Abstract
Artificial light decreases the amplitude of daily rhythms in human lifestyle principally by permitting activity and food intake to occur during hours of darkness, and allowing day-time activity to occur in dim light, indoors. Endogenous circadian timing mechanisms that oscillate with a period of 24 h have evolved to ensure physiology is synchronized with the daily variations in light, food, and social cues of the environment. Artificial light affects the synchronization between these oscillators, and metabolic disruption may be one consequence of this. By dampening the amplitude of environmental timing cues and disrupting circadian rhythmicity, artificial lighting might initiate metabolic disruption and contribute to the association between global urbanization and obesity. The aim of this review is to explore the historical, physiological, and epidemiological relationships between artificial light and circadian and metabolic dysfunction.
Key words::
Key messages
Artificial light affects the rhythmicity of human lifestyle by facilitating increased work, sleep, and eating in hours of darkness.
Disruption to circadian rhythmicity causes metabolic dysfunction and weight-gain in animals.
Disruption of circadian rhythms, and of synchronization between endogenous and environmental cycles, might contribute to the detrimental effect of urbanization on human metabolic health.
A journey through time: dampening of the rhythmicity of human life
The availability of artificial light marked a milestone in human evolution, facilitating manipulation of the daily light–dark cycle, illuminating feeding and social interactions, and extending waking hours into the night. Candlelight was progressively supplemented by gas lighting, and the early nineteenth century marked a turning-point in human light exposure when artificial lighting became both efficient and affordable, finally ending dependence on the light of the sun ().
Figure 1. The year 1800 is taken as a turning-point in the development of human reliance on artificial light. This period marked the beginning of the industrial revolution, and also when artificial light provided by candles, gas, and finally electricity became efficient and affordable. A logarithmic scale is required to allow comparison across the ages, also illustrating the large magnitude of the change in light consumption that occurred over a relatively short time period. A lumen hour is defined as the luminous energy equal to that emitted in 1 hour by a light source emitting a luminous flux of 1 lumen. This graph is redrawn from data reported by Fouquet and Pearson, 2006 (Citation89).
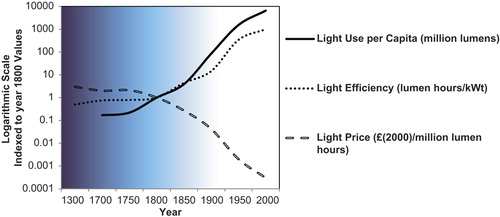
In 1879, Thomas Edison announced one of the first electric light bulbs, which was indeed ‘the best artificial light ever known ...’ (), quickly swamping all preceding exposure to man-made light (), and eliminating darkness from the human night. Electric light allowed humans to override the strong rhythmicity that had pervaded life for millennia. The times reserved for eating, sleeping, and exercising were now malleable. The rhythmicity of human lifestyle was eroded by artificial night-light, and it slipped away largely unrecorded.
Figure 2. Advertisement for Edison Electric Illuminating Company of Brooklyn printed in the Brooklyn Institute of Arts and Sciences Bulletin, Volume 3, no. 16, December 25, 1909 and reproduced here with kind permission of the Brooklyn Museum Library.
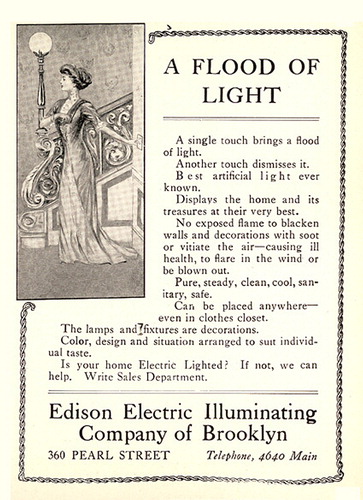
One specific effect of artificial lighting was to consolidate the Winter night, shortening the hours of darkness to those of Summer and enabling evening-time activity. An immediate implication of this was the demise of a human biphasic sleep pattern which is thought to have been a common feature of the sleep patterns of medieval Europeans (Citation1). The biphasic pattern of Winter sleep consisted of two sleep periods with an intervening ‘quiet time’ around midnight which medieval man reserved for reading by candlelight, thinking, or praying (Citation1). Many other animals show similar splitting of Winter activity patterns (), as do humans placed on 10–16-h night-time darkness in the laboratory (Citation2,Citation3), and these patterns may reflect the underlying regulation of dual oscillators (Citation4). Splitting of the sleep pattern is probably a response to the long Winter night, rather than the emergence of an endogenous pattern, since the human sleep period is generally monophasic in constant darkness, and humans with enforced biphasic sleep patterns report increased sleep disturbances (Citation5). Split sleep patterns in response to long nights, as well as the consolidation of Winter sleep patterns by night-light, illustrate the capacity of the human clock to adapt circadian rhythms to accommodate photoperiodic conditions. Artificial night-light has long since eradicated the midnight interval for quiet reflection, and the two sleeps of the Winter night of our ancestors, and the consequences of this, if there are any, are unknown.
Figure 3. Biphasic sleep patterns are a feature of short photoperiod circadian rhythms in sheep (Wyse et al., unpublished data) and in humans sleeping in 10:14 L:D cycles in a laboratory, left (redrawn from data reported by Wehr et al., 1993 (Citation2)). This is in contrast to the single, consolidated sleep period, the Summer short nights. Note that the human data are sleep-recorded by EEG, and sheep data are locomotor activity.
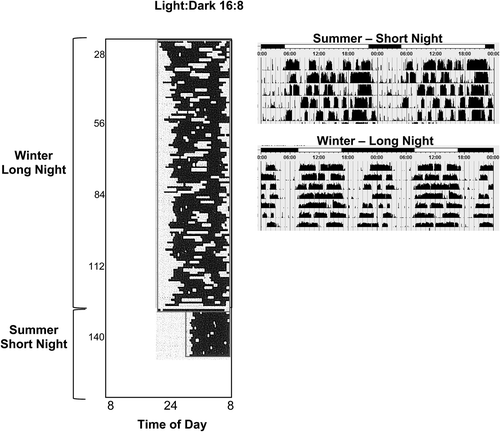
Urbanization: dumbing down daily rhythmicity
In addition to disruption of circadian rhythms, and termination of human seasonal sleep patterns, artificial light further affects photoperiodic timing of physiology by reducing overall light exposure. Work and leisure activities are increasingly based indoors, causing a reduction in day-time exposure to sunlight (Citation6). Artificial lighting has created a modern photoperiod of relatively brighter nights and dimmer days, with a net effect of dampening the photic signal delivered to circadian timing centres. Changes in the circadian patterns of light exposure induced by artificial light were recently quantified in the same individuals living outdoors with no access to artificial light, and during their normal Western lives (Citation6). A striking decrease in day-time light exposure was evident in the urban environment, as well as the extension of activity well into the hours of darkness (). Access to the internet and PCs has removed temporal boundaries to work and social interactions, so that even non-photic zeitgebers are losing their restriction to daylight hours. For example, human social interaction is a non-photic timing cue which is affected by ‘social media’ (internet, twitter), which supports communication during sleeping hours, even with those in their waking hours on the opposite hemisphere. Together with dampening of the photoperiodic signal, and erratic meal-timing, these factors converge in the urban environment to compromise the entraining signals to the human circadian clock. Therefore, any potential detrimental effects of night-light are likely not simply a consequence of shorter hours of darkness, but rather a general disruption of the temporal architecture of human life.
