Abstract
Increased NAD(P)H oxidase-dependent free radical generation has been proposed to be a mechanism in glycerol-induced acute renal failure (ARF). Previously, we showed a PPARγ-mediated regulation of free radical generation in ARF. In this study, we examined NAD(P)H oxidase-dependent pathology in ARF and its connection with PPARγ using both Sprague–Dawley rats and gp91phox (+/−) mice. Male gp91phox (+/−) or wild type (+/+) mice were distributed into vehicle and ARF group (50% glycerol; 8 mL/kg bw; i.m.). Animals were placed in metabolic cages for 24 hr and were sacrificed under pentobarbital anesthesia. Urine, plasma and kidneys were processed for biochemical and molecular analysis. Glycerol doubled proteinuria in (+/+) mice (68 ± 4 mg/24 hr) but not in (+/−) mice (43 ± 9 mg/24 hr). This was associated with a markedly reduced creatinine excretion in (+/+) mice (Con: 0.6 ± 0.03 & ARF: 0.37 ± 0.02). Basal plasma and urinary NO was higher in (+/−) mice than the (+/+) type while plasma 8-isoprostane level was lower in (+/−) mice (WT: 165 ± 20; KO: 100 ± 15 pg/mL). Glycerol reduced UNOXV in both (+/+) and (+/−) mice although plasma NO was unchanged. Glycerol also doubled 8-isoprostane in (+/+) (363 ± 22 pg/mL) but not in (+/−) mice (152 ± 20 pg/mL) and this was associated with an increased NAD(P)H oxidase activity in the (+/+) mice. In ARF, PPARγ expression was reduced in (+/+) mice but increased in (+/−) mice. PPARγ activity was also reduced in (+/+) mice but was unchanged in (+/−) mice. We conclude that gp91phox contributes to NAD(P)H oxidase-mediated increased free radical generation in ARF and this may be via reduced PPARγ.
Introduction
Glycerol-induced acute renal failure (ARF) is characterized by myoglobinuria, tubular necrosis and enhanced renal vasoconstriction.Citation1,Citation2 Although the mechanism by which glycerol induces ARF is not clear, this phenomenon is a widely recognized experimental model of myoglobinuric nephropathy and the renal vasoconstriction seen in the initial phase of the injury is considered to be an important pathogenic event. Other than tubular necrosis, endothelial damage and vasoconstriction may be important contributors to the ensuing ischemia. Increased production of reactive oxygen species (ROS) has been elucidated in the pathogenesis of glycerol-induced ARF as they are implicated as the mediators of cellular injury after organ ischemia and reperfusion.Citation3,Citation4 In ARF, there are several potential sources of ROS, including the mitochondrial respiratory electron transport chain, xanthine oxidase activation as a result of ischemia/reperfusion, and endothelial- and neutrophil-associated respiratory burst through activation of NAD(P)H oxidase.Citation5 Among these sources NAD(P)H oxidase has drawn much attention because of its abundance and proximity in the vascular system.
NAD(P)H oxidase is a multi-component membrane-bound enzyme complex, found in the plasma membrane as well as in the membrane of phagosome. The enzyme is made up of six subunits including: a Rho guanosine triphosphatase (GTPa), usually Rac1 or Rac2, and five “phox” units: P91phox, p22phox, p40phox, p47phox, and p67phox.Citation6,Citation7 NAD(P)H generates superoxide, a highly-reactive free-radical, by transferring electrons from NAD(P)H inside the cell across the membrane and coupling these to molecular oxygen. Therefore, NAD(P)H oxidase expression, assembly, and localization are important regulatory steps in superoxide production as well as oxidative stress.
Recently, we have demonstrated that increased free radical generation plays a critical role in the pathology of ARF.Citation8 This was further supported by studies showing burst of oxygen free radicals in glycerol-induced ARF that leads to the altered renal vascular reactivity.Citation9 It has been further pointed out that besides superoxide, hydroxyl radical is also involvedCitation10 as scavenging of hydroxyl species offered protection in glycerol-induced ARF.Citation8,Citation11 Although the involvement of oxygen species such as superoxide has been well established, it is not clear how these oxygen species (superoxide and hydroxyl radicals) are generated as an initiator to the pathogenesis of ARF. Furthermore, linking superoxide generation to its most possible sources such as NAD(P)H oxidase has not been investigated extensively.
