Abstract
Different cells of adipose tissue secrete compounds which regulate various biological processes. Changes in body weight, body composition, and amount of fat mass can alter the secretory profile and function of adipose tissue. Comparison of adipose tissue mRNA expression profiles before versus after weight loss or between obese and lean subjects has promoted the identification of novel adipokines. Weight loss decreases the expression of the tenomodulin (TNMD) mRNA in the adipose tissue, and the expression level is strongly correlated with body mass index. TNMD (locus Xq22) is expressed in both adipocyte and stromal vascular fraction of adipose tissue. Tenomodulin inhibits angiogenesis, but its specific function in adipose tissue is still unknown. We have reported modest association between TNMD sequence variation and different obesity-related phenotypes, including anthropometric measurements, inflammation, glucose and lipid metabolism, and age-related macular degeneration. In this review, the potential mechanisms that could link TNMD with the pathogenesis of obesity and related disorders are discussed.
Key words::
Abbreviations | ||
2h-PG | = | 2-hour plasma glucose level in an oral glucose tolerance test |
AD | = | Alzheimer's disease |
AMD | = | age-related macular degeneration |
APOE | = | apolipoprotein E |
AT | = | adipose tissue |
BMI | = | body mass index |
CCL | = | chemokine (C-C motif) ligand |
CHM | = | chondromodulin |
CI | = | confidence interval |
DPS | = | the Finnish Diabetes Prevention Study |
ER | = | endoplasmic reticulum |
FPG | = | fasting plasma glucose level in an oral glucose tolerance test |
MetS | = | metabolic syndrome |
METSIM | = | the Metabolic Syndrome in Men Study |
MSTN | = | myostatin |
T2D | = | type 2 diabetes |
TNMD | = | tenomodulin |
Key messages
Tenomodulin is an angiogenesis inhibitor, which has recently been shown to be expressed in adipose tissue. The expression levels are different between obese and nonobese, and the expression is down-regulated by weight loss.
Altered angiogenesis has been linked with obesity and related phenotypes.
The single nucleotide polymorphisms in the tenomodulin gene have been associated with obesity and obesity-associated phenotypes, although these findings have not yet been replicated.
Introduction
The role of adipose tissue (AT) as one of the major endocrine organs is well established (Citation1). Different cell types of AT secrete compounds which regulate various processes, including inflammation (Citation1,Citation2), vascular function (Citation2,Citation3), lipid and glucose metabolism (Citation1,Citation4), and extracellular matrix remodelling and angiogenesis (Citation1,Citation5,Citation6). Alterations in body weight (Citation1,Citation7), body composition, and amount of fat mass (Citation8) have been shown to alter the secretory profile and function of AT.
Comparison of adipose tissue mRNA expression profiles between obese and lean subjects has helped in the identification of novel adipokines (Citation9,Citation10). Furthermore, studies comparing gene expression before and after the weight reduction in AT can be useful in recognizing genes involved in the development of obesity, metabolic syndrome (MetS), or type 2 diabetes (T2D). We (Citation11) and others (Citation12,Citation13) have demonstrated that weight loss decreases the expression of the tenomodulin (TNMD) mRNA in adipose tissue. The mRNA levels of TNMD correlated strongly with body mass index (BMI) (Citation11,Citation13), insulin sensitivity, leptin levels, and both fat and lean body mass (Citation11). We have also reported associations between TNMD sequence variation and different obesity-related phenotypes (Citation14–18). In this review the potential role of TNMD in the pathogenesis of obesity-related disorders is discussed.
Structure and function of the tenomodulin gene and protein
Tenomodulin was identified in 2001 by Cros et al. (Citation19), who demonstrated that a novel gene, which they named myodulin, was 2-fold down-regulated in muscle atrophy in mice. Simultaneously, other groups cloned the same gene and named it chondromodulin-I-like (Citation20), tenomodulin (Citation21), and tendin (Citation22). The tenomodulin gene spans approximately 15 kb in chromosomal locus Xq22. Alternative splicing is known to occur, but the functions and tissue distribution of alternative transcripts have not yet been characterized. The isoform that contains seven exons is the most common splice variant (Citation23).
TNMD belongs to the BRICHOS protein family (Citation24). Similarly to the other members of this family, TNMD is an integral type 2 transmembrane protein with cytoplasmic N-terminal and extracellular C-terminal domains () (Citation24,Citation25). The extracellular part of the TNMD protein is composed of two domains: BRICHOS domain, which has been suggested to function as an intramolecular chaperone for the cleaved part (Citation24,Citation26), and a cysteine-rich C-terminal anti-angiogenic domain, which is cleaved proteolytically (Citation25,Citation27).
Figure 1. The organization of human tenomodulin gene and protein. Different domains (transmembrane, BRICHOS, and the antiangiogenic domain) are indicated with grey shading. The functional RXXR cleavage site is denoted with an arrow. The locations of the markers used in the different genetic association studies are included. (AD = Alzheimer's disease study; AMD = age-related macular degeneration study; DPS = the Finnish Diabetes Prevention Study; METSIM = the Metabolic Syndrome in Men Study).
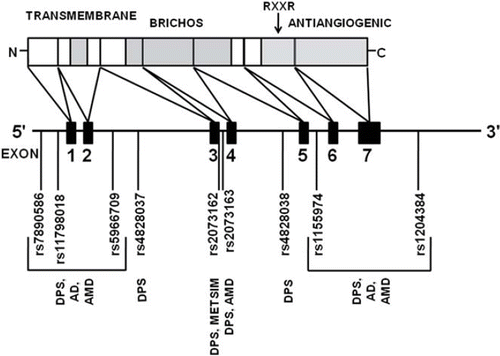
TNMD does not have any close homologues, but it exhibits overall 33% amino acid sequence identity with chondromodulin (CHM), which is a chondrocyte growth factor (Citation28) and angiogenesis inhibitor (Citation29). The similarities in the structural organization between these two proteins are apparent. The sequence similarity of the C-terminal is quite high (65%), and the cysteine residues that are needed for the correct folding are identically spaced in both CHM and TNMD (Citation25,Citation28,Citation29). However, the molecular weight the of secreted part is different. The secreted part of TNMD is only 16 kDa (Citation25), while that of CHM is 25 kDa (Citation28,Citation29). The exact targets of these domains are unknown.
