Abstract
Introduction. Dysregulation of circadian rhythms is a key symptom of mood disorders, including anxiety disorders and depression. Whether the circadian abnormalities observed in depressed patients are cause or consequence of the disease remains elusive. Here we aimed to explore potential disturbances of circadian rhythms in a validated genetic animal model of high trait anxiety and co-morbid depression and examine its molecular correlates.
Materials and methods. Mice selectively bred for high (HAB) and normal (NAB) anxiety- and co-segregating depression-like behavior were subjected to analysis of circadian wheel-running activity to determine light-entrained (LD) and free-running circadian (DD) rhythms and a light-induced phase shift. Clock gene expression in HAB/NAB hippocampal tissue was analyzed by qRT-PCR and verified by Western blotting.
Results. Compared to NABs, HAB mice were found to present with altered DD length of daily cycle, fragmented ultradiem rhythms, and a blunted phase shift response. Clock gene expression analysis revealed a selective reduction of Cry2 expression in hippocampal tissue of HAB mice.
Discussion. We provide first evidence for a dysregulation of circadian rhythms in a mouse model of anxiety and co-morbid depression which suggests an association between depression and altered circadian rhythms at the genetic level and points towards a role for Cry2.
Key messages
Aberrant display of circadian rhythms is observed for the first time in a selectively bred mouse line with high trait anxiety and co-morbid depression (HAB mice).
The circadian behavioral phenotype of HAB mice is associated with specific alterations in clock gene expression in the hippocampus.
Data obtained from this mouse model provide evidence for an association between depression and altered circadian rhythms at the genetic level and point towards a role for Cry2.
Introduction
The etiology of mood disorders, like most neuropsychiatric disorders, is most likely a multifactorial process, involving complex gene–environment interactions, and its molecular mechanisms still remain to be fully elucidated. One key symptom associated with mood disorders, including anxiety disorders and depression, is a dysregulation of biological rhythms manifested as sleep disturbances, alterations in the diurnal patterns of core body temperature, and hormonal secretion, such as cortisol (Citation1). Conversely, chronic disruption of sleep patterns is known as risk factor for the development of several neuropsychiatric illnesses, among those mood disorders (Citation2,Citation3).
Recently, several lines of evidence, mainly based upon genetic association studies, suggest that those deficiencies in rhythmic regulations observed in anxiety disorders and depression may not only result from the disease state but could even be relevant for the underlying pathophysiology (Citation4–6). However, a direct causal link between development of mood disorders and alterations in the circadian system is still elusive, mainly due to the lack of appropriate (animal) model systems.
Here we aimed to explore experimentally, in a genetic mouse model of high trait anxiety and co-morbid depression, a possible association between emotional and circadian dysregulation at the behavioral and molecular level. A mouse line, resulting from selective in-breeding of CD1 mice for high anxiety-related behavior (HAB) displayed on the elevated plus maze (Citation7) (for review see (Citation8,Citation9)) for more than 30 generations, is also characterized by high depression-like behavior as compared to normal anxiety-related behavior (NAB) controls. This was demonstrated in HAB versus NAB mice by the preference of immobility/passive stress-coping strategies in paradigms including forced swim and tail suspension test as well as clear signs of anhedonia assessed by the sucrose preference test (Citation10,Citation11). The high anxiety- and/or depression-related behavior of HAB mice can be normalized by diverse pharmacotherapeutic and invasive interventions including selective serotonin re-uptake inhibitors (Citation10), benzodiazepines (Citation12), and deep brain stimulation (Citation11). Interestingly, it has been recently described that HAB mice also present with reduced hippocampal neurogenesis (Citation9), generally accepted as one of the cellular correlates of depression-like behavior and deficient functional integration of the newly born cells (for review see (Citation13)). Stimulated by a study reporting that HAB mice display aberrant sleep patterns and alterations in EEG activities (Citation14), we assessed potential disturbances of circadian rhythms and finally aimed at examining their molecular correlates by analyzing the expression of core and tightly associated clock genes in the hippocampus, a brain region central to the neural circuitry of stress-related psychopathologies, including depressive disorders.