Peroxisome proliferator-activated receptors (PPARs) are ligand-activated transcription factors that belong to the nuclear receptor super family and consists of three isoforms named PPARα, PPARδ (or β), and PPARγ. PPARγ is expressed in adipose tissue, endothelial cells, and vascular smooth muscle cells (VSMC).Citation12 Because of its critical role in fat metabolism and the clinical efficacy of PPARγ activators in diabetes, the role of PPARγ in vascular function has been studied extensively in diabeticsCitation13 as well as in non-diabeticsCitation14 and convincing evidence emerged that PPARγ activation contributes to vascular function.Citation15 In our earlier studies, we have shown that PPARγ ligands, ciglitazone and GW1929, reduced free radical generation and prevented renal damage in this model of ARF.Citation2,Citation16 These observations raised the possibility that PPARγ ligand mediated protection in ARF may be through reducing transcriptional regulation of NAD(P)H oxidase. This notion is based on recent study showing rosiglitazone, a PPARγ ligand, suppressing vascular NAD(P)H oxidase expression and reducing superoxide production in diabetic mice.Citation17 Similarly, in endothelial cells, it has been shown that PPARγ ligand decreases the expression of NAD(P)H oxidase subunit p22phox and reduces superoxide production directly or indirectly by affecting the synthesis of anti-oxidant agents that are known suppressor of NAD(P)H oxidase activity or free radical generation.Citation18 Therefore, in this study, we explored the involvement of NAD(P)H oxidase in the pathology of glycerol-induced ARF and its connection with PPARγ-mediated transcriptional regulation by using gp91phox knockout (+/−) mice.
Materials and methods
Unless specified otherwise in the text, all chemicals were obtained from Sigma-Aldrich (St Louis, MO) and are of the highest analytical grade. This study was approved by the institutional Animal Care and Use Committee and conforms to the NIH guidelines on animal care and use.
Animals
This study was carried out in both rats (250–300 g, male Sprague–Dawley; Harlan Sprague–Dawley, Houston, TX) and mice [male gp91phox knock-out (+/−) mice; 18–20 g, Jackson Laboratory, Bar Harbor, ME]. The animals were housed under standard conditions of light and dark cycle with free access to food and water. Rats were randomly divided in two groups: Control and ARF: where they received Ciglitazone (9 nmol/kg/day; orally; 21 days), a PPARγ ligand or its vehicle. For mice study gp91phox knockout mice and their littermate wild type were distributed into control and ARF groups without any pre or post treatment.
Induction of acute renal failure
ARF was induced in the experimental animals after 21 days of pretreatment (except mice which did not receive any pretreatment) with ciglitazone or vehicle. Glycerol (50% v/v, 8 mL/kg, i.m.) was administered as a deep intramuscular injection equally distributed to both hind leg. Rats / mice were deprived of food and water for 24 hr after glycerol administration. After injecting glycerol, animals were placed in metabolic cages and 24 hr urine was collected. Blood was collected via cardiac puncture under pentobarbital anesthesia (Sodium Pentobarbital, 40 mg/kg; i.p). Kidneys were processed for subsequent biochemical and molecular biology analysis.
Biochemical analyses
Urinary excretion of nitrite (UNOXV) was determined by fluorometric kit from Cayman Chemicals (Ann Arbor, MI). Urinary protein excretion was determined by a colorimetric kit from Sigma-Aldrich (St Louis, MO) while creatinine excretion was measured by a kit from Cayman Chemicals. Plasma level of 8-isoprostane, an indicator of free radical generation, was determined by EIA using a kit from Cayman Chemicals following manufacturers recommended protocols. PPARγ activity was determined by a TransAm ELISA kit from Active Motif.
