Abstract
During exercise, the plasma urate levels and urinary excretion increase due to the enhanced purine degradation in skeletal muscle. Although urate transporter-1 (URAT1) is the main transporter responsible for the reabsorption of filtered urate, potential changes in its activity and expression during exercise have not been studied yet. Therefore, the effect of heavy muscle activity on renal URAT1 activity and expression was investigated in this study. Wistar rats were used in the study and the experimental design consisted of three groups: a control group, an exercise group where animals were exhausted once a day for 5 days, and a hyperuricemia group, which was induced by an uricase inhibitor, oxonic acid. URAT1 activity measurements were performed in isolated proximal tubule segments and expression of URAT1 mRNA and protein levels were determined by the reverse transcription polymerase chain reaction and western blot analyses, respectively. Increased citrate synthase activity in soleus muscle of exercised animals proved the efficiency of our exercise protocol. Proteinuria, glucosuria, and hypoglycemia were observed only in exercised animals; however, plasma and urinary urate levels were found to be elevated in both exercising and hyperuricemia groups. Moreover, in both of the groups URAT1 transporter activity was found to be increased despite the significant decrease in URAT1 protein levels. Considering the similar changes of urate metabolism observed in both exercising and hyperuricemic rats, our results suggest that exercise-induced changes in URAT1 expression and activity depend on the increased urate concentration in plasma.
INTRODUCTION
Our previous studies indicated that exhaustive heavy muscle activity affects proximal tubule (PT) cytoresistance, as well as some of renal tubular functions.Citation1,Citation2 Previously published data revealed that in heavy physical exercise severe glucosuria and proteinuria might be observed despite the presence of PT cells with normal secretory functions.Citation3–5 Unaltered secretory functions associated with impaired glucose and protein absorption suggest the vulnerability of absorption capacity of the PT cells against exercise-induced injury.Citation6,Citation7 In case of such disparity between the absorption and secretory functions of PT cells, the occurrence of serious clinical problems based on renal reabsorption seems to be unavoidable unless some preventive adaptations develop in exercisers.
The exercising body needs normal kidney functions to eliminate metabolic waste products generated during heavy muscle activity. Elevated urate, which is the final product of purine metabolism in humans, that is released from the activated muscle during heavy physical activity is of crucial importance among the major waste products.Citation8–10 Because of uricase deficiency, humans have a tendency toward uric acid crystallization even during modest alterations in uric acid homeostasis and thus, its renal clearance keeps the plasma urate levels within normal limits.Citation11 Interestingly however, despite its potential harm, kidneys are not in a hurry to eliminate uric acid and its handling by PT cells.Citation12,Citation13 According to four-component hypothesis, following its glomerular filtration, 98% of filtered urate is reabsorbed from the S1 segment of the PT (presecretory reabsorption). Subsequently, 50% of urate is then secreted by the S2 segment into the lumen (secretion). Eventually, 40% of urate is reabsorbed once again (postsecretory reabsorption).Citation12,Citation13 Possibly, being one of the free radical scavengers, its tubular recirculation contributes to the efficient use of urate before being fully eliminated into the urine.
Regarding the elevated reactive oxygen species (ROS) and urate generation during physical activity,Citation9–10,Citation14–16 urate recirculation in the PT cells seems quite logical and may have some physiological importance in preventing hazardous effects of ROS and maintaining the integrity of the PT cells. Therefore, reduced urate reabsorption or excretion may deteriorate the maintenance of the normal uric acid homeostasis in exercisers. Urate mishandling by the PTs that alters urate excretion leads to either hyper or hypouricemia. When hyperuricemia occurs, the body will form crystals that accumulate in the joints and cause painful gouty symptoms.Citation17 Cardiovascular risks and renal injuries especially in hypertensive patients are also associated with hyperuricemia.Citation18,Citation19 On the other hand, it is known that several pathologies such as multiple sclerosis, Parkinson's and Alzheimer's diseases are accompanied by hypouricemia.Citation20,Citation21 The ability of uric acid to scavenge peroxynitrite is involved in improving certain neurological conditions associated with increased oxidative stress.Citation22 Since none of the above-mentioned pathologies was reported to occur frequently as a consequence of heavy physical activity, the normal urate handling in the PT cells is presumed to be continuous despite exercise-induced renal injury. However, some authors ruled out this assumption by indicating the reverse relation between tubular lactate secretion and urate excretion in exercisers.Citation23,Citation24
Urate transporter-1 (URAT1) localized on the apical membrane of the S1 segment of the PT cells plays a pivotal role in the 98% presecretory reabsorption of filtered urate.Citation11,Citation25 This transporter serves as an anion exchanger and secretes anions such as lactate, chloride, hydroxyl, nicotinate, acetoacetate together with the reabsorption of uric acid.Citation11,Citation26 Other anion transporters, OAT1 and OAT3, localized on the basolateral membrane were also shown to be involved in the influx of blood urate into the tubule cells but URAT1 seems to be the main transporter responsible for the reabsorption of filtered urate. This is further supported by the presence of hypouricemia and high urinary urate excretion in URAT1-mutated patients.Citation27 On the other hand, elevated blood lactate and other anion concentrations by increasing urate reabsorption leads to decrease in urate excretion which contributes to hyperuricemia. As demonstrated by Ohno et al.,Citation28 hyperuricemia is also induced by the diets rich in urate precursors during physical exercise. In their study, Ohno et al.Citation28 showed that dietary grape juice which contains considerable amount of fructose significantly increases serum urate concentration in exercisers.