Functional studies performed in vitro have shown that the secreted parts of both CHM and TNMD inhibit angiogenesis by preventing endothelial proliferation and tube formation (Citation30,Citation31). However, TNMD-deficient mice did not exhibit any vascular abnormalities. Thus the loss of TNMD expression did not affect tendon vessel density, and mice lacking both TNMD and CHM had normal retinal vascularization and neovascularization after oxygen-induced retinopathy (Citation25). Since angiogenesis is regulated by a multitude of other factors, this could be explained by compensatory mechanisms.
The ablation of TNMD expression by gene targeting did not affect the body size, basic histology of the main organs, or skeletal development of mice (Citation25). The viability and life-span of these mice were also normal (Citation25). The only observed consequence was the reduced tenocyte density due to impaired proliferation and an altered structure of collagen fibrils. However, despite the lower cell numbers, the tendons of knock-out mice were of the same size as those of the wild-type mice, suggesting that either the remaining tenocytes were able to compensate for the loss of cells or that the turn-over of extracellular matrix was delayed in the TNMD-deficient tendons (Citation25). The necessity of TNMD for tenocyte proliferation and tendon maturation has been demonstrated also in other in vivo studies with mice (Citation32) and chicks (Citation33). For a thorough review on the functional properties, the reviews by Hiraki et al. and Shukunami et al. are recommended (Citation23,Citation34,Citation35).
Expression profile and tissue distribution
TNMD is mainly expressed in hypovascular connective tissues such as tendons, ligaments, and eye (Citation21,Citation22,Citation25). In addition, other human tissues that exhibit relatively high mRNA expression of TNMD are cardiac myocytes, tongue, and certain regions of brain, such as temporal lobe and globus pallidus (Citation36). Recently, Kimura et al. showed that TNMD is abundantly expressed in chordae tendinae cordis, which are tendon-like structures anchoring the cardiac valve leaflets to papillary muscles (Citation27). They also observed that TNMD was locally absent in the ruptured areas of chordae tendinae cordis, while the expression of CHM was similar in normal and ruptured chordae. Thus, despite the high similarity between CHM and TNMD, the expression profiles of these angiogenesis inhibitors are not fully identical.
Three independent studies have shown that TNMD is expressed in human AT (Citation11–13), but not in AT of mice (Citation36) (http://symatlas.gnf.org/SymAtlas/). On the basis of expression data from 65 different human tissues, obtained from the Gene Expression Omnibus database (http://www.ncbi.nlm.nih.gov/geo/), subcutaneous AT has the highest expression levels of TNMD, followed by AT of undefined origin, mammary gland, and omental AT (Citation13). The expression of TNMD was over 3-fold higher in isolated human adipocytes and adipose tissue in comparison to other human tissue, and the expression in the isolated stromal vascular fraction cells was similar to that in whole subcutaneous adipose tissue (Citation13). Thus, it seems that TNMD is expressed in both adipocyte and stromal vascular fractions. Our preliminary results show that also the tenomodulin protein is expressed in these fractions, since both adipocyte cell walls and blood vessels show positive immunostaining (data not shown).
We have previously reported that the expression levels of TNMD are strongly correlated with BMI and various obesity-related parameters and that weight reduction down-regulates the expression of TNMD in the AT of overweight individuals (Citation11). Recently, Saiki et al. also reported similar observations and showed also that obese individuals have higher expression of TNMD than lean persons (Citation13). We have also shown that women have 2-fold expression levels of TNMD in comparison to men (Citation11). This is probably due to the X-chromosomal location. Saiki et al. observed a similar gender difference among the obese, but not among the lean individuals (Citation13).
Regulators of tenomodulin expression
The regulators of TNMD expression are not very well known. Scleraxis, a transcription factor and a tendon-specific marker (Citation37), has been shown to up-regulate TNMD expression in chick embryo and tenocyte cultures (Citation27), and myostatin (MSTN) up-regulates TNMD expression in tendon fibroblasts (Citation32). Myostatin belongs to the transforming growth factor-β superfamily, a group of secreted growth and differentiation factors that are essential for regulation of tissue development and homeostasis. The members of this family are involved in myogenesis, angiogenesis, and adipogenesis (Citation39–42).
The MSTN gene has been widely studied, since the alterations in its expression affect the body composition. MSTN-deficiency causes muscle hypertrophy in animals (Citation41,Citation42), and a similar human phenotype has been reported (Citation43). Correspondingly, MSTN over-expression leads to decreased adipogenesis (Citation45). Rebbapragada et al. (Citation45) first demonstrated that MSTN blocks adipogenesis in both mesenchymal precursor cells and pre-adipocytes. Subsequently, Feldman et al. (Citation44) showed that myostatin modulates adipogenesis so that the generated adipocytes had favourable metabolic characteristics including reduced lipid accumulation and high insulin sensitivity. MSTN has not been studied in the context of human obesity, but it has been claimed that weight loss significantly down-regulates the expression of MSTN in the skeletal muscle of morbidly obese persons (Citation46).
Genetic association studies with TNMD
Since TNMD is located in the X chromosome, it has often been excluded from the reports of genome-wide scans, although the X-chromosomal data are publicly available for some of the studies (e.g. http://www.wtccc.org.uk/). The progress in statistical methodology also allows the analysis of X-chromosomal data simultaneously from both genders. Thus the knowledge on non-autosomal genetic risk factors, acquired from genome-wide studies, is likely to increase in future.