NAD(P)H oxidase enzyme activity assay
NAD(P)H oxidase activity was estimated by a modified enzyme activity assay.Citation19 Briefly, kidney tissue were homogenized and centrifuged in HEPES buffer (250 mM, pH 7.4) and protein concentration was measured with Bradford reagent. Tetrazolium salt radical detector (Cayman Chemicals, Ann Arbor, MI), dissolved in HEPES buffer (200 µL) was added to a 96-microwell plate. Equal volumes (20 µL) of protein samples were then added to separate wells and the reaction was initiated by 100 µM NADH and 100 µM NAD(P)H. Absorbance at 450 nm was measured with a plate reader EL808IU (Bio-Tek Instruments Inc, Winoski, VT) every 10 min for 1 hr at room temperature. Parallel experiments were carried out in the presence of apocynin (10−4 M), an inhibitor of NAD(P)H oxidase. Changes in absorbance were then normalized to the protein concentration.
Immunodetection of protein expression
A total of 40 µg of protein from kidney homogenate was electrophoresed on 12% polyacrylamide gels and transferred to PVDF membrane (Amersham Pharmacia, Piscataway, NJ). Blots were probed with antibody raised against NAD(P)H oxidase component p47phox (1:200 dilution) and p22phox (1:200 dilution), eNOS (1:500 dilution), and PPARγ (1:600 dilution) proteins (Santa Cruz Biotechnology, Santa Cruz, CA), followed by addition of respective secondary antibody (1:5000 dilution) after washing off the primary antibodies. Immuno-complexes were visualized using an enhanced chemiluminescence (ECL-Plus) detection system from Amersham Pharmacia. The intensity of the bands was scanned and quantified using personal densitometer SI scanner and ImageQuant analysis software (Molecular Dynamics, Sunnyvale, CA). Same membranes were probed with GAPDH antibody (1:500) as house-keeping protein. Expression of P47phox, PPARγ or eNOS was normalized with GAPDH for equal loading.
Statistical analysis
Results were presented as mean ± SEM. Means were compared between groups and between treatments for significant difference using ANOVA. In all cases p < 0.05 was considered as statistically significant.
Results
PPARγ ligand ciglitazone prevents renal injury and improves renal function
The i.m. injection of glycerol produces marked proteinuria and deterioration in renal function. The 24 hr protein excretion was ∼6 times higher in ARF rats (295 ± 20 mg/24 hr) compared to their controls (45 ± 2 mg/24 hr). In ARF rats, this proteinuria was associated with a 56 ± 12% reduction (Control: 0.122 ± .01 mg/mg protein; ARF 0.068 ± 0.01 mg/mg protein) in creatinine excretion (p < 0.05). PPARγ ligand ciglitazone significantly reduced proteinuria by 49 ± 3% (p < 0.05) and blunted the reduction in creatinine excretion (p < 0.05) in rats treated with glycerol. In ARF rats, there was a significant deterioration (∼3-fold) in Na excretion (data not shown) and this was improved by ciglitazone significantly (p < 0.05). Similarly, BUN level was ∼2-fold higher in ARF rats compare to the control and ciglitazone reduces BUN by 37 ± 3% (p < 0.05).
Effect of ciglitazone on free radical generation and NAD(P)H oxidase activity
Plasma 8-isoprostane level was measured () as an indicator of free radical activity. In ARF rats, 8-isoprostane was significantly higher () compared to the control rats. Ciglitazone pre-treatment attenuated free radical activity by 66 ± 9% (ARF; ARF + CG; p < 0.05). Consequently, increased 8-isoprostane was associated with a significantly higher () NAD(P)H oxidase activity which was reduced in ARF after pre-treatment with ciglitazone.
Effect of ciglitazone on nitric oxide (NO) system
NO is involved in the counter-regulation of the effects of ROS. Therefore, we evaluated the effects of ciglitazone on NO production and NOS activity. shows a 47 ± 8% (p < 0.05) reduction in NO excretion from the kidney of ARF rats compared to the control. This attenuation of NO excretion was associated with a significant reduction in eNOS () protein expression indicating a reduction in endogenous NO production. Ciglitazone significantly increased nitrite excretion in ARF rats by 65 ± 13% without affecting eNOS protein expression.
Effect of ciglitazone on expression of p47phox and p22phox protein of NAD(P)H oxidase
ARF rats exhibit a significantly higher expression of both p47phox () and p22phox () proteins of NAD(P)H oxidase when compared to that of control rats. On the other hand, this increase was attenuated when ARF rats were pretreated with ciglitazone suggesting a role for PPARγ on these proteins expressions.
