preproGDNF) surprisingly revealed the presence of two N-terminal sequences, one at the conventional furin cleavage site (..KRLKR▾SPDKQMAV..) described by (Citation1), the other located six amino acid residues after this site (..KRLKRSPDKQM▾AV..). Analysis of the upper band (, band II) of preproFLAG-GG-GDNF shows that in spite of the FLAG-tag, mature GDNF is cleaved at the conventional furin site (..KRLKR▾DYKDDDDKGGSPDKQMAV..), while analysis of the lower band (, band III) of preproFLAG-GG-GDNF corroborates the presence of a second cleavage site located close to the furin site (..KRLKRDYKDDDDKGGSPDKQM▾AV..).
In the untagged construct the two different sites are separated by only six amino acid residues (full sequence shown in ). In the FLAG-tagged construct the two different cleavage sites are separated by 16 residues due to the addition of 10 extra amino acid residues (FLAG-tag + linker). Therefore, the presence of two different cleavage products is easier to detect visually on reducing SDS-PAGE () with tagged GDNF than with untagged GDNF. Taken together, mammalian cells produce two different mature GDNF proteins, consisting of either 134 or 128 amino acid residues.
Finally we studied if the proteolytic cleavage at the second N-terminal site (..KRLKRSPDKQM▾AV..) could be inhibited and if this would lead to an increased stability of GDNF. For this purpose we mutated one to three amino acids located in front of this cleavage site (). No significant difference in the processing of the secreted GDNF variants was detected after expression in CHO cells, and the variants were only somewhat more resistant to proteolytic cleavage when incubated on fibroblasts (data not shown).
N-linked glycan is present at only one of the putative glycosylation sites of GDNF
While human NRTN, ARTN, and PSPN lack potential N-linked glycosylation sites, human GDNF harbours two potential sites at N49 and N85. GDNF has also been shown to be secreted from mammalian cells as an N-linked glycosylated protein (Citation1,Citation22). Here we made two constructs, where either the first (preproFLAG-GG-GDNFN49A) or the second (preproFLAG-GG-GDNFN85A) of the potential N-linked glycosylation sites of human GDNF were mutated (N to A) (). The proteins were analysed after transient expression and secretion from CHO cells. To specifically inhibit N-linked glycosylation, part of the cells were grown in the presence of tunicamycin, and the proteins were analysed by reducing SDS-PAGE and subsequent Western blot analysis. The results show that only the first (N49) of the two potential glycosylation sites is in use (). This glycosylation site is located close to the tip of one of the receptor-binding finger domains of GDNF ().
Figure 3. Characterization of N-linked glycosylation. A: Use of the potential N-linked glycosylation sites. CHO cells were transfected with GFP, preproFLAG-GG-GDNF (WT), preproFLAG-GG-GDNFN85A (N85A), or preproFLAG-GG-GDNFN49A (N49A). The cells were incubated in the absence or presence of tunicamycin (TM). After 2 days the media (upper panel) and cell lysates (lower panel) were collected and analysed as in Figure 1A. B: Characterization of unglycosylated GDNF from mammalian cells. PreproFLAG-GG-GDNF (WT), unglycosylated preproFLAG-GG-GDNFN49A (N49A), preFLAG-GG-GDNF (WT w/o pro), and preFLAG-GG-GDNFN49A (N49A w/o pro) were transiently expressed in CHO cells for 2 days and analysed by Western blot analysis with antibodies to proGDNF (Citation18) (upper panel). The filter was stripped and reprobed with antibodies to GDNF (lower panel). Untagged and unglycosylated GDNF from E. coli was included as a control.