Figure 4. Comparison of light exposure in Western lifestyle (orange) and camping in a mountainous region with no artificial light. Note the significant extension of artificial light into the dark period (arrow), as well as an overall reduction of total light exposure due to the dim days of artificial lighting. Reproduced with permission from Wright et al., 2013 (Citation6).
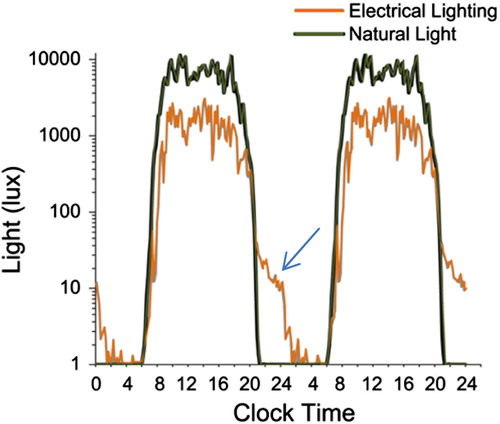
Research in the last two decades now supports that loss of human rhythmicity facilitated by artificial light has detrimental consequences for physical and mental health. Much of this research has focused on shift-workers, whose work schedules disrupt the synchronization between environmental (photoperiod, social cues) and physiological (sleep, neuroendocrine) rhythms. These humans with severely disrupted rhythmicity have increased risks of metabolic dysfunction, obesity, and prostate and breast cancer (Citation7–9). The effects of loss of rhythmicity may be responsible for metabolic dysfunction among the non-shift-working population too. Urbanization and attenuation of the rhythmicity of human lifestyle has occurred in parallel with a global increase in the prevalence of obesity. It is likely that complex and bidirectional relationships between obesity, diet, and exercise interact in the urban environment to induce metabolic disease. Artificial light could contribute to these effects primarily by affecting the amplitude of the photoperiodic zeitgeber, (dim days and brighter nights), but also by facilitating night-time ingestion of food, social interactions, exercise, and other zeitgebers that were once largely reserved to waking, daylight hours. The rate of global obesity has doubled since 1980 (Citation10), while the UK government predicts that around half (47%) of its male population will be obese in 2015 (Citation11). Obesity associated with urbanization often occurs as part of a constellation of metabolic dysfunction (high fasting glucose and systolic blood pressure, dyslipidaemia, inflammation, and insulin insensitivity), termed the metabolic syndrome. Electric lighting could conceivably contribute to the increased incidence of obesity via its role in facilitating the 24-h lifestyle of the urban environment and the disrupted rhythmicity that this implies. The aim of this review is to address this question by examining the evidence supporting the detrimental effects of artificial light on metabolic health and obesity.
Night-light, loss of rhythmicity, and metabolic dysfunction—when did it happen?
Candle and gas lighting has influenced human night-time activity for centuries, but increased metabolic disruption and obesity are relatively recent trends. It might be argued that if artificial night-light was a direct cause of metabolic dysfunction then the current epidemic of obesity and diabetes might have more closely approximated the increased use of lighting in the nineteenth century (). However, the effect of night-light on circadian rhythmicity was most likely gradual and progressive; patterns of meal, work, and exercise times were so engrained in human culture that the freedom to change these might not lead to an immediate shift. That change occurred more gradually throughout the last century as progressive generations became more tolerant of changes in sleeping, eating, and activity patterns. Remarkably, the considerable changes to the rhythmicity of human lifestyle over the last century are largely undocumented and unrecorded. There are few longitudinal data available on changes in circadian rhythmicity of human behaviours such as shopping, sleeping, studying, or even eating or working. It is only in recent decades that it has become acceptable to shop, watch TV, and even exercise in the early hours of the morning. Studies of trends in human night-time activity are now important to document the time-scale of degeneration of rhythmicity. One example of such data is shown in : the library opening hours of the University of Aberdeen, one of the oldest universities in the UK, extended gradually into the hours of darkness over the latter half of the twentieth century until 2010, when students studied well into the hours previously reserved for sleep. Note that the change in opening hours did not coincide with the availability of electric light, but rather occurred gradually over the latter half of the twentieth century, supporting that rhythmicity was progressively eroded as activity at night became increasingly acceptable. The extended opening hours are just one example of an increasing demand for services to support activity at night, and an indication of a longitudinal change in the temporal structure of human lifestyle. The emerging association of human rhythmicity with metabolic health justifies further study of changes to the patterns of eating, work, and exercise of humans over the last century.
Figure 5. Summer term opening hours of the University of Aberdeen's library provide evidence of increasing extension of study time into the dark hours of night since electric light became available (Wyse et al., unpublished data).
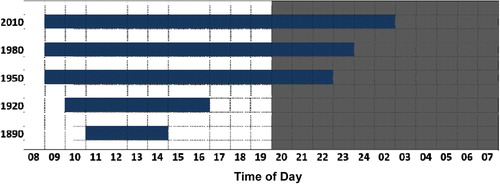
Artificial night-light facilitates night-time activity, and it may also serve as a proxy variable to monitor circadian disruption. For example, the pattern of daily consumption of domestic electricity supply is an interesting indicator of human activity, with a morning peak coinciding with the time of awakening, an evening peak that represents night-time activity; and an additional mid-day peak for lunchtime food preparation (Citation12). Domestic electricity load profiles are widely reported, and show interesting correlates with the human circadian activity pattern. For example, the daily load profile in shows a typical progressive phase delay over the weekend that has been well described using standard chronobiological techniques to measure human rhythmicity (Citation13). On a wider scale, the use of electric lighting and appliances has increased in the UK since 1970, coincident with increased prevalence of obesity (), supporting at least an associative relationship between the use of artificial light and metabolic dysfunction. Nevertheless, the possibility that disruption of rhythmicity and artificial night-light are simply markers of urbanization with no causal effect cannot be excluded by retrospective data analyses. Detailed prospective studies are required to investigate exactly how associations between lighting and appliance use at night relate to changes in metabolism and body weight. Most importantly, we must first understand the physiological mechanisms through which disrupted rhythmicity initiates disordered metabolism and obesity. These mechanisms hold the key to interpreting the significance of associations between artificial night-light and health, and are reviewed in the next sections.
Figure 6. Relationship between lighting use and obesity. A: Average daily load profile for electricity demand in the UK (2009–2011; Winter) showing an early morning peak associated with waking, and an evening peak representing evening activities. Lunch time also is also represented by increased electricity consumption. Notice the progressive phase delay of the peak for wake time electricity use that occurs over the weekend, a typical human pattern of activity. B: Domestic energy consumption for lighting and appliances has increased in the UK, in parallel with increased prevalence of obesity. (Data sources: WHO Global Infobase https://apps.who.int/infobase/Index.aspx and Energy Consumption in the UK (ECUK) UK Government Department of Energy and Climate Change, Publication URN 13D/157, 2013.).