In comparison to the hypothesis-free genome-wide scans, the traditional candidate gene studies are hypothesis-driven with the assumption that genes with functions relevant to the phenotype of interest would represent putative susceptibility genes (Citation47). In our group, this approach has been applied by studying genes whose expression in AT is differentially regulated by weight loss. TNMD was one of the most down-regulated genes in AT during moderate weight loss in overweight individuals in our previous study (Citation11) as well as in studies by other groups (Citation12,Citation13). Given these observations and the interesting biological function, TNMD was selected as a potential candidate gene for obesity and obesity-related phenotypes and diseases, including obesity and anthropometric measurements, inflammation, glucose or lipid metabolism, age-related macular degeneration (AMD), and Alzheimer's disease (AD). The performed association studies are summarized in , and the selected markers in each study are shown in .
Table I. Summary of genetic association studies performed with tenomodulin as a candidate gene.
Associations with body size
Associations with anthropometric measurements were investigated in two study populations, the Finnish Diabetes Prevention Study (DPS) (Citation48,Citation49) and the Metabolic Syndrome in Men Study (METSIM) (Citation50) ().
In the DPS, rs11798018 was associated with BMI and weight during the 3-year follow-up in men, but not at base-line. The persons with the A allele had lower BMI than individuals with the C allele () (Citation14). In the METSIM only the marker rs2073162 was genotyped, because it was associated with T2D and was in strong linkage disequilibrium with two other markers which were related to T2D risk in the DPS. This marker was not associated with BMI, weight, or indicators of central obesity in the METSIM (Citation16) or in the DPS (Citation14). In women, the most consistent associations, both in cross-sectional and longitudinal analysis, were observed with rs4828037 and rs5966709 and different parameters of central obesity () (Citation14). In addition, rs2073162 was associated with horizontal diameter at base-line.
Table II. The observed associations of the TNMD single-nucleotide polymorphisms with anthropometric measurements in the Finnish Diabetes Prevention Study (DPS). Numeric data are given as median (interquartile range).
Associations with glucose metabolism and type 2 diabetes
In the DPS, no genotype differences in insulin and glucose levels were observed at base-line, except for rs2073162 in women (Citation14). Specifically, the rs2073162-GG genotype was associated with lower fasting plasma glucose levels (FPG) than the other genotypes (P = 0.021). The median (interquartile range) FPG was 5.89 (0.88) mmol/L for the carriers of GG genotype, 6.21 (1.03) mmol/L for GA, and 6.16 (0.87) mmol/L for the AA genotype. In women, the same marker was associated with 2h-PG concentrations (2-hour plasma glucose level in an oral glucose tolerance test) during the 3-year follow-up, but, contradictory to the base-line results, the lowest levels were observed with the AA genotype.
Among the men of the DPS, the markers rs2073163 and rs1155974 were associated with 2h-PG during the 3-year follow-up (Citation14). The marker rs2073163 was also associated with conversion of impaired glucose tolerance to T2D in men in the DPS, and a border-line association was observed with rs1155974. The individuals with the minor alleles (rs2073163-C or rs1155974-T), which were associated with higher 2-hour plasma glucose concentration, were approximately two times more likely to develop T2D during the 5-year follow-up than the major allele carriers. A similar association was also observed with rs2073162, which was not associated with 2h-PG. In women, none of the markers contributed to the risk of T2D. In contrast to the observations in the DPS, the marker rs2073162 was not associated with plasma glucose or serum insulin concentrations during oral glucose tolerance test in the METSIM study, and the prevalence of T2D was also similar between the genotypes (Citation16).
Associations with serum levels of systemic immune mediators
The association of the common sequence variation in the TNMD gene with serum levels of different systemic immune mediators was addressed in the base-line data of the DPS. In addition, the modification of effect by the status of glucose tolerance, central obesity, and general body size (indicated by 2h-PG, waist circumference, and BMI, respectively) was assessed.
The three markers, rs2073162, rs2073163, and rs1155974, which were associated with the risk of T2D in men in the DPS (Citation14), were associated with serum concentrations of C-reactive protein and serum amyloid A so that the individuals harbouring the genotypes (rs2073162-A, rs2073163-C, and rs1155974-T) related to higher risk of T2D also had higher serum levels of these acute phase reactants (Citation15). The markers rs2073163 and rs1155974 were also associated with the serum levels of soluble intercellular adhesion molecule so that the men with the rs2073163-C or rs1155974-T genotypes had higher concentrations than the individuals with the other genotypes. In addition, two markers, rs5966709 and rs4828037, were associated with serum levels of chemokine (C-C motif) ligand (CCL)-5 in men so that the rs5966709-G and rs4828037-T genotypes had higher serum concentrations (Citation15).
In women, the same genotypes were associated with elevated serum concentrations of CCL-3, and the rs5966709-GG but not rs4828037-TT genotype was associated with higher CCL-5 concentrations (Citation15). Both of these markers were associated with central obesity in women (Citation14). Furthermore, four markers (rs2073162, rs2073163, rs4828038, and rs1155974), which are in relatively strong linkage disequilibrium, were associated with serum concentrations of macrophage migration inhibitory factor (Citation15).
The genotype effects were modified by the status of glucose metabolism so that the effect was generally clearer in the individuals who had 2h-PG above the median value. In addition, central obesity, as reflected in the waist circumference, modified the effect in a similar manner, i.e. the genotype effect was more pronounced in the upper medians (Citation15).