Materials and methods
Subjects
Female high (HAB) and normal (NAB) anxiety mice (12 weeks at the start of the experiments) were obtained from breeding colonies at the Department of Pharmacology and Toxicology, University of Innsbruck, Austria. Their anxious phenotype was confirmed by an elevated plus maze test at 7 weeks of age as previously described by Krömer et al. (Citation7).
All experiments were designed to reduce animal suffering and keep the number of animals used at the minimum level. Animal experiments described in this study were approved by the national ethical committee on animal care and use (Bundesministerium für Wissenschaft und Forschung) and carried out according to international laws and policies.
Housing
Animals were housed individually in Nalgene cages equipped with running wheels (15 cm in diameter; Actimetrics, Evanston, IL, USA) with food and water available ad libitum in a sound-attenuated room with constant temperature of ≈ 21°C. Animals were kept on a 12 h:12 h light:dark (LD12:12) cycle before experimental manipulations described below. During the light phase, light intensity at the level of the animals’ cages was ≈ 200 lux. During conditions of constant darkness (DD) cage cleaning and animal care taking was carried out under dim red light (15 W).
Locomotor activity assessment
Acquisition
Wheel revolutions were recorded with the ClockLab computer software, with 1-min sampling epochs (Actimetrics). Mice were initially placed in LD12:12 (lights on at 7 a.m.) for 13 days. On the 14th day, conditions were changed to 24 hours darkness (DD), and data acquisition was resumed for 10 days. On day 25, animals were exposed to a brief light pulse (30 minutes, 300 lux) at circadian time (CT) 16 (4 h after activity onset) for induction of a phase shift response. Consecutively, mice were maintained at DD for 7 more days before being switched back to LD for another 7 days prior to sacrification (). Brain dissections were carried out between 9 a.m. and 11 a.m.
Analysis
Activity was assessed and evaluated using the ClockLab software package (Actimetrics). Activity records were double-plotted in threshold format for 6-min bins. Activity onsets were determined using the default window settings of 6 h off and 6 h on. If the automatic detection selected as an onset a time clearly outside of the expected range and manual inspection identified an unambiguous onset bout, the onset time for that day was edited to an activity bout. Period measures were derived from regression lines fit to the activity onsets and used for calculation of chi-square periodograms. The free-running period for each animal was calculated from the days under DD prior to the light-pulse treatment. Phase shifts responses were evaluated by comparing the predicted activity onset for the day after the light pulse from extrapolated lines of the activity onsets of the days preceding the light pulse and the days after the pulse starting. All calculations and figures were derived from ClockLab software.
Gene expression analysis
Brain dissection
Subjects were sacrificed by neck dislocation, and brains were rapidly dissected over ice. Isolated hippocampal tissues were stored in RNA later (Ambion, Austria, Austin, TX, USA) at –20°C until used for RNA isolation or immediately immersed in liquid nitrogen and stored at –80°C for protein isolation.
Real time polymerase chain reaction (qRT-PCR)
Hippocampal RNA was isolated using miRNeasy kit (Qiagen®, USA, Hilden, Germany) according to the manufacturer's instructions. A 900 ng of total RNA was used for cDNA synthesis following manufacturer instructions provided with MMLV reverse transcriptase first-strand cDNA synthesis kit, G1 (Biozym®, Hessisch Oldendorf, Germany). A 1:5 dilution of cDNA reaction was used for PCR amplification using the Fast SYBR Green Mastermix (Applied Biosystems, Foster City, CA, USA) on a StepOnePlus realtime PCR system (serial no. 271000455; Applied Biosystems). Target genes were normalized to beta-actin. All primer sequences are listed in Supplementary Table I available online at http://informahealthcare.com/doi/abs/10.3109/07853890.2013.866440.