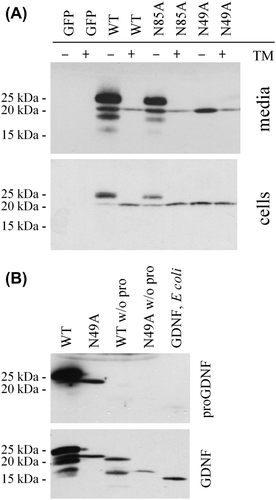
Glycosylation of GDNF affects its processing in mammalian cells, but not its receptor specificity
As can be seen in , the processing of the GDNF mutant with the first N-linked glycosylation site mutated (preproFLAG-GG-GDNFN49A) differs dramatically from the processing of the wild-type protein (preproFLAG-GG-GDNF). In addition the secretion of this variant of GDNF into the medium is impaired, although expression levels inside the cells remain rather equal (). These results indicate that in mammalian cells the glycosylation of GDNF is needed for the correct processing of the protein. This mutant variant was prepared by replacing N into A, and the changed amino acid sequence could indeed directly affect the folding of the protein. However, when wild-type GDNF with unchanged amino acid sequence was expressed in the presence of tunicamycin (inhibitor of N-linked glycosylation), the processing was similar to that of preproFLAG-GG-GDNFN49A (). Therefore we conclude that glycosylation affects the folding/processing of GDNF in mammalian cells.
To characterize the processing of preproFLAG-GG-GDNFN49A, we analysed the protein with an antibody specific to the GDNF prosequence (Citation18). Surprisingly the analysis shows that the only protein band that can be detected after expression of preproFLAG-GG-GDNFN49A represents unprocessed proGDNF (). Taken together, these results indicate that the N to A mutation at the glycosylation site hinders not only glycosylation but also cleavage of proGDNF into mature GDNF.
GFLs make contact with the GFRα receptors via two finger-like structures (Citation20,Citation23). The site where N-linked glycosylation occurs in GDNF is located close to the tip of one of these finger-like structures (). Therefore we examined whether glycosylation of GDNF possibly affects its capacity to activate the receptor complexes. The results show that unglycosylated GDNF lacking the prosequence (preFLAG-GG-GDNFN49A) can activate RET via GFRα1 and GFRα2, while it was unable to activate RET through GFRα3 or GFRα4 (data not shown). These results are in line with previous results with wild-type GDNF (Citation2,Citation24), and we therefore conclude that glycosylation of GDNF is not critical for its ability to activate its receptors.
Mammalian GDNF is more stable than GDNF produced in E. coli
For clinical trials large quantities of GDNF have been produced in E. coli (Citation12–16). The purification of GFLs from E. coli inclusion bodies easily involves harsh steps such as denaturation/renaturation and monomerization/dimerization (Citation1,Citation25). Mammalian GDNF is a dimeric protein with a complex folding that involves the formation of seven -S-S- bonds firmly organized into a complicated cysteine knot (Citation21). In addition GDNF is glycosylated when expressed in mammalian cells, while it remains unglycosylated when expressed in E. coli (Citation1).
To study if the production procedure has an impact on the stability of GDNF, we compared the stability of GDNF from E. coli to that of GDNF from CHO cells. CHO cells were transfected with either FLAG-tagged GDNF (preproFLAG-GG-GDNF), untagged GDNF (preproGDNF), or GFP. After 2 days cell-free media were collected. The medium from GFP-expressing cells was added to GDNF from E. coli, and this mix, as well as the media from GDNF-expressing CHO cells, was incubated for 8 hours at 37°C in the absence of any cells. The stability of GDNF was monitored by reducing SDS-PAGE and Western blot analysis. The results () show that both tagged and untagged mammalian GDNF are rather stable proteins. While proGDNF disappears more quickly, both FLAG-tagged and untagged mature GDNF from mammalian cells are clearly more stable than GDNF produced in E. coli (the bands are identified in and 3B).
Figure 4. Stability of GDNF from E. coli and mammalian cells. A: Stability of GDNF from E. coli compared to tagged and untagged GDNF from mammalian cells. CHO cells were transfected with untagged GDNF (preproGDNF-FLAG), tagged GDNF (preproGDNF + FLAG), or GFP. After 2 days expression the media were collected and GDNF from E. coli was added to the media from cells expressing GFP. Samples were taken from the media after 0, 2, and 8 hours’ incubation at 37°C, and analysed by Western blot using antibodies to GDNF. B: Stability of unglycosylated GDNF from mammalian cells. CHO cells were transfected with four different versions of FLAG-tagged GDNF: preproFLAG-GG-GDNF (preproGDNF), preFLAG-GG-GDNF (preGDNF) or preproFLAG-GG-GDNFN49A (preproGDNFN49A) and preFLAG-GG-GDNFN49A (preGDNFN49A). After 2 days of expression the media were collected and incubated for 0, 2, and 8 hours at 37°C. The samples were analysed as in (A). C: Stability of mammalian GDNF in different extracellular environment. CHO cells were transfected with preproFLAG-GG-GDNF (preproGDNF). After 2 days of expression (in DMEM with 10% FBS) the media were collected, diluted 1:5 into Opti-MEM , and transferred onto untransfected CHO cells, MG87-RET cells, or PC6 - 3 cells. Samples were taken from the media after 1, 6, and 24 hours’ incubation, and analysed as in (A).