Hyperuricemia associated with heavy physical activity is usually attributed to either the excess purine degradation in the active muscleCitation9,Citation10 or the elevated tubular reabsorption due to lactate and/or other organic anion trans-stimulation.Citation23,Citation24 However, the mechanisms underlying the changes in the renal urate handling seen in exercisers are not yet known. Neither the transport capacity of URAT1 nor its expression has been studied in the PT cells during exhausting exercise. Therefore, we aimed to study the functional capacity and expression of URAT1 in rats subjected to exhausting physical activity.
MATERIAL AND METHODS
In order to reveal the effects of heavy muscle activity on renal URAT1 activity and expression, 2.5–3-month-old male Wistar rats weighing 280–320 g were used. Animals were randomly divided into three groups as control (N = 10), heavy muscle activity (N = 10), and hyperuricemia (N = 10). The last group was included to differentiate the effects of exercise and exercise-induced hyperuricemia on URAT1. The rats were provided food and water ad libitum. All procedures were approved by Akdeniz University Animal Care and Usage Committee (07-05/02) and followed the guidelines established by the American Physiological Society.
Exercise protocol
As mentioned previously, in order to mimic the effect of irregular heavy muscle activity of laborers on kidney functions, 5 days exhausting muscle activity was chosen as an exercise model in our experiments.Citation1,Citation2 Animals were exhausted on a motor-driven treadmill (MAY-TME 9805, Commat, Ankara, Turkey) once a day for 5 days. Before exhaustive protocol, the rats were familiarized with treadmill running for 2 days. The exhaustive protocol was started at 20 m/min and a 5% grade for 5 min. The grade and speed were gradually increased to 15% and 24 m/min, and running was continued until exhaustion. For the animals that failed to escape from electrical stimulation, the exhaustion was approved by the loss of the straightening reflex for 10 s when animals were placed on their back.
Oxonic acid treatment
Hyperuricemia is produced by intraperitoneal injection of oxonic acid (OA), a hepatic uricase inhibitor, at a dose of 250 mg/kg body weight daily for 5 days.Citation29
All animals were placed in metabolic cages for 24 h and urine samples were collected. The exhausted and OA-treated rats were placed in metabolic cages on the fourth exercise or treatment day.
The perfusion of kidneys from anesthetized rats were manually performed through abdominal aorta with 30 mL ice-cold Krebs phosphate buffer (118 mM NaCl, 4.8 mM KCl, 2.5 mM CaCl2, 1.2 mM MgSO4, 1.2 mM KH2PO4, 24 mM NaHCO3, and 11 mM glucose; pH 7.4) to wash off the blood. Then, both kidneys were excised, cleaned from adhesive fat tissues, and decapsulated in ice-cold buffer. The cool kidneys were longitudinally dissected and the medullary part was discarded. The renal cortical tissue was used for PT isolation, western blot analysis and RNA isolation.
The blood and urine samples were used for creatinine, protein, glucose, and urate measurements. Protein and creatinine levels were determined using Lowry methodCitation30 and Jaffe method,Citation31 respectively. Glomerular filtration rate (GFR) was estimated by creatinine clearance. Appropriate commercial kits were used for glucose (QuantiChrom™ Glucose Assay Kit, BioAssay Systems, CA, USA) and urate (Amplex® Red Uric Acid/Uricase Assay Kit, Invitrogen, Paisley, UK) measurements.