Protein isolation/protein quantification
Hippocampal tissue was powderized in liquid nitrogen and homogenized in a protein lysis buffer containing 10 mM Tris-HCl, pH 7.5, 150 mM NaCl, 1% SDS, 0.5% Triton X100, 1 mM EDTA, 10 mM NaF, 5 mM Na4O2P7, 10 mM Na3VO4 and protease inhibitor cocktail (1×, Roche Diagnostics, Mannheim, Germany). After sonication for approximately 5 cycles × 5s × 5, the suspension was left at 4°C on a rotator for 30 minutes and centrifuged at 14,000 g for 30 min at 4°C. The supernatant was immediately transferred and was quantified using Pierce BCA assay Kit (Thermo Scientific, Rockford, IL, USA). The standard curve was generated using bovine serum albumin ampules with a concentration of 2 mg/mL. The samples were analyzed in triplicate (microplate procedure: 25 μL sample + 200 μL BCA working reagent and incubated at 37°C for 30 minutes), and concentration was determined by absorbance reading at 595 nm using Synergy H4 Hybrid Reader spectrophotometer (Szabo-Scandic HandelsgmbH & Co KG, Vienna, Austria).
Western blotting
Samples (25 μg protein) were analyzed and loaded on a 10% sodium dodecyl sulfate (SDS) mini-gel (0.75 mm × 6.8 cm × 8.6 cm) and 5% stacking gel and then subjected to electrophoresis at 80 V for 1 hour and 45 min. Electrophoresis was performed with a Mini-Protean System (Bio-Rad Laboratories Inc., Vienna, Austria). Proteins from the gel were transferred onto PVDF membranes (Millipore, Billerica, MA, USA) and were run at 250 mA for 1 h 30 minutes. Membranes were blocked by incubating with 5% non-fat dry milk in 100 mM Tris, pH 7.5, 150 mM NaCl, and 0.1% Tween 20 (TTBS) for 1 h. Membranes were then incubated with diluted primary antibody (Rabbit Polyclonal to Cry2 (1:500); Abcam PLC, Cambridge, UK) overnight at 4°C, rinsed three times with TTBS, and incubated for 1 h at room temperature with horseradish peroxidase-conjugated secondary antibody (Goat Anti-rabbit HRP-linked IgG (1:3000); Cell Signaling Technology, Inc., Danvers, MA, USA). Immunoreactivity was visualized by enhanced chemiluminescence Pierce ECL substrate (Thermo Scientific). Detectable molecular masses were determined by running standard protein markers (Thermo Scientific) ranging from 10 to 250 kDa. Quantification was performed by chemiluminescent imaging with a FluorChem HD2 (Alpha Innotech, San Leandro, CA, USA) using the respective software. Values obtained from densitometry of target proteins were normalized to those of the housekeeping protein β-tubulin for the same samples.
Statistical analysis
For comparisons of behavioral data and gene expression results between HAB and NAB mice, Student t tests were carried out. Results were considered significant when P values were lower than 0.05. All statistical analyses were performed using BioStat software (AnalystSoft Inc., Alexandria, VA, USA).
Results
HAB mice show abnormal circadian period (tau) under free-running conditions
In order to examine whether selective breeding for high anxiety and co-morbid depression may in parallel lead to in vivo consequences in alterations in circadian behavior, the wheel-running rhythms of HAB mice were compared to those of NAB mice.
In free-running conditions (constant darkness, DD), HAB mice displayed a significantly longer free-running period (tau) than NAB females (P < 0.05) (), while no differences were observed under LD conditions (). However, no differences in total wheel revolutions per day, nor individually in the rho- or alpha-phase, respectively, were observed under either LD or DD conditions ( and D), suggesting that alterations in tau during DD in HAB mice do not result from effects on overall locomotor activity.
Figure 2. Alterations of the free-running circadian rhythm observed in HAB mice. (A) HAB mice display a significantly longer circadian period under free-running conditions (DD) than NAB mice. (B) No differences are observed under light-entrained conditions (LD). (C) and (D) HAB and NAB mice show comparable amounts of wheel running activity under DD (C) and LD (D) conditions during both their active (alpha) and inactive (rho) phases. *P < 0.05; data displayed as mean ± SEM.