Renal injury and function in gp91phox knock-out mice
Induction of ARF by glycerol produces marked proteinuria and deterioration in renal function in both wild type and knock-out mice. Surprisingly, the extent of impairment was apparently less in knock-out mice compared to that of wild type. For example, proteinuria was more marked in wild type mice after induction of ARF compare to knockout mice () although there basal protein excretion was similar. Similarly, creatinine excretion was impacted significantly in both strains of mice although reduction was more impacted in wild type mice than the knock-out (). On the contrary, gp91phox knock-out mice exhibit a significantly higher (70 ± 8%) basal NO production than the wild type. Twenty-four hours nitrite excretion was attenuated in an equal manner when ARF was induced in both wild and knock-out mice ().
Figure 4. Urinary excretion of protein (a), Creatinine (b) and Nitrite (c) in 24 hr in the wild type (+/+) and gp91phox knock-out (+/−) mice before and after induction of acute renal failure. Values are mean ± SEM. *p < 0.05 versus (+/+) control. @p < 0.05 versus (+/+) ARF. #p < 0.05 versus (−/−) control.
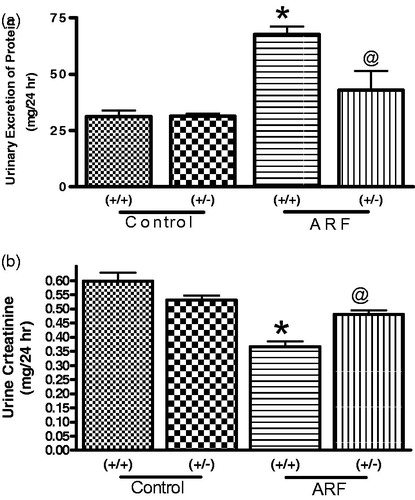
Free radical generation and NAD(P)H oxidase activity in gp91phox knock-out mice
Baseline 8-isoprostane was 39 ± 4% lower in knock-out mice compared to the wild type. Induction of ARF increased plasma 8-isoprostane () in wild type by 84 ± 4% while in knock-out this increase was 52 ± 3%. Similarly, wild type mice have a significantly higher NAD(P)H oxidase activity compared to that of knock-out mice. Induction of ARF in knock-out mice did not alter NAD(P)H oxidase activity significantly although in wild type it was potentiated significantly ().
Figure 5. Plasma 8-Isoprostane (a) and NAD(P)H oxidase (b) activity in the renal homogenates from wild type (+/+) and gp91phox knockout (+/−) mice before and after induction of acute renal failure. Values are mean ± SEM. *p < 0.05 versus (+/+) control. @p < 0.05 versus (+/+) ARF. #p < 0.05 versus (−/−) control.
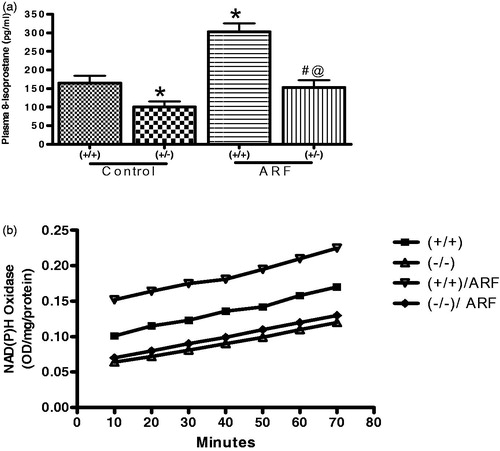
PPARγ protein expression and activity in wild type and knock-out mice
Expression of PPARγ protein was 50% higher in untreated gp91phox knock-out mice compared to that of untreated wild type (). Induction of renal failure significantly reduced PPARγ expression in wild type mice but not in knock-out mice. Similarly, PPARγ activity was higher in untreated knock-out mice compared to their wild type control () and significantly reduced in wild type when ARF was induced ().