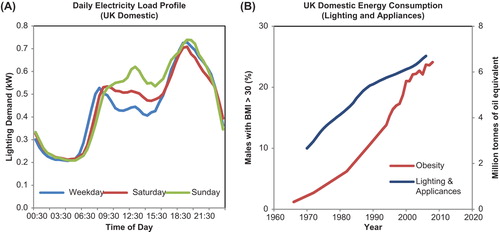
Rhythmicity and metabolic control: failure of physiological synchronicity
Circadian rhythms are defined as daily cycles in physiological and behavioural parameters that are generated endogenously, and that recur with a period approximating 24 h. Circadian rhythms are generated through transcription and translational feedback loops in a panel of specific ‘clock’ genes (e.g. Clock, Bmal1, Per1, Per2, Per3, Cry1, Cry2) which are expressed in virtually every human cell, and regulate hundreds of downstream pathways (Citation14). The basic clock gene mechanism involves a feedback loop that centres on induction of Per and Cry gene expression by the protein dimer product of Clock and Bmal1. The proteins expressed by Per and Cry genes inhibit the activity of the Clock and Bmal1 complex, providing corresponding negative feedback to this system. This clock mechanism transduces timing information by regulating the transcription of many further clock-controlled genes. Indeed, it is estimated that at least 10% of the mammalian peripheral transcriptome is clock-controlled (Citation15). Central control of circadian timing is maintained by a pair of nuclei in the anterior hypothalamus, the suprachiasmatic nuclei (SCN), which control the entrainment of a network of peripheral and central clocks to light and other environmental time-givers, or zeitgebers. The SCN receive photic information from the mammalian eye via photosensitive retinal ganglion cells that express the photopigment, melanopsin (Citation16). The human clock has an innate period close to 24 h to which circadian rhythms revert in conditions of constant darkness and in the absence of all time cues. This endogenous period is called tau, and under entrained conditions it synchronizes to the period of environmental cues provided by the light–dark cycle, and also by food, exercise, and social interactions. Through these mechanisms, circadian rhythms regulate adaptation by controlling entrainment and synchronization of physiology to predictable environmental change.
Exposure to disrupted photoperiods results in desynchronization of endogenous and environmental circadian rhythms, termed ‘circadian desynchrony’ (CD). The types of light disruption that are represented by CD are diverse and poorly defined. Experimental studies apply acute and chronic phase advance or delay to represent ‘jet lag’ and shift-work, respectively. Exposure of animals to light at night, or constant light is also termed CD, as is accommodation in photoperiods with periods not equal to 24 h. ‘Shift-work’ is simulated in some animal models by engaging the animals in gentle activity (walking, or handling) during their inactive period. These diverse models of CD may affect physiology and behaviour in different ways, and a challenging task for the chronobiologists of the future is to determine and define which model most closely represents disruption of human rhythmicity.
The human circadian phenotype shows considerable inter-subject variation, and the terms ‘late’ and ‘early chronotype’ represent the individual preference for the timing of daily activities, and the timing of physiological functions. There is good evidence to associate the late chronotype with increased susceptibility to metabolic dysfunction (Citation17,Citation18), but the relationship is confounded by differences in behaviour between the chronotypes (Citation19). Chronotype reflects functional variability of the human circadian clock and possibly individual susceptibility to metabolic effects of CD.
Genetic models have proved very informative in elucidating relationships between metabolism and the circadian timing mechanisms. Clock gene mutant animals have disrupted circadian rhythmicity as might be expected, but they also show profound metabolic deficits that support a close association between circadian timing and feeding and metabolism. For example, the Clock gene mutant mouse is hyperglycaemic, hyperleptinaemic, and susceptible to obesity, displaying a phenotype similar to the human metabolic syndrome (Citation20). The Bmal1 mutant mouse also displays a phenotype with metabolic defects including impaired glucose homeostasis and reduced insulin secretion (Citation21). Accordingly, genetic evidence also strongly links circadian and metabolic functions in humans. For example, polymorphisms in the human gene, CLOCK, were associated with variation in circadian rhythms (Citation22), with obesity (Citation23), and even with the effectiveness of weight loss interventions (Citation24,Citation25). Racial differences in the circadian clock exist (Citation26,Citation27) and could affect individual susceptibility to circadian disruption, and contribute to ethnic variation in susceptibility to obesity in the urban environment (Citation28). Interaction between ethnic variation in clock function and the CD of urbanization could conceivably contribute to the increased susceptibility of some races to metabolic disease in modern environments. For example, in the Mapuches—an indigenous Native American population living in Chile—a 3-fold difference in insulin resistance has been observed between adults living traditional rural lifestyle and those living in cities, despite rural Mapuches having higher energy intakes and body mass indexes than their urban counterparts (Citation29). Studies of the interaction between the human genome and the prevalence of obesity in the urban environment will be informative in isolating and understanding an independent effect of CD on metabolic function.
How do disrupted rhythms affect metabolism?
Evidence from studies in rodents now conclusively supports a physiological effect of disrupted light–dark cycles on metabolic health. Initial studies in rats in the mid-2000s showed that repeated 12-h shifts of the light–dark cycle stimulated weight-gain (Citation30,Citation31). Convincing evidence to support the suspicion that CD might be an independent risk factor for obesity was later given by Karatsoreos et al. (Citation32), who showed that desynchronized mice showed increased weight-gain and decreased insulin sensitivity, even though their food intake did not differ from control animals. Rats subjected to repeated 3-h phase advances gained weight, showed disrupted glucose homeostasis and altered food preference, with increased consumption of simple carbohydrates (Citation33). Similar effects of CD have been shown in experimental studies in humans, where acute disruption of the light–dark cycle induced changes in glucose homeostasis consistent with reduced insulin sensitivity in healthy volunteers (Citation34,Citation35).
Mechanisms that underlie the effects of CD on metabolism include the following not mutually exclusive factors: disruption of central SCN function, desynchronization of metabolic and light-entrained circadian rhythms, direct effects on pancreatic function, sleep deprivation, and disruption of melatonin rhythms.
Disruption of SCN rhythmicity
Glucose homeostasis is subject to strong, endogenous circadian regulation (Citation36), and ablation of the central circadian pacemaker in the SCN initiated severe hepatic insulin resistance in mice (Citation37). In vivo electrophysiological recording revealed an immediate dampening of rhythm amplitude in the SCN of desynchronized mice that was associated with increased food intake and reduced energy expenditure (Citation38). Not surprisingly, these animals also gained weight, and showed a complete loss of circadian rhythms in insulin sensitivity determined using a euglycaemic clamp, and decreased energy expenditure. The animals subjected to CD rapidly lost SCN rhythm amplitude and gained weight even before a high-fat control group (Citation38). This finding demonstrated how rapidly metabolic control is lost following disruption of SCN function, albeit using a non-physiological intervention of constant light. These findings demonstrate a significant role of central circadian pacemaker function in regulating the effects of CD on glucose homeostasis, and predict that disruption of SCN function by CD, and by other factors such as ageing and neuropsychiatric disorders, will have deleterious metabolic consequences.
Desynchronization of metabolic and light-entrained circadian rhythms
Constant light severely affects rhythmicity of the central pacemaker, but other models of CD did not affect SCN function, although they still affected metabolism. For example, the light-sensitive oscillators in the brain of rats subjected to experimental shift-work remained entrained to the light–dark cycle, while rhythms in the centres that control feeding located in the dorsomedial and arcuate nucleus of the hypothalamus were disrupted (Citation39,Citation40). Just two weeks of CD was sufficient to induce profound disruption of the oscillation of clock and metabolic transcripts in the liver, and inhibited gluconeogenesis, but rhythms in the SCN still remained locked to the phase of the light–dark cycle (Citation41). These effects were ameliorated by confining feeding to the normal (circadian) active phase. However, enforcing the shifted rat's disrupted feeding pattern on control animals did not completely reproduce the metabolic effects, suggesting that desynchronization of feeding rhythms is a critical but not exclusive cause of the metabolic effects of CD. All of these data indicate that uncoupling of light- and food-entrained oscillators is a mechanism that underlies the effects of CD on glucose homeostasis. Accordingly, enforced day-time activity in mice had similar effects on circadian oscillators, with central pacemaker transcripts entrained to the photoperiod, while profound disruption was evident in circadian rhythms in clock gene expression and liver transcripts regulating metabolic pathways (Citation41). Thus, the metabolic effects of CD in rodents can be mediated through disruption of the synchrony between oscillators that regulate feeding with those entrained to the light–dark cycle (Citation40,Citation41).