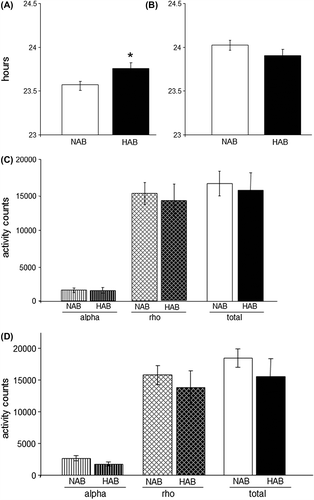
Fragmented ultradiem rhythms under LD and DD conditions
In HAB mice the actograms generated from wheel-running behavior appeared to be fragmented as compared with those derived from NAB mice, suggesting potential alterations of ultradiem rhythms in the HAB model (). This observation was further investigated by analysis of activity bouts and indeed revealed fragmented ultradiem rhythms as manifested by a significantly higher number of activity bouts in HAB females under LD and DD conditions (P < 0.05) ( and C).
Figure 3. HAB mice display fragmented ultradiem rhythms. (A) Sample double-plotted actograms depicting wheel-running activity in NAB (left) and HAB (right) mice. Individual days are represented by horizontal rows with black vertical bars indicating locomotor activity (wheel revolutions). (B) and (C) HAB mice show significantly more activity bouts under both LD (B) and DD (C) conditions. * P < 0.05; data displayed as mean ± SEM.
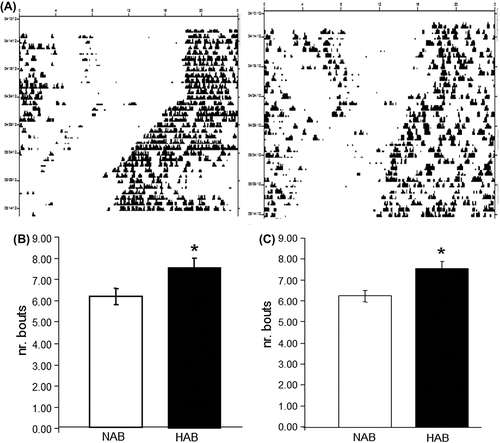
HAB mice display deficient entrainment to light
We next examined light-induced clock entrainment in HAB mice using light-induced phase shift as paradigm assessing the responsiveness of the endogenous circadian rhythms to exogenous zeitgeber. To this end, mice were exposed to a brief light pulse (30 minutes, 300 lux) in the early night (CT 16) for induction of a phase shift response. A dramatic and significant reduction (P < 0.001) of the mean phase delay induced by this light treatment was observed in HAB mice ().
Figure 4. Phase shift response is blunted in HAB mice. (A) A light-induced phase shift at CT 16 reveals a significantly diminished response in HAB mice as evidenced by a more than 50% reduction in the light-induced phase delay (hours). (B) and (C) Expression of Cry2 is significantly reduced at the mRNA level (B) and the protein level (C) in hippocampal tissue of HAB compared to NAB mice. *P < 0.05, **P < 0.01, ***P < 0.001; data displayed as mean ± SEM.
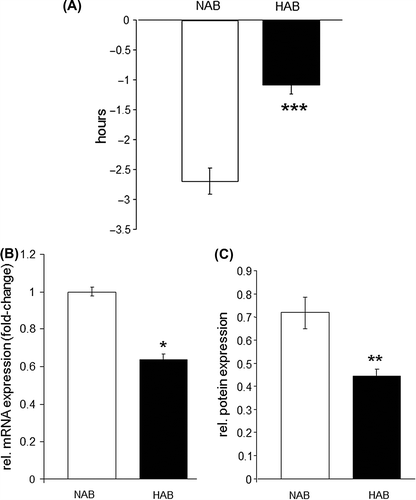
Hippocampal levels of Cry2 are altered in HAB mice
We further aimed to investigate the potential mechanisms underlying the observed circadian behavioral phenotype at the molecular level by analyzing clock gene expression in hippocampal tissue of HAB and NAB mice. A qRT-PCR analysis of Clock, Per1–3, Bmal, Npas2, Cry1–2, Rev-erb α-β, Ror α-β-γ, Dec1/2, E4bp4, NeuroD1, CycloB, and Dbp revealed a selective reduction of Cry2 mRNA levels in the hippocampus of HAB compared to NAB mice (P < 0.05) (), which was further verified at the protein level using Western blot (P < 0.01) (). In order to examine whether the observed changes in Cry2 expression were specific to the hippocampus or also occurred in another brain region relevant to the neural circuitry associated with mood and affective disorders, Cry2 levels were also compared in frontal cortical tissue of HAB and NAB mice. No significant expressional differences between the two mouse lines were observed (P > 0.05).