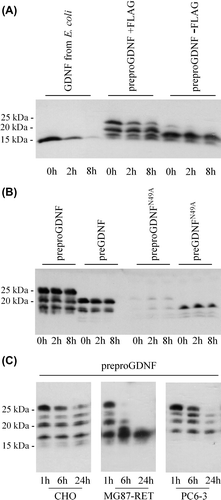
To characterize if the stability of mammalian GDNF depends on N-linked glycosylation or on the presence of a prosequence, we used a GDNF variant with a mutated glycosylation site, without the prosequence. The stability of this variant remained similar to the stability of wild-type GDNF (). We therefore conclude that mammalian GDNF is mostly stabilized by a proper folding and not by glycosylation. Finally, we incubated mammalian GDNF in the presence of different cell types, including CHO, fibroblasts (MG87-RET cells), and pheochromocytoma cells (PC6 - 3) cells for 24 hours. Not surprisingly these results show that variations in the extracellular environment also affect the processing/degradation of mammalian GDNF ().
Discussion
GDNF or proGDNF for in-vivo use?
Mature ligands can be administered as therapeutic proteins by direct injection or infusion. Alternatively, viruses or DNA nanoparticles encoding the ligand can be used to drive its expression in specific target cells of the mammalian host. Naked or encapsulated mammalian cells that secrete a ligand can also be implanted into the tissue. Constructs used in viruses, DNA nanoparticles, or encapsulated cells must encode mammalian secretion sequences and sometimes also require prosequences, which are cleaved off during secretion of the protein. These constructs can naturally be engineered to produce modified ligands. This is for instance relevant for NRTN, which is not very efficiently secreted from transfected cells. The secretion of NRTN from mammalian cells has been improved by two different approaches: Firstly, by deleting the prosequence (Citation26,Citation27), and, secondly, by exchanging the preprosequence of NRTN into the preprosequence of nerve growth factor (Citation28).
However, in the case of GDNF the presence of the prosequence may increase the amount of secreted GDNF (–C, ), possibly by improving the folding of the protein. In addition, two different splice variants of GDNF, with two different prosequences (α and β), have been described (Citation29) and shown to be differently secreted (Citation18,Citation30). The presence/absence and choice of a prosequence might therefore be important. On the other hand the prosequence might also induce unexpected side-effects. As shown here and by others (Citation18), unprocessed proGDNF can be secreted, at least upon overexpression. ProGDNF or the pro-region may have some function outside the cell, especially as an 11-residue peptide from the GDNF pro-region induces biological activity when added to cells (Citation31,Citation32). The secretion of proGDNF might thus lead to an induction of unexpected activity in cells located close to the expression site. Therefore it is important to know that biologically active GDNF can be secreted without a prosequence in mammalian cells, albeit maybe at sub-optimal concentrations (–C, ).
The processing of mammalian GDNF is variable
Here we show that GDNF can be N-terminally tagged but that the type of the tag has to be carefully chosen, not to interfere with the processing of the protein. We also show that a tag can affect the processing differently if introduced into a construct with or without the prosequence (). Finally we show that the presence of an N-terminal FLAG-tag apparently hampers the cleavage of the prosequence from the mature protein, which leads to an increased secretion of proGDNF (). Keeping in mind that even the choice of expression conditions affects the posttranslational processing of GDNF (), the quality and quantity of different GDNF variants need to be carefully characterized in each experimental set-up, both in vitro and in vivo.
Can GDNF be engineered to be more stable?