Measurement of the mean arterial pressure
Under the light ether anesthesia mean arterial pressure (MAP) was determined by tail-cuff method (Biopac, BP HR200 module plus MP100 system, Goleta, CA, USA).
Measurement of the renal blood flow
The renal plasma flow (RPF) was calculated as a clearance of para-aminohippuric acid (PAH), and renal blood flow (RBF) was calculated as the clearance of RPF/(1-Hct). Under the urethane anesthesia (1 mg/kg bw, ip), the left jugular vein and urinary bladder were cannulated for PAH infusion (0.6%) and urine collection, respectively.Citation32 Plasma and urine PAH concentrations were determined by spectrophotometer.Citation33
Activity of citrate synthase
Citrate synthase (CS) activity was determined for the soleus muscle of each rat according to the spectrophotometric method described by Srere.Citation34 Data were expressed as micromoles per gram wet weight per minute.
PT isolation
Isolation of PT was performed according to Vinay et al.Citation35 Briefly, minced renal cortices were incubated for 1 h at 37°C in 30 mL of 0.15% collagenase, while continuously being gassed with 95% O2 and 5% CO2. The digested tissue suspensions were washed and resuspended in Krebs buffer. After the Percoll density gradient centrifugation, the tissue was separated into four distinct bands. The band at the bottom that was enriched in PTs was withdrawn and washed twice with ice-cold Krebs buffer and used for URAT1 activity measurements.
URAT1 activity measurement
URAT1 mediated urate uptake is accepted as the transporter activity in the PT segments. As defined in the following paragraph, 300 μg isolated PT segments were incubated in Krebs phosphate buffer containing with and without 100 μM urate at 37°C for 30 min in the presence of suitable transporter inhibitors such as 100 μM Morin (for URAT1), 50 μM Cefadroxil (for OAT1 and OAT3), 5 μM Xhantin (for UAT), and 10 μM MK571 (for MRP4).
To evaluate the contribution of URAT1 in urate influx the following protocols were performed on the isolated PT segments.
Protocol 0: For estimation of basal urate content of tubules, PT segments were incubated in Krebs phosphate buffer without any exogenous urate at 37°C for 30 min.
Protocol 1: To measure the total urate uptake, PT segments subjected to inhibitors of known urate effluxers, UAT and MRP4, were incubated as in Protocol 0, but in the presence of 100 μM urate.
Protocol 2: To determine the amount of urate influx into PT cells through undefined transporters, PT segments were incubated in the presence of 100 μM urate and above-mentioned inhibitors.
Protocol 3: Similar to Protocol 2 but without the addition of Morin, an URAT1 inhibitor.
After 30 min incubation, PT segments were washed twice with ice-cold Krebs containing all inhibitors. Then, tubule cells were disrupted by sonication. After the centrifugation, the urate content in the supernatant was measured using urate kit (Amplex® Red Uric Acid/Uricase Assay Kit, Invitrogen, Paisley, UK).
Subtraction of P2 value from that of P1 was used to estimate the amount of influxed urate via apical and basolateral transporters and the difference between the urate value of P3 and P2 was used to calculate the URAT1 mediated urate transport.
Reverse transcription polymerase chain reaction
From kidney cortex total RNA was extracted using the RNeasy protecting mini kit (Qiagen) and reverse transcribed using Sensiscript RT Kit (Qiagen). The cDNA was then amplified by polymerase chain reaction (PCR) for 35 cycles (30 s at 94°C, 30 s at 52°C, and 40 s at 72°C) using the primer pair specific to a 230 bp region of the URAT1 mRNA (forward: 5′-gcacaatagctgccctcatgc; reverse: 5′-gccaactgcagcatcctcc). As an internal control, the β-actin mRNA was used and a 280 bp region of β-actin mRNA was amplified along with URAT1 using the primers 5′-ggagaagagctatgagctgc (forward) and 5′-gcaatgatcttgatcttcatgg (reverse). The PCR products were visualized under UV light following electrophoresis on a 2% agarose gel and ethidium bromide staining. The intensities of the bands were quantified by densitometric analysis using the NIH Image J 1.41 program (National Institutes of Health) and normalized to that of the β-actin.