Discussion
We here show for the first time disturbances of the circadian rhythm in a genetic mouse model of high trait anxiety and co-morbid depression and propose derangement of hippocampal clock gene expression as molecular correlate.
Mood disorders, including major depression, are tightly associated with alterations in the circadian rhythm, including disturbances of sleep which are even listed as diagnostic criteria for depression in the fourth edition of the Diagnostic and Statistical Manual of Mental Disorders (Citation15). Moreover, a genetic basis for the involvement of the circadian system in mood disorders is suggested by a series of association studies identifying polymorphisms in clock genes in patients suffering from bipolar disorder, seasonal affective disorder (SAD), and major depression (Citation5,Citation16–25). These findings are now complemented with the result of the present study, firstly using a mouse model, indicating that innate high levels of trait anxiety and depression-like behavior may lead to and/or share a common genetic basis with disturbed circadian rhythms.
The lengthened free-running circadian period tau in HAB mice indicates that the derangement of the circadian behavior is likely due to a dysfunction of the endogenous circadian rhythm in these animals, most likely originating from a compromise in the molecular circadian machinery orchestrating the circadian rhythm under DD conditions. Modulation of the free-running circadian period in mice and other laboratory rodents has been reported under several experimental conditions, including ageing (Citation26), exposure to ethanol (Citation27–29) and selective breeding for ethanol-related traits (Citation30), various knock-out mouse models for core clock genes (Citation31–33), as well as candidate genes related to psychiatric disorders. Interestingly, we had previously observed that long-term exposure to constant darkness in mice also lengthens the circadian period without affecting total activity levels and that this modulation of tau is paralleled by depression-like behavior (Citation34).
The increase in the number of activity bouts in HAB mice revealed in the present study complements previous findings characterizing the sleep phenotype of this mouse line (Citation14). Paralleling our own results, it is reported therein that HAB mice exhibited an increase in the number of bouts of wakefulness with recurrent entries to non-REM and REM sleep episodes and shorter episodes of non-REM and REM sleep and an enhancement of all state transitions characterized as sleep fragmentation (Citation14).
Our observation of an attenuated phase shift response induced by a light pulse in the early subject night (CT 16) – known to induce phase delays (Citation35) – suggests a deficiency of the endogenous circadian machinery to the entrainment by external stimuli in HAB mice. However, given a stable circadian period under LD conditions, there is no indication for a general light insensitivity in HAB mice. The molecular mechanisms involved in light-induced clock resetting, as experimental paradigm assessing the critical capability of the endogenous clock to be entrained by external stimuli, thus responding to changing environmental settings, still remain poorly understood (Citation36). While a role for several clock genes, including per1, per2, and cry2 have been described, also several non-clock genes have been lately implicated in mediating light-entrainment (Citation37).