Here we identified a new N-terminal cleavage site of mature GDNF (). When mature human GDNF is cleaved at the conventional furin cleavage site, a protein of 134 amino acid residues is formed (Citation1). With the new cleavage site the mature protein becomes six amino acids shorter. Compared to the other GFLs, GDNF has an N-terminal extension (20–37 amino acids longer than other GFLs) with unknown function and structure (Citation20,Citation21). This extension is not important for the activation of the GFRα1/RET complex (Citation24,Citation33), and only the distal part of it mediates heparin binding (Citation34). Therefore, the here-described new cleavage site at the proximal end is likely not involved in regulating of heparin or GFRα1 binding. Our studies on GDNF variants with amino acid substitutions before the cleavage site show that modifications in this region might possibly affect its resistance to proteolytic cleavage outside the cells. Since little is known about the half-life of GDNF, and how and where GDNF is degraded, it would be interesting to study the here-described new cleavage site in more detail in the future.
In mammalian cells glycosylation is needed for the folding and processing of GDNF
Previously it was shown that GDNF is secreted as a glycosylated protein (Citation1). Here we show that GDNF is glycosylated only at the first of the two potential N-linked glycosylation sites, and that interference with glycosylation affects the processing of the protein. GDNF is a dimeric protein with a cysteine knot-like set of seven -S-S- bonds (Citation21,Citation35). The core moiety of N-linked glycosylation is added to proteins already in the ER, where also the folding and formation of -S-S- bonds takes place. Therefore it is not surprising that interfering with the glycosylation of GDNF affects the subsequent folding/processing of the protein ().
However, in light of the results described above, it is surprising that unglycosylated GDNF purified and renaturated from E. coli is biologically active and even folded with the typical cysteine knot structure (Citation21). The purification of proteins from E. coli often involves denaturation and monomerization/ dimerization steps (Citation1,Citation25), and GDNF can apparently be refolded in vitro into a biologically active protein after reduction. However, as GDNF retains biological activity even after chemical interference with its dimerizing intermolecular -S-S- bond (Citation36), it is possible that batches of GDNF produced in E. coli contain varying proportions of optimally and sub-optimally folded protein.
Comparing the use of GDNF from mammalian cells and E. coli
The GFL concentrations used for induction of biological activity through the GFRα/RET complex in vitro are generally 1–100 ng/mL (Citation1,Citation20,Citation24,Citation25). However, the concentrations used for in-vivo assays in rat models of the Parkinson's disease are considerably higher. Rats are commonly given 3–100 μg of GDNF or NRTN as intracranial injections (Citation37–39), and the dose of GDNF given to animals as chronic infusion amounts to about 3–10 μg of GDNF per day (Citation40,Citation41). If the ligands spread uniformly in the rat brain to a final concentration of 50 ng/mL, then 5 μg of the ligand would be sufficient to cover a brain volume of 100 mL. Human patients have so far received 3–43 μg of GDNF in the caudate putamen per day (Citation12–16). The volume of the human brain is about 1200 mL, while the volume of the whole rat brain is around 2.5 mL. In both cases it should be noted that the extracellular space of the targeted striatum is only a small part of that. Therefore it is clear that GDNF is used in excess, but unclear why so high doses are needed.
GDNF is a sticky protein that is difficult to handle. From a protein chemistry point of view, there is a risk that the injection of excessive amounts and high concentrations of GDNF leads to the aggregation and inactivation of GDNF in the tissue. When GDNF (2 μg/mL) was incubated in a preparation of extracellular matrix from mouse brain, it was stable for more than 48 hours independently of whether it was produced in insect cells or E. coli (Citation39). However, in the striatum of rats, the level of GDNF rapidly decreased between day 3 and 7 postinfusion after a single injection of 15 μg/10 μL (Citation42). It is possible that the stability of GDNF depends not only on the origin of the protein and the extracellular environment, but also on the concentrations used and the composition of the delivery solution.