Western blotting
Using a Teflon-glass tissue homogenizer, renal cortical tissues were homogenized in three volumes of lysis buffer (10 mM Tris pH 7.4, 100 mM NaCl, 1 mM EDTA, 1 mM EGTA, 1 mM NaF, 20 mM Na4P2O7, 2 mM Na3VO4, 1% Triton-X, 10% glycerol, 0.1% SDS, 0.5% deoxycholate) containing protease cocktail inhibitors (Sigma P2714). The lysate was then and centrifuged at 10,000 g for 10 min to isolate total proteins and the amount of the proteins were determined by Bradford method. The protein samples (50 μg) were then subjected to a 7.5% SDS–polyacrylamide gel electrophoresis and transferred on to nitrocellulose membranes (Bio-Rad, 162-0115). At the end of the transfer, the membrane was blocked by tris-buffered saline containing 0.1% Tween-20 (TBST) and 5% milk powder for 1 h. A polyclonal antibody against human URAT1 (at a dilution of 1:100, Alpha Diagnostic, URAT11-A) or a monoclonal antibody against mouse β-actin (at a dilution of 1:100, Santa Cruz; SC47778) was applied overnight at 4°C. After washing the membrane three times with TBST, a horseradish peroxidase conjugated anti-IgG antibody (Alpha Diagnostic International Inc., 20120) was applied at a dilution of 1:3000 for 1 h at room temperature. The proteins were visualized using ECL detection reagent (Pierce, 32209). The density of bands were analyzed using the NIH Image J 1.41 program (National Institutes of Health) and normalized to β-actin.
Statistical analysis
All values are presented as mean ± SEM. Statistical comparisons were performed by one-way ANOVA, Newman Keuls as a post hoc test. p < 0.05 was considered as significant.
RESULTS
To study the physical activity-induced changes in URAT1 functional capacity and expression, the animals were exhausted once a day for 5 days. The exhaustion times were similar in each exercise day and recorded as 53.2 ± 2.3; 53.0 ± 2.8; 52.6 ± 2.1; 54.3 ± 2.2, and 54.9 ± 1.8 min/day in exercised animals. As seen in , neither this exercise model nor OA-induced hyperuricemia caused significant alterations in MAP, RBF, and GFR. However, exercise resulted in marked glucosuria, proteinuria, and hypoglycemia.
TABLE 1. Studied parameters in rats from sedentary control, exercise, and hyperuricemia groups
Soleus muscle of exhausted rats exhibited a significant difference (p < 0.001) in CS activity (118.21 ± 8.20 μM.g wet wt−1.min−1) with respect to the control group (61.43 ± 4.51 μM.g wet wt−1.min−1). The OA treatment on the other hand, did not have any effect on CS activity (69.05 ± 5.24 μM.g wet wt−1.min−1).
As depicted in , heavy muscle activity increased plasma urate level from 0.81 ± 0.03 to 1.22 ± 0.07 mg/dL (p < 0.01) and the OA treatment had an even more pronounced effect on plasma urate concentration. The urinary urate excretion was significantly higher in both exercised and OA-treated animals compared to sedentary control rats ().
URAT1 activity
As seen in , basal urate content (P0) of isolated tubule segments from all three groups was similar. In the presence of UAT and MRP4 (urate efflux transporters) inhibitors, with addition of 100 μM urate into the incubation medium during 30 min incubation (P1), tubular urate content significantly increased from 7.99 ± 1.85 to 11.76 ± 2.93 μg/mg protein (p < 0.001) in sedentary animals, from 6.06 ± 0.84 to 10.53 ± 1.45 μg/mg protein (p < 0.001) in exercised rats, and from 8.46 ± 1.69 to 14.88 ± 1.99 μg/mg protein (p < 0.001) in hyperuricemic animals. When the known efflux transporters were inhibited, the total urate amount following the incubation period (P1) was not different in control and exercise groups, but increased significantly in tubules of OA-treated animals (p < 0.001).
TABLE 2. PT urate content in different protocols as defined detailed in the Material and Methods
In Protocol 2, in addition to the blockage of effluxers, URAT1 mediated apical and OAT1/OAT3 mediated basolateral urate transports were also inhibited by appropriate inhibitors (morin and cefadroxil, respectively). It was observed that the amount of urate influx through the nonselective transporters into the tubule segments from control, exercised, and OA-treated animals decreased significantly compared to their own P1 values (p < 0.01). However, urate contents of the tubule segments exposed to P2 protocol were similar to each other as well as to their basal values (P0) (). Therefore it is assumed that the urate influx through the unknown transporters (nonselective transporters) can be negligible in our experimental conditions.