Trying to elucidate the molecular mechanisms potentially underlying the observed circadian phenotype in HAB mice, we focused on investigating the expression of the elements of the endogenous circadian machinery in the hippocampus. While the suprachiasmatic nucleus (SCN) is the locus of central circadian orchestration, other areas of the brain, including some highly implicated in mood disorders such as the hippocampus, also display clock gene expression (Citation38). It can be speculated that clock gene dysfunction in these extra-SCN sites may directly relate to the pathomechanisms of mood disorders. When analyzing the expression of 21 molecules forming part of the cellular clock we had found a selective reduction of Cry2 expression in hippocampal tissue of HAB mice, both at the mRNA and protein level. Interestingly, no differences have been observed between HAB and NAB mice in the expression of Cry2 in the frontal cortex, another brain region forming part of the neural network whose dysfunctionality relates to mood disorders (see for review (Citation39)). This observation suggests that the results obtained from the hippocampus present a region-specific finding and points toward a role of Cry2 in the regulation of selective functions of the hippocampus potentially altered in mood and anxiety disorders and respective animal models. Previously, a role of Cry2 in the pathophysiology of depression has been proposed based upon the identification of four CRY2 SNPs identified from the human genome and their association with mood disorders (Citation23,Citation24,Citation40) as well as findings from a pharmacogenomic mouse model (Citation41). However, to the best of our knowledge, the present study is the first to reveal specific expressional changes of Cry2 in a mouse model of anxiety and co-morbid depression also displaying alterations of the behavioral circadian rhythm. A potential behavioral phenotype related to depression and anxiety still remains to be tested in Cry2-KO to be able to assign a direct causal relationship between Cry2 and depression, and potential Cry2 SNPs in HAB mice should be analyzed in future studies.
The present study has several limitations: First, the use of bidirectionally bred mouse lines possesses inherent conceptual restrictions, limiting the interpretation of results obtained using these animal models. As such, the possibility that altered Cry2 levels in HAB mice represent an incidental molecular phenotype resulting from co-selection with the phenotype of interest cannot be excluded (e.g. (Citation42)). Second, clock gene expression has been analyzed at a single time point; thus it remains to be investigated whether Cry2 overall levels are down-regulated in HAB mice or whether the peak of the Cry2 expression is shifted. Third, the results of hippocampal clock gene expression have not yet been related to those of the SCN which would allow the nature of circadian dysregulation in HAB mice to be understood in further detail.
In summary, we here provide experimental evidence that genetic selection for high anxiety-like and depression-like behavior alters the behavioral circadian rhythm and compromises the expression of a core element of the molecular circadian machinery. These results propose a potential linkage between emotional and circadian dysregulation at the genetic level and provide support for the hypothesis that circadian abnormalities observed in patients suffering from affective disorders may not be a consequence of the disease but rather suggest a potential involvement in the underlying pathomechanisms. Moreover, results of this study recommend the HAB mouse line as novel animal model for investigating circadian abnormalities in mood and anxiety disorders.
Supplementary Table I
Download PDF (34 KB)Declaration of interest: Daniela D. Pollak is supported by the Austrian Science Fund (FWF): P22424 and member of the special research network (SFB) 35. Nicolas Singewald is funded by the Austrian Science Fund (FWF): P22931-B18 B18 and member of the special research network (SFB) 44. The authors report no conflicts of interest.
References
- Gonik M, Frank E, Kessler MS, Czamara D, Bunck M, Yen YC, et al. The endocrine stress response is linked to one specific locus on chromosome 3 in a mouse model based on extremes in trait anxiety. BMC Genomics. 2012;13:579.
- Spiegelhalder K, Regen W, Nanovska S, Baglioni C, Riemann D. Comorbid sleep disorders in neuropsychiatric disorders across the life cycle. Curr Psychiatry Rep. 2013;15:364.
- Krystal AD, Thakur M, Roth T. Sleep disturbance in psychiatric disorders: effects on function and quality of life in mood disorders, alcoholism, and schizophrenia. Ann Clin Psychiatry. 2008;20:39–46.
- Serretti A, Benedetti F, Mandelli L, Lorenzi C, Pirovano A, Colombo C, et al. Genetic dissection of psychopathological symptoms: insomnia in mood disorders and CLOCK gene polymorphism. Am J Med Genet B Neuropsychiatr Genet. 2003;121B:35–8.
- Partonen T, Treutlein J, Alpman A, Frank J, Johansson C, Depner M, et al. Three circadian clock genes Per2, Arntl, and Npas2 contribute to winter depression. Ann Med. 2007;39:229–38.
- Sipila T, Kananen L, Greco D, Donner J, Silander K, Terwilliger JD, et al. An association analysis of circadian genes in anxiety disorders. Biol Psychiatry. 2010;67:1163–70.