The amounts of GDNF produced inside the tissue with the aid of viral vectors or encapsulated cells are very low compared to the amounts of injected or infused GDNF. After the injection of an adenoviral vector encoding GDNF, only 13 ng of GDNF was detected in the ventral mesencephalon. These low levels of GDNF mediated the protection of dopaminergic neurons in a 6-OHDA model of the Parkinson's disease (Citation43). From 25 pg to 100 ng of GDNF was estimated to be secreted per day from encapsulated cells, and these low levels of GDNF improved both the regeneration of the sciatic nerve (Citation44) as well as protected dopaminergic neurons in animal models of Parkinson's disease (Citation45–47). The successful use of very small amounts of GDNF produced in mammalian cells is supported by results showing that NRTN from mammalian cells is biologically more active than after production in E. coli (Citation26). Here we characterized the posttranslational modification of GDNF in mammalian cells and show that in our testing conditions mammalian GDNF is more stable than GDNF from E. coli. As clinical trials so far were conducted with GDNF from E. coli we hope that our results will support the use of mammalian GDNF in coming trials.
Acknowledgements
We thank Mari Heikkinen and Denise Heinrich for excellent technical assistance, Maria Lume and Mikko Airavaara for critical reading of the manuscript, and Icosagen Ltd (previously Quattromed Ltd) for expression of GDNF with high-expression technology in CHO cells. This work was supported by Academy of Finland (grant numbers 11411226 and 1126735), S. Jusélius foundation, an Erasmus Placement scholarship, IUBMB (Wood-Whelan fellowship), CIMO (Finnish Government Scholarship Pool), and a University of Helsinki Research Foundation grant.
Declaration of interest: The authors report no conflicts of interest.
References
- Lin LF, Doherty DH, Lile JD, Bektesh S, Collins F. GDNF: a glial cell line-derived neurotrophic factor for midbrain dopaminergic neurons. Science. 1993;260:1130–2.
- Airaksinen MS, Saarma M. The GDNF family: signalling, biological functions and therapeutic value. Nat Rev Neurosci. 2002;3:383–94.
- Kodama Y, Asai N, Kawai K, Jijiwa M, Murakumo Y, Ichihara M, . The RET proto-oncogene: a molecular therapeutic target in thyroid cancer. Cancer Sci. 2005;96:143–8.
- Runeberg-Roos P, Saarma M. Neurotrophic factor receptor RET: structure, cell biology, and inherited diseases. Ann Med. 2007;39:572–80.
- Vastag B. Biotechnology: Crossing the barrier. Nature. 2010;466: 916–8.
- Bartolini A, Di Cesare Mannelli L, Ghelardini C. Analgesic and antineuropathic drugs acting through central cholinergic mechanisms. Recent Pat CNS Drug Discov. 2011;6:119–40.
- Kanning KC, Kaplan A, Henderson CE. Motor neuron diversity in development and disease. Annu Rev Neurosci. 2010;33:409–40.
- Sari Y. Huntington's disease: from mutant Huntingtin protein to neurotrophic factor therapy. Int J Biomed Sci. 2011;7:89–100.
- Kokai LE, Bourbeau D, Weber D, McAtee J, Marra KG. Sustained growth factor delivery promotes axonal regeneration in long gap peripheral nerve repair. Tissue Eng Part A. 2011;17:1263–75.
- Ghitza UE, Zhai H, Wu P, Airavaara M, Shaham Y, Lu L. Role of BDNF and GDNF in drug reward and relapse: a review. Neurosci Biobehav Rev. 2010;35:157–71.
- Uchida S, Hara K, Kobayashi A, Otsuki K, Yamagata H, Hobara T, . Epigenetic status of Gdnf in the ventral striatum determines susceptibility and adaptation to daily stressful events. Neuron. 2011;69:359–72.
- Gill SS, Patel NK, Hotton GR, O’Sullivan K, McCarter R, Bunnage M, . Direct brain infusion of glial cell line-derived neurotrophic factor in Parkinson disease. Nat Med. 2003;9:589–95.
- Nutt JG, Burchiel KJ, Comella CL, Jankovic J, Lang AE, Laws ER Jr, . Randomized, double-blind trial of glial cell line-derived neurotrophic factor (GDNF) in PD. Neurology. 2003;60:69–73.
- Love S, Plaha P, Patel NK, Hotton GR, Brooks DJ, Gill SS. Glial cell line-derived neurotrophic factor induces neuronal sprouting in human brain. Nat Med. 2005;11:703–4.