Discussion
Generation of ROS is a common feature in many acute and chronic renal diseases and NAD(P)H oxidase is one of the major sources of ROS production in the kidney. The present study was performed to investigate the involvement of NAD(P)H oxidase in the pathology of glycerol-induced ARF and its connection with PPARγ-mediated protection. In glycerol-induced ARF, a widely used model of experimental rhabdomyolysis, a single intramuscular injection of hypertonic solution of glycerol to the hind legs of rodents induces ARF with the similar symptoms observed in human rhabdomyolysis. This model was used in our study and results indicate that there is an increased generation of ROS in glycerol-induced ARF which may be due to observed increased activity of NAD(P)H oxidase enzyme and also increased expression of its subunits. We have also found that ciglitazone, a PPARγ ligand reduces ROS generation, improves renal function and reduces expression and activity of NAD(P)H oxidase and its subunits, p47phox and p22phox. Our observation in ARF rats were corroborated in gp91phox knock-out mice where extent of damage was minimal in knock-out mice which was associated with reduced NAD(P)H oxidase mediated free radical generation.
Accumulating evidence indicate that ROS plays a crucial role in pathogenesis of renal injury in ARF and generation of ROS have been implicated as one of the mechanisms involved. High level of oxidative stress as the result of excessive ROS generation can cause oxidative damage to cellular macromolecules such as membrane lipids, DNA and proteins. In our previous studies, we have reported an increased free radical activity in glycerol-induced ARF.Citation2 In further support of our earlier findings, in this study, glycerol treatment resulted in a marked renal oxidative stress and significantly deranged the renal function as it was evident by high levels of 8-Isoprostane, increased proteinuria and plasma creatinine. Increase generation of ROS has been reported in different models of ARF. Mishra et al. have reported an increased level of oxidants in children with ARF and concluded that production of ROS has a possible role in ARF pathogenesis.Citation20 Similarly, in nephrectomized mice, ROS were exacerbating factors in ischemia/reperfusion-induced ARF.Citation21 There are also several reports implicating ROS as important mediators of cellular injury after organ ischemia and reperfusion.Citation3,Citation4,Citation9 In accordance to these observations, abatement of NAD(P)H function in the mice in this study provided protection against glycerol-induced activation of oxidative stress as evident by reduced free radical generation in ARF mice. Interestingly, these mice tolerated glycerol insult far more effectively than their wild type littermates and renal function was far less impaired than the wild type mice.
NAD(P)H oxidase is one of the major sources of ROS generation in kidney. We confirmed this notion that NAD(P)H is the source of free radical generation in glycerol-induced ARF by demonstrating an increased expression and activity of NAD(P)H oxidase and its components in this model of ARF. In our previous studies, using a DOCA/Salt model,Citation22 we also reported an increased generation of ROS and NAD(P)H oxidase activity which was improved after treatment with clofibrate, a PPARγ ligand. There are several studies reporting changes in expression and activity of NAD(P)H oxidase as source of ROS generation in different models of ARF. Polymorphisms in NAD(P)H oxidase subunit p22phox has been shown to increase the activity of this enzyme and results in increased generation of ROS. In ARF, NAD(P)H oxidase and catalase gene variants are associated with biomarkers of oxidative stress and adverse outcomes.Citation23 Additionally, Nishiyama et al. have reported that aldosterone-induced renal injury is associated with increases in expression of NAD(P)H oxidase components and ROS levels.Citation24 Furthermore, in angiotensin II induced oxidative stress, renal damage has been reported to be via the activation of NAD(P)H oxidase.Citation25 Similarly, in diabetes nephropathy, ROS play a central role in the progression of diabetic micro vascular complications which appear to be generated primarily from mitochondrial sources and via the enzyme, NAD(P)H oxidase.Citation26 Involvement of ROS in chronic renal failure has also been reported and stimulated activity of NAD(P)H has been recognized as the source of ROS in this situation.Citation26 Therefore, a reduction in NAD(P)H oxidase activity or reduced expression of its components either by antioxidantCitation8 or PPARγ ligand ciglitazoneCitation27 would provide protection in glycerol-induced ARF.