Complex relationships exist between the effects of altered lighting, sleep deprivation, and CD; all of these factors contribute to loss of circadian rhythmicity and synchronicity between environmental, physiological, and behavioural factors. Furthermore, peripheral clocks can be subject to considerable disruption while central rhythmicity remains robust (Citation42). The relative significance of disrupted lighting, eating, and social zeitgebers for the metabolic effects of CD require further investigation in animal models, and in humans under controlled experimental conditions.
Remarkably, bright light was not required to disrupt the rhythms and metabolism of the laboratory mouse; these animals showed significant CD when the darkness of their nights was replaced with light as dim as twilight or candlelight (5 lux) (Citation43). At least in mice, the night-light intensity required to disrupt rhythmicity is far lower than typical electric lighting. Nevertheless, mice exposed to dim night-light showed increases in body weight that were significantly related to the proportion of their daily intake acquired in the light phase, metabolic effects that were prevented by restriction of feeding to the normal activity time (Citation39,Citation43). In concurrence with this, rodents fed only in the light phase gained weight (Citation44–46), and showed disrupted rhythmicity of metabolic gene expression in the liver, compared to animals fed in the dark phase (Citation44). Restricting feeding to the active phase also prevented weight-gain and typical metabolic changes (hyperinsulinemia, hepatic steatosis, and inflammation) induced by a high-fat diet in ad lib fed animals (Citation47). In rodents, eating during the inactive period seems to be an important mechanism in CD-mediated weight-gain. Further studies are required to establish if this finding translates to humans.
Inflammatory signalling
Inflammation is one of the hallmarks of the metabolic syndrome, and recent studies have shown that CD was associated with upregulation of inflammatory markers in humans (Citation1), and increased inflammatory responses in mice exposed to LPS (Citation2) or to a high fat diet (Citation3). There are strong bidirectional associations between the immune and circadian systems (Citation4) which support the contribution of neuroimmune mechanisms to the effects of CD on glucose homeostasis and obesity.
Leproult R, Holmb ck U, Van Cauter E. Circadian misalignment augments markers of insulin resistance and inflammation, independently of sleep loss. Diabetes. 2014; 63:1860–9.
Fonken LK, Weil ZM, Nelson RJ. Mice exposed to dim light at night exaggerate inflammatory responses to lipopolysaccharide. Brain Behav Immun. 2013a;34:159–63.
Fonken LK, Lieberman RA, Weil ZM, Nelson RJ. Dim light at night exaggerates weight gain and inflammation associated with a high-fat diet in male mice. Endocrinology. 2013b;154:3817–25.
Coogan AN, Wyse CA. Neuroimmunology of the circadian clock. Brain Res. 2008; 1232:104–12.
Direct effects of CD on pancreatic function
Exposure of rats to night-light disrupted the phase and synchrony of clock gene expression in pancreatic beta cells and diminished glucose-stimulated insulin secretion (Citation48), supporting that CD might directly affect beta cell function. Furthermore, CD accelerated the onset of diabetes in a transgenic rat model of type 2 diabetes mellitus by increasing the rate of beta cell apoptosis (Citation49), and pancreas-specific ablation of the circadian clock induced diabetes by blocking islet sensitivity to glucose and insulin (Citation21). These results suggest that CD directly affects pancreatic function.
Sleep deprivation
Separating the effects of CD from those of sleep deprivation can be problematic, and both interventions might independently affect metabolism. There is much evidence that the effects of CD are not dependent on sleep deprivation, even though human shift-workers may be subject to both. Studies in rodents have induced CD using photoperiod disruption protocols that do not curtail sleep duration (ultradian cycles, constant light), but still reproduce behavioural desynchrony and glucose intolerance (Citation38,Citation50). It is even more difficult to study the metabolic effects of sleep deprivation without disrupting circadian rhythms, but a rat model of partial sleep deprivation (ventrolateral preoptic area lesion) showed no signs of metabolic disruption despite 40% less sleep than controls (Citation51). Furthermore, mice with no effective circadian clock (Per1/2 mutants) were resistant to the metabolic effects of sleep deprivation, while just 5 days of sleep deprivation increased food intake, serum triglycerides, glucose, and leptin in wild-type controls (Citation52). A mistimed sleep–wake cycle induced a far greater disruption of the human peripheral transcriptome, compared to sleep restriction alone (Citation42), supporting independent effects of sleep timing and sleep deprivation. These data suggest that the obesogenic effects of sleep deprivation are primarily due to disruption of rhythmicity.
Melatonin
Melatonin is a pineal hormone whose secretion is suppressed by light, and which signals night-length, serving particularly as a timing cue for alignment of physiology with the seasons in photoperiodic animals. Pinealectomized rodents show glucose insensitivity (Citation53,Citation54), and reduced melatonin secretion was associated with increased risk of developing diabetes in human patients (Citation55). This supports an effect of melatonin secretion on glucose homeostasis, and disruption and suppression of melatonin by shortening and interruption of the darkness of night potentially contribute to the effects of CD on metabolism.
Significantly, mice with a genetic lesion of the circadian clock (Per1/2 mutants) were not affected by 5 days of CD, in contrast to their wild-type controls, that displayed hyperphagia and hyperleptinaemia (Citation52). Disruption of circadian rhythms by knock-out of another clock gene (Bmal1), or by constant light, also disrupted locomotor rhythmicity and predisposed animals to insulin resistance and obesity, an effect that was rescued by transgenic expression of Bmal1 (Citation56). These findings that clock gene mutant mice were protected from deleterious metabolic effects strongly support a primary involvement of circadian clock gene mechanisms in the metabolic effects of CD.
Despite rigorous experimental controls, studies of CD in humans are confounded by genetic, behavioural, lifestyle, and other factors that are unavoidably variable between individuals. Animal studies allow many of these factors to be controlled but cannot reproduce the human photoperiodic response, since most rodents are nocturnal, with a behavioural response and sensitivity to the intensity and wavelength of light that differs considerably from that of humans. Indeed, several studies have failed to reproduce the metabolic effects of CD in rodents; reversal of the light–dark cycle every 5 days did not affect body weight of mice (Citation57), and 10 weeks of bi-weekly phase advance did not affect metabolism in control rats, although it did accelerate the development of diabetes in rats with a genetic predisposition to beta cell failure (Citation49). A recent study of shift-work in rats was unable to reproduce detrimental effects on metabolism (Citation58), and in one older study lower body weights were reported after a lifetime of weekly 12-h phase shifts in the mouse (Citation59). The relationship between rhythmicity and metabolism is a complex one, and the possibility that CD might exacerbate rather than cause metabolic dysfunction is not yet fully excluded.
Nevertheless, the experimental induction and reversal of obesity and metabolic dysfunction in animals exposed to CD, as well as increased metabolic disease in human shift-workers, and absence of CD-induced metabolic effects in clock gene mutant animals, generate a collective body of evidence that implicates CD as a primary factor in the pathophysiology of human obesity.
The metabolic effects of circadian desynchrony on humans
The disrupted lifestyle of the shift-worker is a model of human CD, and a large body of evidence now implicates shift-work as an independent risk factor for metabolic disease, weight-gain, and obesity (Citation33,Citation41,Citation60,Citation61). The risks of shift-work have long been recognized, and in the early days of artificial light, night-work was generally considered to be immoral and antisocial because of its disruptive effect on family life. In fact, early-twentieth-century legislation banned shift-work for women and children (Berne Convention 1906, 1919, and 1926) (Citation62), regulations later repealed on grounds of discrimination against women. Interestingly, recent evidence does suggest that women are more susceptible to the adverse effects of shift-work than men (Citation63).