Associations with serum lipids and lipoproteins
Strong genotype*BMI interactions were observed in the METSIM study population, although the genotype of rs2073162 was not associated with the serum lipid or lipoprotein concentrations in the unstratified METSIM population (Citation16). In subsequent analyses, the data were divided to the quartiles of BMI. No associations were evident in the three lowest quartiles. However, in the highest quartile (individuals with BMI > 29.41 kg/m2), the carriers of the rs2073162-A allele had higher concentrations of serum total, low-density lipoprotein (LDL), and high-density lipoprotein (HDL) cholesterol than carriers of the rs2073162-G allele, even after additional adjustment for use of cholesterol-lowering medication () (Citation16). No differences were observed in the triglyceride levels.
Table III. The association of the rs207162 of TNMD with serum lipoprotein levels in the men of the Finnish Diabetes Prevention Study (DPS) and the Metabolic Syndrome in Men Study (METSIM). Numeric data are given as median (interquartile range).
In the DPS, genotype differences were not observed in either gender when all individuals were included in the analysis, but again genotype*BMI interactions were observed. In the data stratified by the median of BMI, the genotype was not associated with the serum lipoproteins or lipids in either median in women or in the lower median in men. In the upper median of BMI in men (BMI > 29.45 kg/m2), the genotype of rs2073162 was associated with serum levels of total and LDL cholesterol (). No significant associations with serum HDL cholesterol or triglyceride levels were observed.
Associations with age-related macular degeneration
Age-related macular degeneration (AMD) can be divided into atrophic and exudative forms, the latter being more common and accounting for approximately 80% of AMD cases (Citation51). Choroidal neovascularization and leakage from the blood vessels are diagnostic markers for the exudative AMD (Citation52), and thus the angiogenesis regulators are interesting candidate genes also for AMD. In addition to a substantial genetic component (Citation53), environmental risk factors such as aging, smoking, high body mass index, and obesity-related conditions like hypertension and hypercholesterolemia predispose to AMD (Citation54–56).
The AMD study population consisted of 475 Finnish subjects (162 men, 313 women); 89 men and 175 women had exudative AMD, and 18 men and 25 women had atrophic AMD. The control group consisted of 55 men and 113 women. In women, markers rs7890586 and 1155974 were associated with total prevalence (atrophic or exudative form) of AMD, and a trend was observed with rs2073163 (Citation17). In comparison to women with other genotypes, the women who were homozygous for the minor allele (genotypes rs1155974-TT or rs2073163-CC) had a 2.60-fold (95% confidence interval (CI) 1.15–5.83) or 1.9-fold (95% CI 0.93–3.77) risk for having AMD, respectively. These differences were due to the unequal prevalence of exudative AMD. In comparison to women who were homozygous for the major alleles, the women with rs1155974-TT genotype had a 2.80-fold (95% CI 1.16–6.54), and the women with rs2073163-CC genotype had a 1.87-fold (95% CI 0.91–3.87) risk for exudative AMD. Furthermore, women carrying the rare rs7890586-AA genotype had a significantly smaller risk for having AMD than women with the other genotypes (odds ratio 0.08, 95% CI 0.02–0.0.38), but due to the low frequency of this genotype, this finding must be interpreted cautiously. None of the markers were associated with prevalence of AMD among men.
Associations with Alzheimer's disease
None of the markers associated with AD in men or women, but a gene–gene interaction was found with APOE in women () (Citation18).
Summary of genetic association studies
The most consistent associations were observed with serum lipoprotein levels () (Citation16), whereas the associations of TNMD with obesity and glucose metabolism which were observed in the DPS (Citation14) were not replicated in the METSIM, a larger, population-based sample (Citation16). This might be due to several reasons. Only the markers that associated with T2D risk in men of the DPS were genotyped in the METSIM. These markers did not associate with body size in the DPS. Furthermore, the association with obesity measures in men was observed in the longitudinal data, which was not available from the METSIM. The DPS included participants from five Finnish cities and their surroundings. These individuals were relatively homogeneous as all of the study participants had BMI > 25 kg/m2 and impaired glucose tolerance. The METSIM population is a random sample of men aged from 45 to 70 years and living in the Kuopio area. Therefore, the range for BMI is considerably larger (16.18–52.11 kg/m2), and all four glucose tolerance categories were included. Furthermore, in the METSIM the prevalence of T2D was analysed, while in the DPS the conversion of IGT to T2D was assessed.
The associations with systemic immune mediators, AMD, and AD were also studied in single populations. Due to the different amount of background data in the study populations, it is difficult to draw conclusions on the mechanisms behind the observed associations. However, obesity, inflammation, and perturbations in glucose and lipid metabolism are strongly interconnected. In addition, these states have been linked to pathogenesis of both AD and AMD. Therefore, it is not surprising that a gene related to obesity could also be associated with related conditions and co-morbidities.
Putative role of TNMD in the disease development
The novel findings of Saiki et al. (Citation13) provide a new perspective to the results of genetic association studies discussed above. Although tenomodulin mediates anti-angiogenic effects, its specific function in AT is still unknown. Adipose tissue expresses and secretes several other angiogenesis regulators (Citation1,Citation5,Citation6,Citation9), and thrombospondin-1, an angiogenesis inhibitor with a similar knock-out mouse phenotype as TNMD, was recently shown to be an adipokine that was associated with obesity, AT inflammation, and insulin resistance (Citation9). Due to the common ground between adipogenesis and angiogenesis, the anti-angiogenic function might provide a link between TNMD and obesity.
Adipogenesis and angiogenesis are temporally and spatially coupled processes during embryogenesis, and their reciprocal cross-talk via paracrine signalling systems continues throughout adult life (Citation38). Human adipose tissue-derived stem cells can differentiate into endothelial cells and improve postnatal neovascularization (Citation38), and adipocytes and their accompanying endothelial cells seem to share a common progenitor that can differentiate into adipocytes or endothelial lineages depending on the type of exposure in different environments (Citation57). In normal weight individuals each adipocyte is nourished by a well organized capillary network (Citation58–60), and pro-angiogenic factors, secreted by adipose tissue, stimulate neovascularization during fat mass expansion, either acting alone or in co-operation with angiogenic mediators secreted from other tissues (Citation3,Citation38,Citation59). It has been speculated that when the growth rate of adipose tissue becomes stabilized, high expression levels of angiogenesis inhibitors are required to restrict further vessel growth (Citation38), which is in line with the up-regulation of TNMD and thrombospondin 1 expression in obese and overweight individuals. Furthermore, the inhibition of the vascular endothelial growth factor (VEGF) signalling system inhibits angiogenesis and pre-adipocyte differentiation (Citation61).