- Slevin JT, Gerhardt GA, Smith CD, Gash DM, Kryscio R, Young B. Improvement of bilateral motor functions in patients with Parkinson disease through the unilateral intraputaminal infusion of glial cell line-derived neurotrophic factor. J Neurosurg. 2005;102:216–22.
- Lang AE, Gill S, Patel NK, Lozano A, Nutt JG, Penn R, . Randomized controlled trial of intraputamenal glial cell line-derived neurotrophic factor infusion in Parkinson disease. Ann Neurol. 2006;59:459–66.
- Marks WJ Jr, Bartus RT, Siffert J, Davis CS, Lozano A, Boulis N, . Gene delivery of AAV2-neurturin for Parkinson's disease: a double-blind, randomised, controlled trial. Lancet Neurol. 2010;9:1164–72.
- Lonka-Nevalaita L, Lume M, Leppänen S, Jokitalo E, Peränen J, Saarma M. Characterization of the intracellular localization, processing, and secretion of two glial cell line-derived neurotrophic factor splice isoforms. J Neurosci. 2010;30:11403–13.
- Matsudaira P. Sequence from picomole quantities of proteins electroblotted onto polyvinylidene difluoride membranes. J Biol Chem. 1987; 262:10035–8.
- Parkash V, Leppänen VM, Virtanen H, Jurvansuu JM, Bespalov MM, Sidorova YA, . The structure of the glial cell line-derived neurotrophic factor-coreceptor complex: insights into RET signaling and heparin binding. J Biol Chem. 2008;283:35164–72.
- Eigenbrot C, Gerber N. X-ray structure of glial cell-derived neurotrophic factor at 1.9 A resolution and implications for receptor binding. Nat Struct Biol. 1997;4:435–8.
- Ansorena E, Garbayo E, Lanciego JL, Aymerich MS, Blanco-Prieto MJ. Production of highly pure human glycosylated GDNF in a mammalian cell line. Int J Pharm. 2010;385:6–11.
- Silvian L, Jin P, Carmillo P, Boriack-Sjodin PA, Pelletier C, Rushe M, . Artemin crystal structure reveals insights into heparan sulfate binding. Biochemistry. 2006;45:6801–12.
- Baloh RH, Tansey MG, Johnson EM Jr, Milbrandt J. Functional mapping of receptor specificity domains of glial cell line-derived neurotrophic factor (GDNF) family ligands and production of GFRalpha1 RET-specific agonists. J Biol Chem. 2000;275:3412–20.
- Horger BA, Nishimura MC, Armanini MP, Wang LC, Poulsen KT, Rosenblad C, . Neurturin exerts potent actions on survival and function of midbrain dopaminergic neurons. J Neurosci. 1998;18:4929–37.
- Hoane MR, Puri KD, Xu L, Stabila PF, Zhao H, Gulwadi AG, . Mammalian-cell-produced neurturin (NTN) is more potent than purified Escherichia coli-produced NTN. Exp Neurol. 2000;162:189–93.
- Fjord-Larsen L, Johansen JL, Kusk P, Tornøe J, Grønborg M, Rosenblad C, . Efficient in vivo protection of nigral dopaminergic neurons by lentiviral gene transfer of a modified Neurturin construct. Exp Neurol. 2005;195:49–60.
- Herzog CD, Dass B, Holden JE, Stansell J 3rd, Gasmi M, Tuszynski MH, . Striatal delivery of CERE-120, an AAV2 vector encoding human neurturin, enhances activity of the dopaminergic nigrostriatal system in aged monkeys. Mov Disord. 2007;22:1124–32.
- Suter-Crazzolara C, Unsicker K. GDNF is expressed in two forms in many tissues outside the CNS. Neuroreport. 1994;5:2486–8.
- Fletcher AM, Kowalczyk TH, Padegimas L, Cooper MJ, Yurek DM. Transgene expression in the striatum following intracerebral injections of DNA nanoparticles encoding for human glial cell line-derived neurotrophic factor. Neuroscience. 2011;194:220–6.