Despite diligent correction for known risk factors, the complex nature of human demography means that a confounding covariable can never be completely excluded in studies of weight-gain in human shift-workers. Nevertheless, the fact that shift-work has been associated with metabolic disease in workers across the globe, in diverse occupations, and of different genetic and socio-economic backgrounds (Citation7,Citation60,Citation61,Citation64–66) supports that the metabolic effects of shift-work are independent of work-related factors. A direct, causal relationship is further suggested by the fact that the duration of shift-work was positively and significantly related to weight-gain in several studies (Citation7,Citation61). The population implications of such findings are significant, since at least 20% of UK workers are required to undertake shift-work, and regulation of their working hours would have serious social and economic consequences. The economic and legal impact of compensation of shift-workers for disorders related to their work schedules may also be a serious concern for the employers of the future.
Appetite and eating behaviour are strongly regulated by circadian mechanisms (Citation67), generating a cycle of hunger that is lowest in the morning and increases during daylight hours. Disruption of clock mechanisms by shift-work might affect regulation of daily patterns of eating behaviour and appetite, predisposing to overeating and obesity. On the other hand, regular, rhythmic eating behaviour with consistent meal-times might entrain and amplify circadian rhythms in appetite and eating behaviour, facilitating metabolic health. By definition, shift-work affects the timing and pattern of food intake, but most studies report that total food intake is not increased in shift-workers (Citation68–70). Some studies reported that shift-workers show changes in eating behaviour including reduced meal frequency and increased intake of high-energy snacks (Citation68), which corroborates changes in food preference reported in some studies of desynchronized rodents (Citation33). It remains unclear if the metabolic sequelae of shift-work are mediated directly through circadian timing mechanisms or if they occur secondary to changes in eating behaviour. But there are no consistent effects of CD or shift-work on eating behaviour in humans or in animals, with studies reporting increased, decreased, or no effect. This suggests that the metabolic effects of shift-work are not mediated by direct effects on eating behaviour, but more likely relate to more complex effects of altered timing of food intake relative to endogenous cycles.
Shift-workers have been widely studied as examples of desynchronized humans, but they may represent an extreme example of a chronic desynchrony that is a consequence of modern lifestyle. There is a deficiency of studies of the relationship between CD and metabolism in people not engaged in shift-work. A recent study demonstrated that the degree of exposure to artificial night-light in a controlled home setting was significantly related to BMI and serum triglycerides (Citation71), an interesting finding that corroborates the data from shift-workers. The relationship between CD and metabolic health may be a vicious cycle with CD promoting obesity, which itself causes fragmented sleep patterns and disrupted metabolic rhythms (Citation72). In fact, disrupted rhythmicity preceded the onset of obesity in the leptin-deficient ob/ob mouse (Citation73), and was detected prior to disruption of glucose and insulin response in pharmacological models of diabetes (Citation74). These findings suggest that disrupted rhythmicity is a very early event in the pathophysiology of metabolic dysfunction, which is suggestive of a primary aetiological role. Obesity may be a consequence of a primary metabolic disruption induced by disruption of rhythmicity.
Are rhythmic animals and humans thinner?
If disruption of circadian rhythmicity induces metabolic dysfunction and obesity, then might the corollary also be true, that strong rhythms are protective? There are many models of CD, but few of the reverse condition, of increased rhythmicity. The only genetic animal model of increased rhythmicity is the αMUPA mouse, a transgenic animal that overexpresses a protease involved in brain plasticity, and that exhibits high-amplitude circadian rhythmicity in behaviour and clock gene expression (Citation75). Intriguingly, these rhythmic animals have a low body weight, spontaneously reduced feed intake, and increased lifespan compared to the wild type (Citation75). Exercise is an intervention that amplifies rhythmicity in animals (Citation76) and in humans (Citation77), with well-established metabolic benefits (Citation78); whether changes in rhythmicity contribute to the metabolic benefits of exercise remains to be determined.
Studies of the relation between increased rhythmicity and metabolic health in humans are greatly complicated by multiple diet- and lifestyle-related confounding factors. Nevertheless, it is interesting to consider the metabolic health of subgroups within the global population that have resisted the Western lifestyle and retained pre-electric light circadian rhythms.
The Amish community of Pennsylvania is a Christian group that has actively resisted adoption of a Western lifestyle. A reluctance to use electricity has isolated this community from the CD that is engendered by artificial light. But surprisingly, the BMI of the adult Amish person was not significantly different from the average US individual (Citation79). In spite of this, their rate of diabetes remains low, suggesting that different mechanisms underlie the relationship between BMI and diabetes in the Amish people. Similarly, the native peoples of the North, the Sami and the Inuit, and the Mapuche Indians of Chile, who also maintain agrarian lifestyles, show a capacity to maintain metabolic health at a BMI that might be associated with metabolic dysfunction in other ethnic groups (Citation29,Citation80–82). However, the manual work of these people makes it impossible to establish if their metabolic health is simply due to increased physical activity or if there is an addition effect of the rhythmicity of their lifestyle. In support of the latter, a group of relatively sedentary, urbanization-resisting people—Italian nuns in an enclosed religious order—showed no lifetime increase in blood pressure, in contrast with a control group of never-married women (), despite BMI increasing at a similar rate in both groups over the 20-year observation period and the nuns having a higher overall BMI () (Citation83).
Figure 7. A cohort of Italian nuns in an enclosed order appeared to be protected from typical age-related increases in diastolic and systolic blood pressure of age-matched, unmarried control women (B). This is despite higher values of BMI in the nuns (A). Redrawn from.
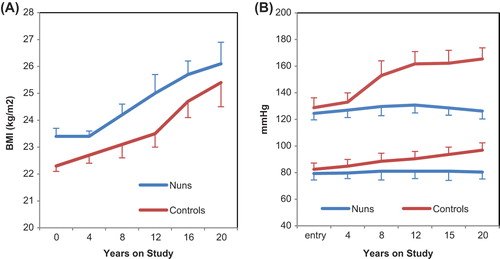
These humans that live in modern times, but maintain rhythmic lifestyles with reduced use of electric light and appliances, seem to share an altered relationship between BMI and metabolic dysfunction. These effects cannot be attributed purely to reduced exposure to electric light, or even to increased circadian rhythmicity, since there also exist striking differences in genotype, exercise, diet, stress, and other lifestyle-associated factors. Nevertheless, the increased metabolic health in these groups is intriguing and at least supports an association between a rhythmic life and metabolic health. There is of course, substantial evidence to support the reverse argument, that loss of rhythmicity causes metabolic dysfunction.