The altered vascularization in obesity has been demonstrated in animal models: the fat pads of obese mice have increased vascularization (Citation62), and fat pads of obese rats have increased perfusion and decreased vascular resistance (Citation63). Voros et al. (Citation62) showed that the increased blood content of adipose tissue in obese animals was not only the consequence of functional modulations but also resulted from the growth of the vascular network. In the same study, the protein expression of angiogenesis-promoting angiopoietin-1 was lower, and the expression of TSP-1 was higher in the adipose tissue of ob/ob mice when compared to the corresponding expression levels in the wild-type mice. Animal studies have also demonstrated that targeted induction of apoptosis in the vasculature of adipose tissue can reverse obesity and normalize metabolism in ob/ob mice (Citation64), and the administration of angiogenesis inhibitors (angiostatin and endostatin) reverses both genetic and diet-induced obesity in mice (Citation65,Citation66). Since angiostatin and endostatin specifically target endothelial cells, these effects are due to the anti-angiogenic properties of these molecules (Citation65,Citation66). It has also been suggested that changes in AT blood flow may modulate the β-cell dysfunction in T2D in a rat model (Citation67). However, these data are solely based on animal studies. Still, one possibility is that tenomodulin could regulate vasculature formation in AT and thereby also modulate adiposity, glucose metabolism, and T2D risk.
In addition to obesity, angiogenesis provides an interesting link between TNMD, AMD, and AD. The harmful new blood vessels are diagnostic for exudative AMD (Citation51), and angiogenetic changes have been linked to pathogenesis of AD (Citation68,Citation69). The other functional domain, BRICHOS, has been linked to endoplasmic reticulum (ER) stress and proteasome dysfunction (Citation26), which provides another interesting functional connection between TNMD and phenotypes listed in . It has also been shown that obesity increases ER stress in human subcutaneous AT (Citation70) and that the unfolded protein response, a mechanism aimed to alleviate ER stress, is activated in subcutaneous AT of obese humans (Citation71).
Interestingly, the markers rs2073162 and rs20 73163 are located within the region that encodes the BRICHOS domain, and also rs1155974 is in close vicinity. These markers were associated with T2D, central obesity, AMD, and inflammation (Citation14–17). The ER chaperones (Citation72) and ER stress in general (Citation73) are known to affect the expression of angiogenic factors. Thus, TNMD could, in theory, affect the vascularization via ER stress caused by the dysfunction of the BRICHOS domain and the resulting accumulation of misfolded TNMD. Mutations in the BRICHOS region of surfactant protein C have been shown to increase ER stress by this mechanism (Citation26).
Conclusions
Adipogenesis and angiogenesis are closely coupled during the developmental period, and their cross-talk continues throughout adult life (Citation39). Thus the genes involved in angiogenesis are an interesting group of susceptibility genes for obesity and related traits. Candidate gene studies have associated the sequence variation of TNMD with glucose metabolism, serum lipoprotein, and inflammatory marker levels in men, and with central obesity, serum levels of systemic immune mediators, and exudative AMD in women. These phenotypes are linked by inflammation and angiogenesis.
It might be that the association of TNMD with inflammation could explain many of the observed associations in men. It has been speculated that acute phase reactants and other inflammatory factors can affect lipolysis and cholesterol synthesis (Citation74). It is also known that lipid overload interferes with insulin signalling pathways and that enhanced lipolysis and dyslipidaemias contribute to the deterioration in glucose metabolism in obesity (Citation75). The hypothesis of inflammation as a linking mechanism is supported by the observation that the same genotype associated with elevated serum concentrations of acute phase reactants in the DPS (Citation15) was related to elevated serum LDL levels in obese individuals in the DPS and the METSIM, and also to increased HDL levels in the METSIM (Citation16).
In women, the same markers that were associated with central obesity (Citation14) were associated with elevated serum concentrations of CCL-3 and CCL-5 (Citation15). In addition, two markers which were linked to concentrations of CCL-5 and macrophage migration inhibitory factor (Citation15) were associated with the risk of AMD (Citation17). Therefore, one possible scheme is that the elevated serum levels of inflammatory factors, secondary to central obesity, facilitate the pathophysiological changes related to development of AMD. In addition, due to its angiogenesis-inhibiting properties, TNMD might also contribute to the altered neovascularization. However, we emphasize that although the observations from genetic association studies are interesting, they certainly need confirmation in independent studies.
Experimental data support the role of angiogenesis mediators in regulation of fat mass and obesity-associated co-morbidities, such as diabetes (Citation64–67). Since TNMD is up-regulated by myostatin, an anti-adipogenic factor (Citation32), it is tempting to speculate that the increased TNMD expression in obesity might be a protective mechanism aimed at limiting growth of new blood vessels during more stabilized periods of adipose tissue expansion (Citation38). This is supported by the finding that weight loss down-regulates the expression of MSTN in morbidly obese persons (Citation46). However, without the knowledge of haemodynamics in the adipose tissue of TNMD knock-out mice or mice with ablation or over-expression of MSTN, it is impossible to conclude whether this is the mechanism.
Declaration of interest: The authors report no conflicts of interest. The authors alone are responsible for the content and writing of the paper.
References
- Trayhurn P, Wood IS.. Adipokines: inflammation and the pleiotropic role of white adipose tissue. Br J Nutr. 2004;92: 347–55.