In order to further confirm our findings in rats, second phase of this study was designed with gp91phox knock-out mice. Injection of glycerol resulted in severe renal injury in both wild type and knock-out mice as it was evidenced by increased proteinuria and reduced urine creatinine. As expected, impact of inducing ARF was far less in knock-out mice than the wild type. This finding is in accordance with our hypothesis that reduced activity of NAD(P)H oxidase should be protective as it will produce less oxygen free radical. Similarly, NAD(P)H oxidase activity was also less in gp91phox knock-out mice and confirms that NAD(P)H oxidase plays a critical role in the pathogenesis of ARF. Interesting observation from this study was to identify the critical relationship between NOS system and NAD(P)H oxidase system. It has been reported earlier that NO is a key inhibitor of NAD(P)H oxidase and a scavenger of superoxide generated by NAD(P)H oxidase. These are in accordance with our observation where in knock-out mice NO availability was higher compared to the wild type mice. At the same time amount of NO generation was also higher in knock-out mice after induction of ARF. This result confirms the notion that increased NAD(P)H oxidase activity is detrimental to NO system and thus prone to cellular injury.
This study confirms the critical connection between PPARγ and NAD(P)H oxidase in the pathology of ARF and highlighted the interaction between NAD(P)H and PPARγ. In our earlier studies, we proposed PPARγ mediated reduction in ROS generation and apparent protection in ARF.Citation27 We also reported a PPARγ-mediated alteration in NAD(P)H oxidase expression and this was corroborated by other studies where NAD(P)H oxidase components were reported to be a transcriptional target of PPARγ.Citation17 These notions are further authenticated when we observed an increased PPARγ expression in KO mice. This is the testament that PPARγ is sensitive to oxidative stress generated by NAD(P)H oxidase. Obviously, when ARF is induced, it is expected to have more radical generation in wild type but less in knock-out mice and therefore, there will be less PPARγ activation in wild type only. This explanation is conformed when we observed a reduced PPARγ expression and activity in wild type ARF mice and increased PPARγ activity in knock-out mice. This is in accordance with earlier works reporting a DNA microarray study, where reduced PPARγ gene expression has been observed in ARF and this was independently verified by quantitative real time PCR.Citation28,Citation29
These data suggest that ciglitazone prevents ROS-mediated organ injury and improves renal function in glycerol-induced ARF through increased expression of PPARγ protein and reduction in free radical production. We also report that in glycerol-induced ARF, changes in components of NAD(P)H oxidase enzyme are responsible for increased production of ROS.
Declaration of interest
The authors report no conflicts of interests. The authors alone are responsible for the content and writing of this article.
References
- Karam H, Bruneval P, Clozel JP, Loffler BM, Bariety J, Clozel M. Role of endothelin in acute renal failure due to rhabdomyolysis in rats. J Pharmacol Exp Ther. 1995;274(1):481–486
- Newaz MA, Oyekan AO. Vascular responses to endothelin-1, angiotensin-II, and U46619 in glycerol-induced renal failure. J Cardiovasc Pharmacol. 2001;38(4):569–577
- Metnitz P, Fischer M, Bartens C, Steltzer H, Lang T, Druml W. Impact of acute renal failure on antioxidant status in multiple organ failure. Acta Anesthesiol Scand. 2000;44:236–240
- Goligorsky MS, Brodsky SV, Noiri E. NO bioavailability, endothelial dysfunction, and acute renal failure: new insights into pathophysiology. Semin Nephrol. 2004;24:316–323
- Griendling KK, Sorescu D, Ushio-Fukai M. NAD(P)H oxidase: role in cardiovascular biology and disease. Circ Res. 2000;86:494–501