As shown in , despite the lack of statistical significance between the results of P3 amongst the groups (), URAT1 mediated influx, which was estimated from the difference of P3–P2, was found to be statistically significant in exercised and OA-treated animals. The URAT1 mediated urate uptake into PT cells was calculated as 1.22 ± 0.22 μg/mg protein in controls, 2.03 ± 0.31 μg/mg protein in exercising rats (p < 0.05) and 2.88 ± 0.23 μg/mg protein in hyperuricemic rats (p < 0.05).
RT-PCR results
Exercise and hyperuricemia-induced changes in mRNA levels of urate transporters are shown in . Despite its elevated functional activity, URAT1 mRNA levels were found to be down-regulated significantly in both exercised and OA-treated animals, compared to those of sedentary controls. The ratio of URAT1 to β-actin mRNA level was decreased from 6.40 ± 2.66 in control to 2.38 ± 0.70 in exercise (p < 0.01) and 1.94 ± 0.83 in hyperuricemia group (p < 0.01).
FIGURE 2. Effect of heavy muscle activity on the mRNA levels of URAT1 in renal cortex from Wistar rats. Total RNA was generated from kidney cortex. RT-PCR against URAT1 and β-actin was performed as described in Materials and Methods. (A) Typical pattern of RT-PCR products. RT-PCR leads to products of the predicted lengths for URAT1 (231 bp), and β-actin (282 bp). (B) Effect of heavy muscle activity on the mRNA levels of URAT1. Amount of URAT1 mRNA signal normalized to the respective signal from β-actin. Data are mean ± SEM of measurements in five independent experiments each group. *p < 0.01 Statistical differences from control animals.
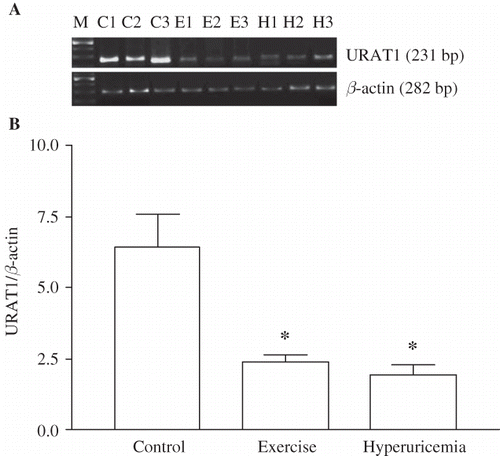
Western blotting analysis
Western blotting analysis revealed that heavy muscle activity and hyperuricemia caused a marked decrease in the renal URAT1 protein expression. The ratio of URAT1 to β-actin protein was significantly lower in exercised (0.90 ± 0.05; p < 0.001) and OA-treated animals (0.89 ± 0.03; p < 0.01) than that of sedentary control rats (1.09 ± 0.02, ).
FIGURE 3. Effect of heavy muscle activity on the protein levels of URAT1 in renal cortex from Wistar rats. Total protein (50 μg) was gathered from kidney cortex. Western blot against URAT1 and β-actin was performed as described in Materials and Methods. (A) Western blot against URAT1 and β-actin. Antibody against URAT1 recognized a band in the range of 60 kDa, and the anti-β-actin antibody recognized a band at 45 kDa. (B) Effect of heavy muscle activity on the expression of URAT1. Amount of URAT1 western blot signal was normalized to the respective signal from β-actin. Data are mean ± SEM of measurements in five independent experiments each group. *p < 0.001 Statistical differences from control animals.
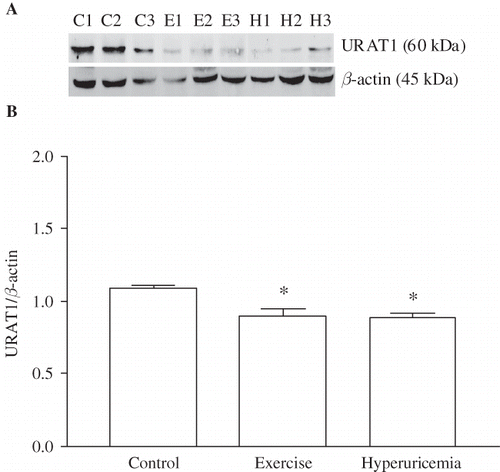
DISCUSSION
This study demonstrated that intense physical activity as shown by increased CS activity in soleus muscle causes some degree of renal dysfunction associated with proteinuria and glucosuria without altering MAP, RBF, and GFR. These results are in agreement with the studies reporting the effects of vigorous muscle activity on kidney functions.Citation3–7,Citation36,Citation37 Despite the presence of several reports indicating the frequent association of hypertension, proteinuria, and reduced GFR with hyperuricemia,Citation12,Citation13,Citation38,Citation39 we were not able to detect such disturbing effects of OA injection in our experimental settings, possibly due to the short period of hyperuricemia (5 days).