- Berg AH, Scherer PE. Adipose tissue, inflammation, and cardiovascular disease. Circ Res. 2005;96:939–49.
- Cao R, Brakenhielm E, Wahlestedt C, Thyberg J, Cao Y. Leptin induces vascular permeability and synergistically stimulates angiogenesis with FGF-2 and VEGF. Proc Natl Acad Sci U S A. 2001;98:6390–5.
- Smith U. Impaired (‘diabetic’) insulin signaling and action occur in fat cells long before glucose intolerance—is insulin resistance initiated in the adipose tissue? Int J Obes Relat Metab Disord. 2002;26:897–904.
- Ledoux S, Queguiner I, Msika S, Calderari S, Rufat P, Gasc JM, . Angiogenesis associated with visceral and subcutaneous adipose tissue in severe human obesity. Diabetes. 2008;57:3247–57.
- Wang P, Mariman E, Renes J, Keijer J. The secretory function of adipocytes in the physiology of white adipose tissue. J Cell Physiol. 2008;216:3–13.
- Wu H, Ghosh S, Perrard XD, Feng L, Garcia GE, Perrard JL, . T-cell accumulation and regulated on activation, normal T cell expressed and secreted upregulation in adipose tissue in obesity. Circulation. 2007;115:1029–38.
- Haque WA, Shimomura I, Matsuzawa Y, Garg A. Serum adiponectin and leptin levels in patients with lipodystrophies. J Clin Endocrinol Metab. 2002;87:2395.
- Varma V, Yao-Borengasser A, Bodles AM, Rasouli N, Phanavanh B, Nolen GT, . Thrombospondin-1 is an adipokine associated with obesity, adipose inflammation, and insulin resistance. Diabetes. 2008;57:432–9.
- Kloting N, Berndt J, Kralisch S, Kovacs P, Fasshauer M, Schon MR, . Vaspin gene expression in human adipose tissue: association with obesity and type 2 diabetes. Biochem Biophys Res Commun. 2006;339:430–6.
- Kolehmainen M, Salopuro T, Schwab US, Kekalainen J, Kallio P, Laaksonen DE, . Weight reduction modulates expression of genes involved in extracellular matrix and cell death: the GENOBIN study. Int J Obes (Lond). 2008;32: 292–303.
- Dahlman I, Linder K, Arvidsson Nordstrom E, Andersson I, Liden J, Verdich C, . Changes in adipose tissue gene expression with energy-restricted diets in obese women. Am J Clin Nutr. 2005;81:1275–85.
- Saiki A, Olsson M, Jernas M, Gummesson A, McTernan PG, Andersson J, . Tenomodulin is highly expressed in adipose tissue, increased in obesity and down regulated during diet-induced weight loss. J Clin Endocrinol Metab. 2009; 94:3987–94.
- Tolppanen AM, Pulkkinen L, Kolehmainen M, Schwab U, Lindstrom J, Tuomilehto J, . Tenomodulin is associated with obesity and diabetes risk: the Finnish diabetes prevention study. Obesity (Silver Spring). 2007;15:1082–8.
- Tolppanen AM, Pulkkinen L, Herder C, Koenig W, Kolehmainen M, Lindstrom J, . The genetic variation of the tenomodulin gene (TNMD) is associated with serum levels of systemic immune mediators—the Finnish Diabetes Prevention Study. Genet Med. 2008;10:536–44.
- Tolppanen AM, Pulkkinen L, Kuulasmaa T, Kolehmainen M, Schwab U, Lindstrom J, . The genetic variation in the tenomodulin gene is associated with serum total and LDL cholesterol in a body size-dependent manner. Int J Obes (Lond). 2008;32:1868–72.
- Tolppanen AM, Nevalainen T, Kolehmainen M, Seitsonen S, Immonen I, Uusitupa M, . Single nucleotide polymorphisms of the tenomodulin gene (TNMD) in age-related macular degeneration. Mol Vis. 2009;15:762–70.
- Tolppanen AM, Helisalmi S, Hiltunen M, Kolehmainen M, Schwab U, Pirttila T, . Tenomodulin variants, APOE and Alzheimer's disease in a Finnish case-control cohort. Neurobiol Aging. 2009 Jun 11 (Epub ahead of print).
- Cros N, Tkatchenko AV, Pisani DF, Leclerc L, Leger JJ, Marini JF, . Analysis of altered gene expression in rat soleus muscle atrophied by disuse. J Cell Biochem. 2001;83:508–19.
- Yamana K, Wada H, Takahashi Y, Sato H, Kasahara Y, Kiyoki M. Molecular cloning and characterization of CHM1L, a novel membrane molecule similar to chondromodulin-I. Biochem Biophys Res Commun. 2001;280:1101–6.
- Shukunami C, Oshima Y, Hiraki Y. Molecular cloning of tenomodulin, a novel chondromodulin-I related gene. Biochem Biophys Res Commun. 2001;280:1323–7.
- Brandau O, Meindl A, Fassler R, Aszodi A. A novel gene, tendin, is strongly expressed in tendons and ligaments and shows high homology with chondromodulin-I. Dev Dyn. 2001;221:72–80.
- Shukunami C, Oshima Y, Hiraki Y. Chondromodulin-I and tenomodulin: a new class of tissue-specific angiogenesis inhibitors found in hypovascular connective tissues. Biochem Biophys Res Commun. 2005;333:299–307.
- Sanchez-Pulido L, Devos D, Valencia A. BRICHOS: a conserved domain in proteins associated with dementia, respiratory distress and cancer. Trends Biochem Sci. 2002;27: 329–32.
- Docheva D, Hunziker EB, Fassler R, Brandau O. Tenomodulin is necessary for tenocyte proliferation and tendon maturation. Mol Cell Biol. 2005;25:699–705.