- Kromer SA, Kessler MS, Milfay D, Birg IN, Bunck M, Czibere L, et al. Identification of glyoxalase-I as a protein marker in a mouse model of extremes in trait anxiety. J Neurosci. 2005;25:4375–84.
- Landgraf R, Kessler MS, Bunck M, Murgatroyd C, Spengler D, Zimbelmann M, et al. Candidate genes of anxiety-related behavior in HAB/LAB rats and mice: focus on vasopressin and glyoxalase-I. Neurosci Biobehav Rev. 2007;31:89–102.
- Sartori SB, Landgraf R, Singewald N. The clinical implications of mouse models of enhanced anxiety. Future Neurol. 2011;6:531–71.
- Sah A, Schmuckermair C, Sartori SB, Gaburro S, Kandasamy M, Irschick R, et al. Anxiety- rather than depression-like behavior is associated with adult neurogenesis in a female mouse model of higher trait anxiety- and comorbid depression-like behavior. Transl Psychiatry. 2012;2:e171.
- Schmuckermair C, Gaburro S, Sah A, Landgraf R, Sartori SB, Singewald N. Behavioral and neurobiological effects of deep brain stimulation in a mouse model of high anxiety- and depression-like behavior. Neuropsychopharmacology. 2013;38:1234–44.
- Sartori SB, Hauschild M, Bunck M, Gaburro S, Landgraf R, Singewald N. Enhanced fear expression in a psychopathological mouse model of trait anxiety: pharmacological interventions. PLoS One. 2011;6:e16849.
- Sahay A, Hen R. Adult hippocampal neurogenesis in depression. Nat Neurosci. 2007;10:1110–15.
- Jakubcakova V, Flachskamm C, Landgraf R, Kimura M. Sleep phenotyping in a mouse model of extreme trait anxiety. PLoS One. 2012;7:e40625.
- American Psychiatric Association APATFoDSMIV. Diagnostic and statistical manual of mental disorders: DSM-IV-TR. Washington, DC: American Psychiatric Association; 2000.
- Benedetti F, Serretti A, Colombo C, Barbini B, Lorenzi C, Campori E, et al. Influence of CLOCK gene polymorphism on circadian mood fluctuation and illness recurrence in bipolar depression. Am J Med Genet B Neuropsychiatr Genet. 2003;123B:23–6.
- Johansson C, Willeit M, Levitan R, Partonen T, Smedh C, Del Favero J, et al. The serotonin transporter promoter repeat length polymorphism, seasonal affective disorder and seasonality. Psychol Med. 2003;33: 785–92.
- Mansour HA, Wood J, Logue T, Chowdari KV, Dayal M, Kupfer DJ, et al. Association study of eight circadian genes with bipolar I disorder, schizoaffective disorder and schizophrenia. Genes Brain Behav. 2006; 5:150–7.
- Benedetti F, Dallaspezia S, Colombo C, Pirovano A, Marino E, Smeraldi E. A length polymorphism in the circadian clock gene Per3 influences age at onset of bipolar disorder. Neurosci Lett. 2008;445:184–7.
- Kishi T, Ikeda M, Kitajima T, Yamanouchi Y, Kinoshita Y, Kawashima K, et al. Association analysis of functional polymorphism in estrogen receptor alpha gene with schizophrenia and mood disorders in the Japanese population. Psychiatr Genet. 2009;19:217–18.
- Kripke DF, Nievergelt CM, Joo E, Shekhtman T, Kelsoe JR. Circadian polymorphisms associated with affective disorders. J Circadian Rhythms. 2009;7:2.
- Mansour HA, Talkowski ME, Wood J, Chowdari KV, McClain L, Prasad K, et al. Association study of 21 circadian genes with bipolar I disorder, schizoaffective disorder, and schizophrenia. Bipolar Disord. 2009;11:701–10.
- Lavebratt C, Sjoholm LK, Soronen P, Paunio T, Vawter MP, Bunney WE, et al. CRY2 is associated with depression. PLoS One. 2010;5:e9407.
- Sjoholm LK, Backlund L, Cheteh EH, Ek IR, Frisen L, Schalling M, et al. CRY2 is associated with rapid cycling in bipolar disorder patients. PLoS One. 2010;5:e12632.