As expected, the exercising group displayed hyperuricemia. It has been known that several factors such as excess urate release from the activated muscle, high consumption of purine rich diets and reduced urinary excretion can be involved in the occurrence of hyperuricemiaCitation17 in exercising rats. Since, all animals were fed with standard rat food during the experimental procedures, high intake of purine rich diet cannot be considered as a causative agent of hyperuricemia in our experimental groups. Therefore in accordance with the related literatureCitation8–10 the hyperuricemia seen in our exercised animals can be attributed to the alteration in renal handling of over produced urate by the active muscle.
Renal tubular urate handling has four steps, including filtration, presecretory reabsorption, secretion, and postsecretory reabsorption. Thus, URAT1 which is localized in the apical region of PT cells acts as a urate anion exchanger and is responsible for presecretory and postsecretory reabsorptions seen in step 2 and 4. So, URAT1 mediated transport is accounted for all the luminal urate influx into the PT cells.Citation11–13
In this study, in the isolated PT segments of both exercising and hyperuricemic rats, URAT1 mediated influx was found to be increased significantly in association with elevated urinary urate excretion (, ). Although the exercise-induced enhancement in tubular urate reabsorption has been known, neither in exercisers nor in hyperuricemics, the involvement of URAT1 activity in this reabsorption was previously demonstrated. Therefore our results for the first time demonstrated the involvement of URAT1 mediated urate uptake in both situations.
Despite the presence of several reports indicating heavy muscle activity-induced deteriorations in some tubular reabsorption such as glucosuria and proteinuria, it is interesting that urate reabsorption, which is one of the main reabsorptive functions of PT cells, seems to have remained intact and even elevated in our exercising animals. This result may imply the resistance of URAT1 transporter against exercise-induced tubular injury. However, the preservation of urate reabsorption despite the deterioration of some reabsorptive functions in tubule segments cannot be attributed to one of the beneficial effects of exercise on urate transporter since, the similar enhancement in URAT1 activity was also observed in hyperuricemic rats.
Another interesting data we had is the disparity between the changes of URAT1 activity and its expression. Currently in the literature, there is not any evidence demonstrating the direct effect of heavy muscle activity or hyperuricemia on URAT1 transporter activity or expression. We demonstrated that the elevated URAT1 mediated urate reabsorption was associated with low mRNA and protein expression since both URAT1 mRNA and protein levels were reduced significantly in exercising and hyperuricemia rats. There could be some possible explanations for these seemingly contradictory results: First, URAT1 could be trans-stimulated due to the presence of high lactate as a consequence of heavy exercise. However, this possibility is rather unlikely, since URAT1 capacity was also found to be increased in hyperuricemic animals, in which lactate levels are found to be normal during our preliminary study. Second, the luminal urate load might have a stimulatory effect on URAT1 activity. Interestingly, in an in vitro study it was demonstrated that there was a linear increase of URAT1 mediated urate transport in Xenopus oocytes incubated with the increasing doses of urate.Citation40 In accordance with this study, the elevated urate reabsorption in the tubule segments subjected to high urate load for five days in both exercising and hyperuricemia rats might result in an increase of the URAT1 activity as a consequence of urate-induced adaptive response. As being an anion capable of influencing several intracellular signaling pathways, upon entering into the cells, urate may affect various signaling molecules such as PKC, MAPK, cPLA2 or NF-κBCitation41 and might down-regulate its own expression to prevent further reabsorption.