Migration from rural to urban environments is a well- established instigator of metabolic disruption and obesity (Citation84–86). For example, members of the Gbagi tribe that lived in an urban centre in Nigeria had increased BMI and fasting blood glucose compared to genetically similar, age-matched controls that retained a village life. Changes in physical activity were unlikely fully to account for the effects of urbanization on the Gbagi, since the urban group worked as labourers or construction workers (Citation85). Studies of 1726 rural and urban Cameroonians showed that exposure to an urban environment was significantly associated with BMI, an effect that was independent of physical activity and socio-professional category (Citation86). Unfortunately, neither of these studies comparing rural and urban Africans assessed dietary factors. Indeed, it is difficult to establish the relative importance of complex and interrelated diet, physical activity, and lifestyle factors that contribute to the effects of urbanization on metabolism. However, studies of global trends in the association between cardiovascular risk factors and Westernization have reported weakening of the relationship between BMI and national income or Western diet, although global BMI remains strongly associated with urbanization (Citation87). This finding led the authors to suggest that the effects of urbanization on BMI may be mediated by lifestyle factors additional to those of the Western diet (Citation87). Loss of the rhythmicity and synchronicity of human sleep, work, exercise, and eating patterns is one feature of urban lifestyle that might be of unsuspected significance in the global obesity epidemic. The human clock shows a sensitivity to light that is highly variable between individuals, but is also dependent on previous light exposure (Citation88). This suggests that dim day-time and disrupted night-time light exposure might directly affect the sensitivity of the clock to entrainment, further exacerbating the effects of the weak amplitude environmental rhythms of the urban environment. Genetic variation in clock function could interact with these environmental effects to regulate susceptibility to CD and its metabolic effects. This mechanism might contribute to individual and ethnic variations in susceptibility to metabolic disease associated with CD and urbanization.
To summarize
Artificial light has significantly impacted human circadian rhythms by: 1) permitting desynchronization of circadian rhythms in activity, sleep, social interactions, and food intake; 2) dampening the photic zeitgeber by instigating bright nights and dim days; and 3) eliminating seasonal changes in circadian rhythms.
These changes are likely to have implications for many aspects of physiology and behaviour. Adaptation of metabolism to this modern photoperiod appears to be particularly problematic, and photoperiodic disruption is associated with insulin resistance and predisposition to obesity in man and in animals. These metabolic changes occur through desynchronization of central and peripheral oscillators, disruption of central clock mechanisms, and through direct effects on pancreatic function. Secondary effects of changes in eating behaviour and food preference require further investigation, especially in humans. The obesity of urbanization might be a consequence of metabolic dysfunction induced by CD.
Future directions—where will the night-light take us next?
The evidence reviewed here support an association between artificial night-light and metabolic dysfunction, but a causal relationship is not yet established. Studies in desynchronized laboratory animals may not translate to human, or even to wild animal physiology. Numerous covariables complicate the interpretation of epidemiological data from shift-workers, and it should be considered that shift-work is not exclusive to the modern age; sailors, shepherds, and soldiers have been so engaged for centuries, without evidence of diabetes and obesity. The human lifestyle of recent decades is somehow responsible for an unprecedented rise of metabolic disease. Artificial light, and the loss of rhythmicity it generates, is one facet of this, and its relative importance is the focus of continued study.
Mechanistic data from animal models in addition to circumstantial evidence from humans support a role for disrupted rhythmicity and artificial light on metabolic health. This evidence will fuel future efforts to regulate exposure to light at night, increase day-time light, and increase consideration of clock function in the maintenance of human health. Rather than curtail artificial light, research will inform how rhythmicity might instead be managed in parallel to our manipulation of the photoperiod. Future studies of metabolic health will require that two further dimensions to human physiology and behaviour are considered: those of rhythmicity and synchronicity.
Declaration of interest: The authors report no conflicts of interest.
References
- Ekirch AR. At day's close. London: Orion Books; 2005.
- Wehr TA, Moul DE, Barbato G, Giesen HA, Seidel JA, Barker C, et al. Conservation of photoperiod-responsive mechanisms in humans. Am J Physiol. 1993;265(4 Pt 2):R846–57.
- Wehr TA. In short photoperiods, human sleep is biphasic. J Sleep Res. 1992;1:103–7.
- Pittendrigh CSDS. A functional analysis of circadian pacemakers in nocturnal rodents IV. Entrainment: Pacemaker as clock. Journal of Comparative Physiology. 1976;106:291.
- Arnulf I, Brion A, Pottier M, Golmard JL. Ring the bell for matins: circadian adaptation to split sleep by cloistered monks and nuns. Chronobiol Int. 2011;28:930–41.
- Wright KP, McHill AW, Birks BR, Griffin BR, Rusterholz T, Chinoy ED. Entrainment of the human circadian clock to the natural light-dark cycle. Curr Biol. 2013;23:1554–8.
- Pan A, Schernhammer ES, Sun Q, Hu FB. Rotating night shift work and risk of type 2 diabetes: two prospective cohort studies in women. PLoS Med. 2011;8:e1001141.
- Grundy A, Richardson H, Burstyn I, Lohrisch C, SenGupta SK, Lai AS, et al. Increased risk of breast cancer associated with long-term shift work in Canada. Occup Environ Med. 2013;70:831–8.
- Flynn-Evans EE, Mucci L, Stevens RG, Lockley SW. Shiftwork and prostate-specific antigen in the National Health and Nutrition Examination Survey. J Natl Cancer Inst. 2013;105:1292–7.
- World Health Organization. Obesity and Overweight. World Health Organisation Fact Sheet No. 311, March 2013. http://www.who.int/mediacentre/factsheets/fs311/en/
- Report F. Tackling obesities: future choices-project report. UK Government Stationery Office, 2007. http://www.foresight.gov.uk/Obesity/obesity_final/Index.html
- Knight I, Manning M, Swinton M, Ribberink H. European and Canadian non-HVAC Electric and DHW load profiles for use in simulating the performance of residential cogeneration systems. UK: Cardiff, Cardiff University; 2007.
- Yang C-M, Spielman AJ, D’Ambrosio P, Serizawa S, Nunes J, Birnbaum J. A single dose of melatonin prevents the phase delay associated with a delayed weekend sleep pattern. Sleep. 2001;24:272–81.
- Buhr ED, Takahashi JS. Molecular components of the mammalian circadian clock. Handb Exp Pharmacol. 2013;(217):3–27.
- Storch KF, Lipan O, Leykin I, Viswanathan N, Davis FC, Wong WH, et al. Extensive and divergent circadian gene expression in liver and heart. Nature. 2002;417:78.
- Freedman MS, Lucas RJ, Soni B, von Schantz M, Muñoz M, David-Gray Z, et al. Regulation of mammalian circadian behavior by non-rod, non-cone, ocular photoreceptors. Science. 1999;284:502–4.
- Roenneberg T, Allebrandt KV, Merrow M, Vetter C. Social jetlag and obesity. Curr Biol. 2012;22:939–43.
- Merikanto I, Lahti T, Puolijoki H, Vanhala M, Peltonen M, Laatikainen T, et al. Associations of chronotype and sleep with cardiovascular diseases and type 2 diabetes. Chronobiol Int. 2013; 30:470–7.
- Lucassen EA, Zhao X, Rother KI, Mattingly MS, Courville AB, de Jonge L, et al. Evening chronotype is associated with changes in eating behavior, more sleep apnea, and increased stress hormones in short sleeping obese individuals. PLoS One. 2013;8:e56519.
- Turek FW, Joshu C, Kohsaka A, Lin E, Ivanova G, McDearmon E, et al. Obesity and metabolic syndrome in circadian Clock mutant mice. Science. 2005;308:1043.
- Marcheva B, Ramsey KM, Buhr ED, Kobayashi Y, Su H, Ko CH, et al. Disruption of the clock components CLOCK and BMAL1 leads to hypoinsulinaemia and diabetes. Nature. 2010;466:627.
- Bandín C, Martinez-Nicolas A, Ordovás JM, Ros Lucas JA, Castell P, Silvente T, et al. Differences in circadian rhythmicity in CLOCK 3111T/C genetic variants in moderate obese women as assessed by thermometry, actimetry and body position. Int J Obes (Lond). 2013;37:1044–50.