- Mulugeta S, Nguyen V, Russo SJ, Muniswamy M, Beers MF. A surfactant protein C precursor protein BRICHOS domain mutation causes endoplasmic reticulum stress, proteasome dysfunction, and caspase 3 activation. Am J Respir Cell Mol Biol. 2005;32:521–30.
- Kimura N, Shukunami C, Hakuno D, Yoshioka M, Miura S, Docheva D, . Local tenomodulin absence, angiogenesis, and matrix metalloproteinase activation are associated with the rupture of the chordae tendineae cordis. Circulation. 2008;118:1737–47.
- Hiraki Y, Tanaka H, Inoue H, Kondo J, Kamizono A, Suzuki F. Molecular cloning of a new class of cartilage-specific matrix, chondromodulin-I, which stimulates growth of cultured chondrocytes. Biochem Biophys Res Commun. 1991;175:971–7.
- Hiraki Y, Inoue H, Iyama K, Kamizono A, Ochiai M, Shukunami C, . Identification of chondromodulin I as a novel endothelial cell growth inhibitor. Purification and its localization in the avascular zone of epiphyseal cartilage. J Biol Chem. 1997;272:32419–26.
- Oshima Y, Sato K, Tashiro F, Miyazaki J, Nishida K, Hiraki Y, . Anti-angiogenic action of the C-terminal domain of tenomodulin that shares homology with chondromodulin-I. J Cell Sci. 2004;117(Pt 13):2731–44.
- Oshima Y, Shukunami C, Honda J, Nishida K, Tashiro F, Miyazaki J, . Expression and localization of tenomodulin, a transmembrane type chondromodulin-I-related angiogenesis inhibitor, in mouse eyes. Invest Ophthalmol Vis Sci. 2003;44:1814–23.
- Mendias CL, Bakhurin KI, Faulkner JA. Tendons of myostatin-deficient mice are small, brittle, and hypocellular. Proc Natl Acad Sci U S A. 2008;105:388–93.
- Shukunami C, Takimoto A, Oro M, Hiraki Y. Scleraxis positively regulates the expression of tenomodulin, a differentiation marker of tenocytes. Dev Biol. 2006;298: 234–47.
- Hiraki Y, Shukunami C. Angiogenesis inhibitors localized in hypovascular mesenchymal tissues: chondromodulin-I and tenomodulin. Connect Tissue Res. 2005;46:3–11.
- Shukunami C, Hiraki Y. Chondromodulin-I and tenomodulin: the negative control of angiogenesis in connective tissue. Curr Pharm Des. 2007;13:2101–12.
- Su AI, Cooke MP, Ching KA, Hakak Y, Walker JR, Wiltshire T, . Large-scale analysis of the human and mouse transcriptomes. Proc Natl Acad Sci U S A. 2002;99:4465–70.
- Schweitzer R, Chyung JH, Murtaugh LC, Brent AE, Rosen V, Olson EN, . Analysis of the tendon cell fate using Scleraxis, a specific marker for tendons and ligaments. Development. 2001;128:3855–66.
- Cao Y. Angiogenesis modulates adipogenesis and obesity. J Clin Invest. 2007;117:2362–8.
- McPherron AC, Lawler AM, Lee SJ. Regulation of skeletal muscle mass in mice by a new TGF-beta superfamily member. Nature. 1997;387:83–90.
- McPherron AC, Lee SJ. Suppression of body fat accumulation in myostatin-deficient mice. J Clin Invest. 2002;109: 595–601.
- Kambadur R, Sharma M, Smith TP, Bass JJ. Mutations in myostatin (GDF8) in double-muscled Belgian Blue and Piedmontese cattle. Genome Res. 1997;7:910–6.
- Lin J, Arnold HB, Della-Fera MA, Azain MJ, Hartzell DL, Baile CA. Myostatin knockout in mice increases myogenesis and decreases adipogenesis. Biochem Biophys Res Commun. 2002;291:701–6.
- Schuelke M, Wagner KR, Stolz LE, Hubner C, Riebel T, Komen W, . Myostatin mutation associated with gross muscle hypertrophy in a child. N Engl J Med. 2004;350: 2682–8.
- Feldman BJ, Streeper RS, , Farese RV JrYamamoto KR. Myostatin modulates adipogenesis to generate adipocytes with favorable metabolic effects. Proc Natl Acad Sci U S A. 2006;103:15675–80.
- Rebbapragada A, Benchabane H, Wrana JL, Celeste AJ, Attisano L. Myostatin signals through a transforming growth factor beta-like signaling pathway to block adipogenesis. Mol Cell Biol. 2003;23:7230–42.
- Milan G, Dalla Nora E, Pilon C, Pagano C, Granzotto M, Manco M, . Changes in muscle myostatin expression in obese subjects after weight loss. J Clin Endocrinol Metab. 2004;89:2724–7.
- Comuzzie AG, Williams JT, Martin LJ, Blangero J. Searching for genes underlying normal variation in human adiposity. J Mol Med. 2001;79:57–70.
- Tuomilehto J, Lindström J, Eriksson JG, Valle TT, Hämäläinen H, Ilanne-Parikka P, . Prevention of type 2 diabetes mellitus by changes in lifestyle among subjects with impaired glucose tolerance. N Engl J Med. 2001;344:1343–50.
- Eriksson J, Lindström J, Valle T, Aunola S, Hämäläinen H, Ilanne-Parikka P, . Prevention of type II diabetes in subjects with impaired glucose tolerance: the Diabetes Prevention Study (DPS) in Finland. Study design and 1-year interim report on the feasibility of the lifestyle intervention programme. Diabetologia. 1999;42:793–801.
- Wang J, Kuusisto J, Vanttinen M, Kuulasmaa T, Lindstrom J, Tuomilehto J, . Variants of transcription factor 7-like 2 (TCF7L2) gene predict conversion to type 2 diabetes in the Finnish Diabetes Prevention Study and are associated with impaired glucose regulation and impaired insulin secretion. Diabetologia. 2007;50:1192–200.