The duration of this activation/repression or the mechanisms underlying these events are not yet known. It is plausible to assume that the functional activation may be transient and long lasting heavy muscle activity reducing urate transporter may lead to hypouricemia and consequently impairment in urate homeostasis. The exercise intolerance and predisposition to nephrolithiasis in patients with mutated URAT1 support this assumption.Citation27
Although the changes in URAT1 mediated urate transport is not specific to exercise, the urate uptake from the basolateral membrane seems to be influenced significantly from the heavy muscle activity. The amount of basolateral urate influx was calculated from the difference between the total urate uptake and URAT1 mediated urate content (). We demonstrated that, contrary to URAT1 mediated influx increase, OAT1 and OAT3 mediated basolateral urate intake decreased significantly in the tubule of exercising rats (p < 0.05). As depicted in , urate influx via these transporters in hyperuricemia group was not statistically different from those of control rats. This finding suggests the vulnerability of OAT1 and OAT3 transporters to exercise-induced tubular injury; however, further studies are required on the role of these transporters in urate absorption during exercise.
In conclusion, the present data, for the first time, demonstrated the enhanced URAT1 mediated urate uptake associated with its reduced expression in the tubular segments of rats exposed to heavy muscle activity. However, this effect cannot be attributed solely to exercise, as high plasma urate levels seem to play an important role as well. Nevertheless, exercise seems to have a disturbing effect on basolateral urate intake and thus, heavy muscle activity has a potential to disturb urate homeostasis in the body.
Acknowledgments
This study was supported by a research grant from Akdeniz University Research Foundation (No: 2007.03.0122.008).
Declaration of interest: The authors report no conflicts of interest. The authors alone are responsible for the content and writing of the paper.
REFERENCES
- Oner G, Cirrik S. The nephrotoxicity risk in rats subjected to heavy muscle activity. J Sport Sci Med. 2009;8:481–488.
- Cirrik S, Oner G. The effect of heavy muscle activity on renal cytoresistance in rats. Ren Fail. 2009;31:683–689.
- Poortmans JR. Exercise and renal function. Sports Med. 1984;1:125–153.
- Poortmans JR, Vanderstraeten J. Kidney function during exercise in healthy and diseased humans. An update. Sports Med. 1994;18:419–437.
- Mittleman KD, Zambraski EJ. Exercise-induced proteinuria is attenuated by indomethacin. Med Sci Sports Exerc. 1992;24:1069–1074.
- Poortmans JR, Vancalck B. Renal glomerular and tubular impairment during strenuous exercise in young women. Eur J Clin Invest. 1978;8:175–178.
- Poortmans JR, Mathieu N, De Plaen P. Influence of running different distances on renal glomerular and tubular impairment in humans. Eur J Appl Physiol Occup Physiol. 1996;72: 522–527.
- Balsom PD, Seger JY, Sjödin B, Ekblom B. Physiological responses to maximal intensity intermittent exercise. Eur J Appl Physiol Occup Physiol. 1992;65:144–149.
- Stathis CG, Febbraio MA, Carey MF, Snow RJ. Influence of sprint training on human skeletal muscle purine nucleotide metabolism. J Appl Physiol. 1994;76:1802–1809.
- Tullson PC, Bangsbo J, Hellsten Y, Richter EZ. IMP metabolism in human skeletal muscle after exhaustive exercise. J Appl Physiol. 1995;78:146–152.
- Hediger MA, Johnson RJ, Miyazaki H, Endou H. Molecular physiology of urate transport. Physiology (Bethesda). 2005;20:125–133.
- Capasso G, Jaeger P, Robertson WG, Unwin RJ. Uric acid and the kidney: Urate transport, stone disease and progressive renal failure. Curr Pharm. 2005;11:4153–4159.
- Enomoto A, Endou H. Roles of organic anion transporters (OATs) and a urate transporter (URAT1) in the pathophysiology of human disease. Clin Exp Nephrol. 2005;9:195–205.
- Gündüz F, Sentürk UK. The effect of reactive oxidant generation in acute exercise-induced proteinuria in trained and untrained rats. Eur J Appl Physiol. 2003;90(5–6):526–532.
- Ji LL, Leichtweis S. Exercise and oxidative stress: Sources of free radicals and their impact on antioxidant systems. Age. 1997;20:91–106.
- Banerjee AK, Mandal A, Chanda D, Chakraborti S. Oxidant, antioxidant and physical exercise. Mol Cell Biochem. 2003;253(1–2):307–312.
- Choi HK, Mount DB, Reginato AM. Pathogenesis of gout. Ann Intern Med. 2005;143:499–516.