- Soria V, Martinez-Amoros E, Escaramis G, Valero J, Perez-Egea R, Garcia C, et al. Differential association of circadian genes with mood disorders: CRY1 and NPAS2 are associated with unipolar major depression and CLOCK and VIP with bipolar disorder. Neuropsychopharmacology. 2010;35:1279–89.
- Valentinuzzi VS, Scarbrough K, Takahashi JS, Turek FW. Effects of aging on the circadian rhythm of wheel-running activity in C57BL/6 mice. Am J Physiol. 1997;273(6 Pt 2):R1957–64.
- Mistlberger RE, Nadeau J. Ethanol and circadian rhythms in the Syrian hamster: effects on entrained phase, reentrainment rate, and period. Pharmacol Biochem Behav. 1992;43:159–65.
- Dwyer SM, Rosenwasser AM. Neonatal clomipramine treatment, alcohol intake and circadian rhythms in rats. Psychopharmacology (Berl). 1998;138:176–83.
- Rosenwasser AM, Fecteau ME, Logan RW. Effects of ethanol intake and ethanol withdrawal on free-running circadian activity rhythms in rats. Physiol Behav. 2005;84:537–42.
- McCulley WD 3rd, Ascheid S, Crabbe JC, Rosenwasser AM. Selective breeding for ethanol-related traits alters circadian phenotype. Alcohol. 2013;47:187–94.
- Thresher RJ, Vitaterna MH, Miyamoto Y, Kazantsev A, Hsu DS, Petit C, et al. Role of mouse cryptochrome blue-light photoreceptor in circadian photoresponses. Science. 1998;282:1490–4.
- Shearman LP, Jin X, Lee C, Reppert SM, Weaver DR. Targeted disruption of the mPer3 gene: subtle effects on circadian clock function. Mol Cell Biol. 2000;20:6269–75.
- Nakamura T, Takumi T, Takano A, Hatanaka F, Yamamoto Y. Characterization and modeling of intermittent locomotor dynamics in clock gene-deficient mice. PLoS One. 2013;8:e58884.
- Monje FJ, Cabatic M, Divisch I, Kim EJ, Herkner KR, Binder BR, et al. Constant darkness induces IL-6-dependent depression-like behavior through the NF-kappaB signaling pathway. J Neurosci. 2011;31: 9075–83.
- Daan S, Pittendrigh C. A functional analysis of circadian pacemakers in nocturnal rodents. Journal of Comparative Physiology. 1976;106: 253–66.
- Meijer JH, Schwartz WJ. In search of the pathways for light-induced pacemaker resetting in the suprachiasmatic nucleus. J Biol Rhythms. 2003;18:235–49.
- Hannibal J, Jamen F, Nielsen HS, Journot L, Brabet P, Fahrenkrug J. Dissociation between light-induced phase shift of the circadian rhythm and clock gene expression in mice lacking the pituitary adenylate cyclase activating polypeptide type 1 receptor. J Neurosci. 2001;21: 4883–90.
- Li JZ, Bunney BG, Meng F, Hagenauer MH, Walsh DM, Vawter MP, et al. Circadian patterns of gene expression in the human brain and disruption in major depressive disorder. Proc Natl Acad Sci U S A. 2013;110: 9950–5.
- Price JL, Drevets WC. Neural circuits underlying the pathophysiology of mood disorders. Trends Cogn Sci. 2012;16:61–71.
- Partonen T. Clock gene variants in mood and anxiety disorders. J Neural Transm. 2012;119:1133–45.
- Ogden CA, Rich ME, Schork NJ, Paulus MP, Geyer MA, Lohr JB, et al. Candidate genes, pathways and mechanisms for bipolar (manic- depressive) and related disorders: an expanded convergent functional genomics approach. Mol Psychiatry. 2004;9:1007–29.
- Distler MG, Palmer AA. Role of glyoxalase 1 (Glo1) and methylglyoxal (MG) in behavior: recent advances and mechanistic insights. Front Genet. 2012;3:250.