- Gehrs KM, Anderson DH, Johnson LV, Hageman GS. Age-related macular degeneration—emerging pathogenetic and therapeutic concepts. Ann Med. 2006;38:450–471.
- Beatty S, Koh H, Phil M, Henson D, Boulton M. The role of oxidative stress in the pathogenesis of age-related macular degeneration. Surv Ophthalmol. 2000;45:115–34.
- Seddon JM, Cote J, Page WF, Aggen SH, Neale MC. The US twin study of age-related macular degeneration: relative roles of genetic and environmental influences. Arch Ophthalmol. 2005;123:321–7.
- Hyman L, Neborsky R. Risk factors for age-related macular degeneration: an update. Curr Opin Ophthalmol. 2002; 13:171–5.
- Buch H, Vinding T, la Cour M, Jensen GB, Prause JU, Nielsen NV. Risk factors for age-related maculopathy in a 14-year follow-up study: the Copenhagen City Eye Study. Acta Ophthalmol Scand. 2005;83:409–18.
- van Leeuwen R, Ikram MK, Vingerling JR, Witteman JC, Hofman A, de Jong PT. Blood pressure, atherosclerosis, and the incidence of age-related maculopathy: the Rotterdam Study. Invest Ophthalmol Vis Sci. 2003;44:3771–7.
- Planat-Benard V, Silvestre JS, Cousin B, Andre M, Nibbelink M, Tamarat R, . Plasticity of human adipose lineage cells toward endothelial cells: physiological and therapeutic perspectives. Circulation. 2004;109:656–63.
- Silverman KJ, Lund DP, Zetter BR, Lainey LL, Shahood JA, Freiman DG, . Angiogenic activity of adipose tissue. Biochem Biophys Res Commun. 1988;153:347–52.
- Bouloumie A, Lolmede K, Sengenes C, Galitzky J, Lafontan M. Angiogenesis in adipose tissue. Ann Endocrinol (Paris). 2002;63(2 Pt 1):91–5.
- Larson DR, Zipfel WR, Williams RM, Clark SW, Bruchez MP, Wise FW, . Water-soluble quantum dots for multiphoton fluorescence imaging in vivo. Science. 2003;300:1434–6.
- Fukumura D, Ushiyama A, Duda DG, Xu L, Tam J, Krishna V, . Paracrine regulation of angiogenesis and adipocyte differentiation during in vivo adipogenesis. Circ Res. 2003; 93:e88–97.
- Voros G, Maquoi E, Demeulemeester D, Clerx N, Collen D, Lijnen HR. Modulation of angiogenesis during adipose tissue development in murine models of obesity. Endocrinology. 2005;146:4545–54.
- Crandall DL, Goldstein BM, Lizzo FH, Gabel RA, Cervoni P. Hemodynamics of obesity: influence of pattern of adipose tissue cellularity. Am J Physiol. 1986;251(2 Pt 2):R314–9.
- Kolonin MG, Saha PK, Chan L, Pasqualini R, Arap W. Reversal of obesity by targeted ablation of adipose tissue. Nat Med. 2004;10:625–32.
- Rupnick MA, Panigrahy D, Zhang CY, Dallabrida SM, Lowell BB, Langer R, . Adipose tissue mass can be regulated through the vasculature. Proc Natl Acad Sci U S A. 2002; 99:10730–5.
- Brakenhielm E, Cao R, Gao B, Angelin B, Cannon B, Parini P, . Angiogenesis inhibitor, TNP-470, prevents diet-induced and genetic obesity in mice. Circ Res. 2004;94:1579–88.
- Kampf C, Bodin B, Kallskog O, Carlsson C, Jansson L. Marked increase in white adipose tissue blood perfusion in the type 2 diabetic GK rat. Diabetes. 2005;54:2620–7.
- Meyer EP, Ulmann-Schuler A, Staufenbiel M, Krucker T. Altered morphology and 3D architecture of brain vasculature in a mouse model for Alzheimer's disease. Proc Natl Acad Sci U S A. 2008;105:3587–92.
- Thirumangalakudi L, Samany PG, Owoso A, Wiskar B, Grammas P. Angiogenic proteins are expressed by brain blood vessels in Alzheimer's disease. J Alzheimers Dis. 2006;10:111–8.
- Das SK, Chu WS, Mondal AK, Sharma NK, Kern PA, Rasouli N, . Effect of pioglitazone treatment on endoplasmic reticulum stress response in human adipose and in palmitate-induced stress in human liver and adipose cell lines. Am J Physiol Endocrinol Metab. 2008;295:E393–400.
- Boden G, Duan X, Homko C, Molina EJ, Song W, Perez O, . Increase in endoplasmic reticulum stress-related proteins and genes in adipose tissue of obese, insulin-resistant individuals. Diabetes. 2008;57:2438–444.
- Ozawa K, Tsukamoto Y, Hori O, Kitao Y, Yanagi H, Stern DM, . Regulation of tumor angiogenesis by oxygen-regulated protein 150, an inducible endoplasmic reticulum chaperone. Cancer Res. 2001;61:4206–13.
- Abcouwer SF, Marjon PL, Loper RK, Vander Jagt DL. Response of VEGF expression to amino acid deprivation and inducers of endoplasmic reticulum stress. Invest Ophthalmol Vis Sci. 2002;43:2791–8.
- Manley PN, Ancsin JB, Kisilevsky R. Rapid recycling of cholesterol: the joint biologic role of C-reactive protein and serum amyloid A. Med Hypotheses. 2006;66:784–92.
- Guilherme A, Virbasius JV, Puri V, Czech MP. Adipocyte dysfunctions linking obesity to insulin resistance and type 2 diabetes. Nat Rev Mol Cell Biol. 2008;9:367–77.