- Mazzali M, Hughes J, Kim YG, Elevated uric acid increases blood pressure in the rat by a novel crystal-independent mechanism. Hypertension. 2001;38:1101–1106.
- Menè P, Punzo G. Uric acid: Bystander or culprit in hypertension and progressive renal disease? J Hypertens. 2008;26:2085–2092.
- Koch M, De Keyser J. Uric acid in multiple sclerosis. Neurol Res. 2006;28:316–319.
- Weisskopf MG, O'Reilly E, Chen H, Schwarzschild MA, Ascherio A. Plasma urate and risk of Parkinson's disease. Am J Epidemiol. 2007;166:561–567.
- Hooper DC, Spitsin S, Kean RB, Uric acid, a natural scavenger of peroxynitrite, in experimental allergic encephalomyelitis and multiple sclerosis. Proc Natl Acad Sci U S A. 1998;95:675–680.
- Kahn AM. Indirect coupling between sodium and urate transport in the proximal tubule. Kidney Int. 1989;36(3):378–384.
- Reem GH, Vanamee P. Effect of sodium lactate on urate clearance in the dalmatian and in the mongrel. Am J Physiol. 1964;207:113–117.
- Enomoto A, Kimura H, Chairoungdua A, Molecular identification of a renal urate anion exchanger that regulates blood urate levels. Nature. 2002;417:447–452.
- Rizwan AN, Burckhardt G. Organic Anion Transporters of the SLC22 Family: Biopharmaceutical, physiological, and pathological roles. Pharm Res. 2007;24:450–470.
- Sperling O. Hereditary renal hypouricemia. Mol Genet Metab. 2006;89:14–18.
- Ohno M, Ka T, Inokuchi T, Effects of exercise and grape juice ingestion in combination on plasma concentrations of purine bases and uridine. Clin Chim Acta. 2008;388:167–172.
- Nguyen MT, Awale S, Tezuka Y, Hypouricemic effects of acacetin and 4,5-o-dicaffeoylquinic acid methyl ester on serum uric acid levels in potassium oxonate-pretreated rats. Biol Pharm Bull. 2005;28:2231–2234.
- Lowry OH, Rosenbrough NJ, Farr AL, Randell RJ. Protein measurement with folin-phenol reagent. J Biol Chem. 1951;193:265–275.
- Newman DJ, Price CP. Renal function and nitrogen metabolites. In: Burtis CA, Ashwood ER, eds. Tietz Textbook of Clinical Chemistry. Philadelphia, PA: WB Saunders Company; 1999:1204–1270.
- Gehrig JJ Jr., Jamison RL, Baylis C, Troy JL, Brenner BM, Jamison RL. Effect of intermittent feeding on renal hemodynamics in conscious rats. Am J Physiol. 1986;250:F566–F572.
- Agarwal R. Rapid microplate method for PAH estimation. Am J Physiol Renal Physiol. 2002;283: F236–F241.
- Srere PA. Citrate synthase. In: Lowenstein JM, ed. Methods in Enzymology. New York: Academic Press; 1969:3–5.
- Vinay P, Gougoux A, Lemieux G. Isolation of a pure suspension of rat proximal tubules. Am J Physiol. 1981;241:F403–F411.
- Poortmans JR, Labilloy D. The influence of work intensity on postexercise proteinuria. Eur J Appl Physiol Occup Physiol. 1988;57:260–263.
- Middlekauff HR, Nitzsche EU, Nguyen AH, Hoh CK, Gibbs GG. Modulation of renal cortical blood flow during static exercise in humans. Circ Res. 1997;80:62–68.
- Nakagawa T, Mazzali M, Kang DH, Hyperuricemia causes glomerular hypertrophy in the rat. Am J Nephrol. 2003;23:2–7.
- Nakagawa T, Mazzali M, Kang DH, Sanchez-Lozada LG, Herrera-Acosta J, Johnson RJ. Uric acid-a uremic toxin?. Blood Purif. 2006;24:67–70.
- Hosoyamada M, Ichida K, Enomoto A, Hosoya T, Endou H. Function and localization of urate transporter 1 in mouse kidney. J Am Soc Nephrol. 2004;15:261–268.
- Han HJ, Lim MJ, Lee YJ, Lee JH, Yang IS, Taub M. Uric acid inhibits renal proximal tubule cell proliferation via at least two signaling pathways involving PKC, MAPK, cPLA2, and NF-kappaB. Am J Physiol Renal Physiol. 2007;292:F373–F381.