- Sookoian S, Gemma C, Scott EM, Carter AM, Grant PJ. Genetic variants of Clock transcription factor are associated with individual susceptibility to obesity; association between polymorphisms in the Clock gene, obesity and the metabolic syndrome in man. Int J Obes (Lond). 2008;87; 32(6; 4):1606; 658.
- Sookoian S, Gemma C, Gianotti TF Burgueño A, Castaño G, Pirola CJ. Genetic variants of Clock transcription factor are associated with individual susceptibility to obesity. Am J Clin Nutr. 2008;87: 1606–15.
- Garaulet M, Corbalan-Tutau MD, Madrid JA, Baraza JC, Parnell LD, Lee YC, et al. PERIOD2 variants are associated with abdominal obesity, psycho-behavioral factors, and attrition in the dietary treatment of obesity. J Am Diet Assoc. 2010;110:917–21.
- Smith MR, Burgess HJ, Fogg LF, Eastman CI. Racial differences in the human endogenous circadian period. PloS One. 2009;4:e6014.
- Eastman CI, Molina TA, Dziepak ME, Smith MR. Blacks (African Americans) have shorter free-running circadian periods than whites (Caucasian Americans). Chronobiol Int. 2012;29:1072–7.
- Luke A, Guo X, Adeyemo AA, Wilks R, Forrester T, Lowe W Jr, et al. Heritability of obesity-related traits among Nigerians, Jamaicans and US black people. Int J Obes Relat Metab Disord. 2001;25:1034–41.
- Celis-Morales CA, Perez-Bravo F, Ibañes L, Sanzana R, Hormazabal E, Ulloa N, et al. Insulin resistance in Chileans of European and indigenous descent: evidence for an ethnicity x environment interaction. PLoS One. 2011;6:e24690.
- Tsai LL, Tsai YC, Hwang K, Huang YW, Tzeng JE. Repeated light-dark shifts speed up body weight gain in male F344 rats. Am J Physiol Endocrinol Metab. 2005;289:E212–17.
- Tsai LL, Tsai YC. The effect of scheduled forced wheel activity on body weight in male F344 rats undergoing chronic circadian desynchronization. Int J Obes (Lond). 2007;31:1368–77.
- Karatsoreos IN, Bhagat S, Bloss EB, Morrison JH, McEwen BS. Disruption of circadian clocks has ramifications for metabolism, brain, and behavior. Proc Natl Acad Sci U S A. 2011;108:1657.
- McDonald RJ, Zelinski EL, Keeley RJ, Sutherland D, Fehr L, Hong NS. Multiple effects of circadian dysfunction induced by photoperiod shifts: alterations in context memory and food metabolism in the same subjects. Physiol Behav. 2013;118:14–24.
- Scheer FA, Hilton MF, Mantzoros CS, Shea SA. Adverse metabolic and cardiovascular consequences of circadian misalignment. Proc Natl Acad Sci U S A. 2009;106:4453–8.
- Buxton OM, Cain SW, O’Connor SP, Porter JH, Duffy JF, Wang W, et al. Adverse metabolic consequences in humans of prolonged sleep restriction combined with circadian disruption. Sci Translat Med. 2012;4:129ra43.
- la Fleur SE, Kalsbeek A, Wortel J, Fekkes ML, Buijs RM. A daily rhythm in glucose tolerance: a role for the suprachiasmatic nucleus. Diabetes. 2001;50:1237–43.
- Coomans CP, van den Berg SA, Lucassen EA, Houben T, Pronk AC, van der Spek RD, et al. The suprachiasmatic nucleus controls circadian energy metabolism and hepatic insulin sensitivity. Diabetes. 2013;62:1102.
- Coomans CP, van den Berg SA, Houben T, van Klinken JB, van den Berg R, Pronk AC, et al. Detrimental effects of constant light exposure and high-fat diet on circadian energy metabolism and insulin sensitivity. FASEB J. 2013;27:1721–32.
- Salgado-Delgado R, Angeles-Castellanos M, Saderi N, Buijs RM, Escobar C. Food intake during the normal activity phase prevents obesity and circadian desynchrony in a rat model of night work. Endocrinology. 2010;151:1019–29.
- Salgado-Delgado R, Nadia S, Angeles-Castellanos M, Buijs RM, Escobar C. In a rat model of night work, activity during the normal resting phase produces desynchrony in the hypothalamus. J Biol Rhythms. 2010;25:421–31.
- Barclay JL, Husse J, Bode B, Naujokat N, Meyer-Kovac J, Schmid SM, et al. Circadian desynchrony promotes metabolic disruption in a mouse model of shiftwork. PloS One. 2012;7:e37150.
- Archer SN, Laing EE, Möller-Levet CS, van der Veen DR, Bucca G, Lazar AS, et al. From the cover: mistimed sleep disrupts circadian regulation of the human transcriptome. Proc Natl Acad Sci U S A. 2014;111:E682–91.
- Fonken LK, Workman JL, Walton JC, Weil ZM, Morris JS, Haim A, et al. Light at night increases body mass by shifting the time of food intake. Proc Natl Acad Sci U S A. 2010;107:18664.
- Bray MS, Ratcliffe WF, Grenett MH, Brewer RA, Gamble KL, Young ME. Quantitative analysis of light-phase restricted feeding reveals metabolic dyssynchrony in mice. Int J Obes (Lond). 2013;37:843–52.
- Arble DM, Bass J, Laposky AD, Vitaterna MH, Turek FW. Circadian timing of food intake contributes to weight gain. Obesity (Silver Spring). 2009;17:2100–2.
- Reznick J, Preston E, Wilks DL, Beale SM, Turner N, Cooney GJ. Altered feeding differentially regulates circadian rhythms and energy metabolism in liver and muscle of rats. Biochim Biophys Acta. 2013;1832:228–38.
- Hatori M, Vollmers C, Zarrinpar A, DiTacchio L, Bushong EA, Gill S, et al. Time-restricted feeding without reducing caloric intake prevents metabolic diseases in mice fed a high-fat diet. Cell Metab. 2012;15:848–60.
- Qian J, Block GD, Colwell CS, Matveyenko AV. Consequences of exposure to light at night on the pancreatic islet circadian clock and function in rats. Diabetes. 2013;62:3469–78.
- Gale JE, Cox HI, Qian J, Block GD, Colwell CS, Matveyenko AV. Disruption of circadian rhythms accelerates development of diabetes through pancreatic beta-cell loss and dysfunction. J Biol Rhythms. 2011;26:423–33.
- Oishi K. Disrupted light-dark cycle induces obesity with hyperglycemia in genetically intact animals. Neuro Endocrinol Lett. 2009;30:458–61.
- Vetrivelan R, Fuller PM, Yokota S, Lu J, Saper CB. Metabolic effects of chronic sleep restriction in rats. Sleep. 2012;35:1511–20.
- Husse J, Hintze SC, Eichele G, Lehnert H, Oster H. Circadian clock genes Per1 and Per2 regulate the response of metabolism-associated transcripts to sleep disruption. PLoS One. 2012;7:e52983.
- Lima FR, Gervais A, Colin C, Izembart M, Neto VM, Mallat M. Regulation of microglial development: a novel role for thyroid hormone. J Neurosci. 2001;21:2028–38.
- Lima FB, Machado UF, Bartol I, Seraphim PM, Sumida DH, Moraes SM, et al. Pinealectomy causes glucose intolerance and decreases adipose cell responsiveness to insulin in rats. Am J Physiol. 1998;275(6 Pt 1):E934–41.