- Chabrashvili T, Tojo A, Onozato ML, et al. Expression and cellular localization of classic NADPH oxidase subunits in the spontaneously hypertensive rat kidney. Hypertension. 2002;39:269–274
- Babior BM. NADPH oxidase. Curr Opin Immunol. 2004;16:42–47
- Yousefipour Z, Hercule H, Oyekan AO, Newaz MA. Antioxidant U74389G improves glycerol-induced acute renal failure without affecting PPARgamma gene. Ren Fail. 2007;29(7):903–910
- Baliga R, Ueda N, Walker P, Shah S. Oxidant mechanisms in toxic acute renal failure. Drug Metab Rev. 1999;31:971–997
- Shah SV, Walker PD. Reactive oxygen metabolites in toxic acute renal failure. Ren Fail. 1992;14(3):363–370
- Zager RA. Rhabdomyolysis and myohemoglobinuric acute renal failure. Kidney Int. 1996;49:314–326
- Marx N, Bourcier T, Sukhova GK, Libby P, Plutzky J. PPAR-activation in human endothelial cells increases plasminogen activator inhibitor type-1 expression: PPARg as a potential ediator in vascular disease. Arterioscler Thromb Vasc Biol. 1999;19:546–551
- Vinik A, Parson H, Ullal J. The role of PPARs in the microvascular dysfunction in diabetes. Vascul Pharmacol. 2006;45(1):54–64
- Panunti B, Fonseca V. Effects of PPAR gamma agonists on cardiovascular function in obese, non-diabetic patients. Vascul Pharmacol. 2006;45(1):29–35
- Blaschke F, Spanheimer R, Khan M, Law RE. Vascular effects of TZDs: new implications. Vascul Pharmacol. 2006;45(1):3–18
- Yousefipour Z, Hercule H, Truong L, Oyekan A, Newaz M. Ciglitazone, a peroxisome proliferator-activated receptor gamma inducer, ameliorates renal preglomerular production and activity of angiotensin II and thromboxane A2 in glycerol-induced acute renal failure. J Pharmacol Exp Ther. 2007;322(2):461–468
- Hwang J, Kleinhenz DJ, Rupnow HL, et al. The PPARgamma ligand, rosiglitazone, reduces vascular oxidative stress and NADPH oxidase expression in diabetic mice. Vascul Pharmacol. 2007;46(6):456–462
- Inoue I, Noji S, Awata T, et al. Bezafibrate has an antioxidant effect: peroxisome proliferator-activated receptor a is associated with Cu2+/Zn2+-superoxide dismutase in the liver. Life Sci. 1998;63:135–144
- Bagi Z, Koller A, Kaley G. PPARg activation, by reducing oxidative stress, increases NO bioavailability in coronary arterioles of mice with Type 2 diabetes. Am J Physiol Heart Circ Physiol. 2004;286:H742–H748
- Mishra OP, Pooniya V, Ali Z, Upadhyay RS, Prasad R. Antioxidant status of children with acute renal failure. Pediatr Nephrol. 2008;23(11):2047–2051
- Yamanobe T, Okada F, Iuchi Y, Onuma K, Tomita Y, Fujii J. Deterioration of ischemia/reperfusion-induced acute renal failure in SOD1-deficient mice. Free Radic Res. 2007;41(2):200–207
- Newaz M, Blanton A, Fidelis P, Oyekan A. NAD(P)H oxidase/nitric oxide interactions in peroxisome proliferator activated receptor (PPAR)alpha-mediated cardiovascular effects. Mutat Res. 2005;579(1–2):163–171
- Perianayagam MC, Orfeas L, Kolyada AY, et al. NADPH oxidase p22phox and catalase gene variants are associated with biomarkers of oxidative stress and adverse outcomes in acute renal failure. J Am Soc Nephrol. 2007;18:255–263
- Nishiyama A, Kusaka T, Kitajima H. Role of aldosterone in oxidative stress and renal injury. Yakugaku Zasshi. 2007;127(9):1331–1337
- Nangaku M, Fujita T. Activation of the renin-angiotensin system and chronic hypoxia of the kidney. Hypertens Res. 2008;31(2):175–184
- Coughlan MT, Cooper ME, Forbes JM. Renal microvascular injury in diabetes: RAGE and redox signaling. Antioxid Redox Signal. 2007;9(3):331–342
- Modlinger PS, Wilcox CS, Aslam S. Nitric oxide, oxidative stress, and progression of chronic renal failure. Semin Nephrol. 2004;24(4):354–365
- Newaz M, Yousefipour Z, Oyekan A. Role of PPAR-gamma on the pathogenesis and vascular changes in glycerol-induced acute renal failure. Pharmacol Res. 2006;54(3):234–240
- Yoshida T, Kurella M, Beato F, et al. Monitoring changes in gene expression in renal ischemia-reperfusion in the rat. Kidney Int. 2002;61(5):1646–1654