- Immonen T, Alakuijala A, Hytönen M, Sainio K, Poteryaev D, Saarma M, . A proGDNF-related peptide BEP increases synaptic excitation in rat hippocampus. Exp Neurol. 2008;210:793–6.
- Bradley LH, Fuqua J, Richardson A, Turchan-Cholewo J, Ai Y, Kelps KA, . Dopamine neuron stimulating actions of a GDNF propeptide. PLoS One. 2010;5:e9752.
- Xu RY, Pong K, Yu Y, Chang D, Liu S, Lile JD, . Characterization of two distinct monoclonal antibodies specific for glial cell line- derived neurotrophic factor. J Neurochem. 1998;70:1383–93.
- Alfano I, Vora P, Mummery RS, Mulloy B, Rider CC. The major determinant of the heparin binding of glial cell-line-derived neurotrophic factor is near the N-terminus and is dispensable for receptor binding. Biochem J. 2007;404:131–40.
- Haniu M, Hui J, Young Y, Le J, Katta V, Lee R, . Glial cell line- derived neurotrophic factor: selective reduction of the intermolecular disulfide linkage and characterization of its disulfide structure. Biochemistry. 1996;35:16799–805.
- Hui JO, Woo G, Chow DT, Katta V, Osslund T, Haniu M. The intermolecular disulfide bridge of human glial cell line-derived neurotrophic factor: its selective reduction and biological activity of the modified protein. J Protein Chem. 1999;18:585–93.
- Hoffer BJ, Hoffman A, Bowenkamp K, Huettl P, Hudson J, Martin D, . Glial cell line-derived neurotrophic factor reverses toxin-induced injury to midbrain dopaminergic neurons in vivo. Neurosci Lett. 1994;182:107–11.
- Aoi M, Date I, Tomita S, Ohmoto T. The effect of intrastriatal single injection of GDNF on the nigrostriatal dopaminergic system in hemiparkinsonian rats: behavioral and histological studies using two different dosages. Neurosci Res. 2000;36:319–25.
- Piltonen M, Bespalov MM, Ervasti D, Matilainen T, Sidorova YA, Rauvala H, . Heparin-binding determinants of GDNF reduce its tissue distribution but are beneficial for the protection of nigral dopaminergic neurons. Exp Neurol. 2009;219:499–506.
- Lu X, Hagg T. Glial cell line-derived neurotrophic factor prevents death, but not reductions in tyrosine hydroxylase, of injured nigrostriatal neurons in adult rats. J Comp Neurol. 1997;388:484–94.
- Kirik D, Georgievska B, Rosenblad C, Björklund A. Delayed infusion of GDNF promotes recovery of motor function in the partial lesion model of Parkinson's disease. Eur J Neurosci. 2001;13:1589–99.
- Hadaczek P, Johnston L, Forsayeth J, Bankiewicz KS. Pharmacokinetics and bioactivity of glial cell line-derived factor (GDNF) and neurturin (NTN) infused into the rat brain. Neuropharmacology. 2010;58:1114–21.
- Choi-Lundberg DL, Lin Q, Chang YN, Chiang YL, Hay CM, Mohajeri H, . Dopaminergic neurons protected from degeneration by GDNF gene therapy. Science. 1997;275:838–41.
- Li Q, Ping P, Jiang H, Liu K. Nerve conduit filled with GDNF gene-modified Schwann cells enhances regeneration of the peripheral nerve. Microsurgery. 2006;26:116–21.
- Kishima H, Poyot T, Bloch J, Dauguet J, Condé F, Dollé F, . Encapsulated GDNF-producing C2C12 cells for Parkinson's disease: a pre-clinical study in chronic MPTP-treated baboons. Neurobiol Dis. 2004;16:428–39.
- Sajadi A, Bensadoun JC, Schneider BL, Lo Bianco C, Aebischer P. Transient striatal delivery of GDNF via encapsulated cells leads to sustained behavioral improvement in a bilateral model of Parkinson disease. Neurobiol Dis. 2006;22:119–29.
- Grandoso L, Ponce S, Manuel I, Arrúe A, Ruiz-Ortega JA, Ulibarri I, . Long-term survival of encapsulated GDNF secreting cells implanted within the striatum of parkinsonized rats. Int J Pharm. 2007;343:69–78.