- Radziuk J, Pye S. Diurnal rhythm in endogenous glucose production is a major contributor to fasting hyperglycaemia in type 2 diabetes. Suprachiasmatic deficit or limit cycle behaviour? Diabetologia. 2006;49:1619–28.
- Shi SQ, Ansari TS, McGuinness OP, Wasserman DH, Johnson CH. Circadian disruption leads to insulin resistance and obesity. Curr Biol. 2013;23:372–81.
- Preuss F, Tang Y, Laposky AD, Arble D, Keshavarzian A, Turek FW. Adverse effects of chronic circadian desynchronization in animals in a “challenging” environment. Am J Physiol Regul Integr Comp Physiol. 2008;295:R2034–40.
- Leenaars CH, Kalsbeek A, Hanegraaf MA, Foppen E, Joosten RN, Post G, et al. Unaltered instrumental learning and attenuated body-weight gain in rats during non-rotating simulated shiftwork. Chronobiol Int. 2012;29:344–55.
- Nelson W, Halberg F. Schedule-shifts, circadian rhythms and lifespan of freely-feeding and meal-fed mice. Physiol Behav. 1986;38:781–8.
- Tucker P, Marquie JC, Folkard S, Ansiau D, Esquirol Y. Shiftwork and metabolic dysfunction. Chronobiol Int. 2012;29:549–55.
- van Amelsvoort LG, Schouten EG, Kok FJ. Duration of shiftwork related to body mass index and waist to hip ratio. Int J Obes Relat Metab Disord. 1999;23:973–8.
- 1906 BC. International convention respecting the prohibition of night work for women in industrial employment. Am J Int Law (Supplement: Official Documents). 1910;4:328–37.
- Karlsson B, Knutsson A, Lindahl B. Is there an association between shift work and having a metabolic syndrome?Results from a population based study of 27,485 people. Occup Environ Med. 2001;58:747–52.
- Suwazono Y, Dochi M, Oishi M, Tanaka K, Kobayashi E, Sakata K. Shiftwork and impaired glucose metabolism: a 14-year cohort study on 7104 male workers. Chronobiol Int. 2009;26:926–41.
- Parkes KR. Shift work and age as interactive predictors of body mass index among offshore workers. Scand J Work Environ Health. 2002;28:64–71.
- Martinez JA, Kearney JM, Kafatos A, Paquet S, Martinez-Gonzalez MA. Variables independently associated with self-reported obesity in the European Union. Public Health Nutr. 1999;2(1A):125–33.
- Scheer FA, Morris CJ, Shea SA. The internal circadian clock increases hunger and appetite in the evening independent of food intake and other behaviors. Obesity (Silver Spring). 2013;21:421–3.
- Atkinson G, Fullick S, Grindey C, Maclaren D. Exercise, energy balance and the shift worker. Sports Med. 2008;38:671–85.
- Lennernas M, Akerstedt T, Hambraeus L. Nocturnal eating and serum cholesterol of three-shift workers. Scand J Work Environ Health. 1994;20:401–6.
- de Assis MA, Nahas MV, Bellisle F, Kupek E. Meals, snacks and food choices in Brazilian shift workers with high energy expenditure. J Hum Nutr Diet. 2003;16:283–9.
- Obayashi K, Saeki K, Iwamoto J, Okamoto N, Tomioka K, Nezu S, et al. Exposure to light at night, nocturnal urinary melatonin excretion, and obesity/dyslipidemia in the elderly: a cross-sectional analysis of the HEIJO-KYO Study. J Clin Endocrinol Metab. 2013;98:337–44.
- Prasai MJ, Mughal RS, Wheatcroft SB, Kearney MT, Grant PJ, Scott EM. Diurnal variation in vascular and metabolic function in diet-induced obesity: divergence of insulin resistance and loss of clock rhythm. Diabetes. 2013;62:1981–9.
- Ando H, Kumazaki M, Motosugi Y, Ushijima K, Maekawa T, Ishikawa E, et al. Impairment of peripheral circadian clocks precedes metabolic abnormalities in ob/ob mice. Endocrinology. 2011;152:1347.
- Yang SC, Tseng HL, Shieh KR. Circadian-clock system in mouse liver affected by insulin resistance. Chronobiol Int. 2013;30:796–810.
- Froy O, Chapnik N, Miskin R. Long-lived alphaMUPA transgenic mice exhibit pronounced circadian rhythms. Am J Physiol Endocrinol Metab. 2006;291:E1017–24.
- Leise TL, Harrington ME, Molyneux PC, Song I, Queenan H, Zimmerman E, et al. Voluntary exercise can strengthen the circadian system in aged mice. Age (Dordr). 2013;35:2137–52.
- Van Someren EJ, Lijzenga C, Mirmiran M, Swaab DF. Long-term fitness training improves the circadian rest-activity rhythm in healthy elderly males. J Biol Rhythms. 1997;12:146–56.
- Whyte LJ, Gill JM, Cathcart AJ. Effect of 2 weeks of sprint interval training on health-related outcomes in sedentary overweight/obese men. Metabolism. 2010;59:1421–8.
- Hairston KG, Ducharme JL, Treuth MS, Hsueh WC, Jastreboff AM, Ryan KA, et al. Comparison of BMI and physical activity between old order Amish children and non-Amish children. Diabetes Care. 2013;36:873–8.
- Young TK, Bjerregaard P, Dewailly E, Risica PM, Jorgensen ME, Ebbesson SE. Prevalence of obesity and its metabolic correlates among the circumpolar inuit in 3 countries. Am J Public Health. 2007;97:691–5.
- Bjerregaard P, Jorgensen ME, Andersen S, Mulvad G, Borch-Johnsen K; Greenland Population Study. Decreasing overweight and central fat patterning with Westernization among the Inuit in Greenland and Inuit migrants. Int J Obes Relat Metab Disord. 2002;26:1503–10.
- Kellett S, Poirier P, Dewailly E, Sampasa H, Chateau-Degat ML. Is severe obesity a cardiovascular health concern in the Inuit population?Am J Hum Biol. 2012;24:441–5.
- Timio M, Verdecchia P, Venanzi S, Gentili S, Ronconi M, Francucci B, et al. Age and blood pressure changes. A 20-year follow-up study in nuns in a secluded order. Hypertension. 1988;12:457.
- Shivpuri S, Allison MA, Macera CA, Lindsay S, Gallo LC. Associations between nocturnal blood pressure dipping and the metabolic syndrome in high- vs. low-acculturated Mexican American women. Am J Hypertens. 2013;26:1030–6.
- Adediran OS, Adebayo PB, Akintunde AA. Anthropometric differences among natives of Abuja living in urban and rural communities: correlations with other cardiovascular risk factors. BMC Res Notes. 2013;6:123.
- Sobngwi E, Mbanya JC, Unwin NC, Porcher R, Kengne AP, Fezeu L, et al. Exposure over the life course to an urban environment and its relation with obesity, diabetes, and hypertension in rural and urban Cameroon. Int J Epidemiol. 2004;33:769–76.
- Danaei G, Singh GM, Paciorek CJ, Lin JK, Cowan MJ, Finucane MM, et al. The global cardiovascular risk transition: associations of four metabolic risk factors with national income, urbanization, and Western diet in 1980 and2008. Circulation.2013;127:1493–502, 502e1–8.
- Hébert M, Martin SK, Lee C, Eastman CI. The effects of prior light history on the suppression of melatonin by light in humans. J Pineal Res. 2002;33:198–203.
- Fouquet R. Seven centuries of energy services: the price and use of light in the United Kingdom (1300–2000). The Energy Journal. 2006;27:139–177.