Abstract
Background: Acute kidney injury (AKI) is one of the most important causes of mortality and morbidity worldwide. Mesenchymal stem cells (MSCs) can be used for stem cell-based therapy containing AKI. Magnetic resonance imaging (MRI) is an ideal mean for stem cells tracking by labeling with superparamagnetic iron oxide (SPIO). Therefore, using the iron oxide-labeled mesenchymal stem cells (MSC) to treat the AKI and evaluating migration, distribution, and homing of cells by MRI is an ideal method for cell therapy and cell tracking in vivo. Methods: In vitro, the MSCs were labeled with 25 μg/mL for 24 h, and test the labeled efficiency and cells viability. In vitro experiments, magnetic resonance imaging (MRI) measurement of non-labeled and SPIO-labeled MSCs (SPIO-MSCs) was performed in correlation to detectable cells concentrations and detectable time windows. In vivo experiments, MRI evaluation was performed before and after ischemic/reperfusion AKI (N = 14) and intravenous injection of 5 × 105 SPIO-MSCs (N = 10), PBS (N = 6) up to 8 days using a clinical 1.5 T scanner. Signal intensity of kidneys were measured and tested for statistical significance (unpaired Student’s t-test, p < 0.05) in comparison histology (hematoxylin and eosin [H&E], Prussian blue). Results: In vitro, MSCs can be labeled with the SPIO without affecting the viability and labeling efficiency. SPIO-MSCs showed a reduction of signal intensity at T2WI and T2*WI, 5 × 104 cells/mL, SPIO-MSCs were the minimum imaging cells concentration using a 1.5 T MR in vitro. In vivo, SPIO-MSCs administration resulted in a T2*WI signal intensity decrease in renal medulla caused by SPIO-MSCs accumulation in contrast to control groups (p < 0.05) up to day 3 after transplantation, but T2*WI low signal intensity region of the renal medulla revealed an decrease at day 5, and no significant differences between SPIO-MSCs and control animals at day 8. Conclusion: Our data demonstrate that in vitro and in vivo, cell-tracking and monitoring of kidney distribution of intravenous injected SPIO-MSCs after AKI is feasible in MRI at 1.5 T.
Introduction
Acute kidney injury (AKI) is one of the most important causes of mortality and morbidity worldwide.Citation1 In clinical practice, kidney ischemia–reperfusion (I/R) is the most common cause of AKI in both native and transplanted kidneys. AKI alone may increase the risk of death and led to numerous complications, such as seizures, bleeding, coma, or multiorgan failure.Citation2 Furthermore, AKI may often cause end-stage renal disease, which represents a major clinical and socioeconomic problem.Citation3 However, despite the development of regenerative medicine, the treatment of severe AKI remains non-specific and is essentially limited to supportive care, and mortality still remains low. These limitations in the treatment have led to a search for better therapeutic options.
Mesenchymal stem cells (MSCs) are of potential treatment source because of their ability to differentiate into cells of several tissues as well as endothelial, myocardial, renal, or pulmonary epithelial cells, their immunomodulatory capabilities, and their organ protective influence.Citation4 Increasing studies have indicated that the beneficial effects of MSCs are primarily mediated via the paracrine/endocrine action of mediators rather than the direct differentiation and substitution of damaged cells,Citation5,Citation6 and many studies have shown that MSCs can secrete a wide range of chemokines and cytokines that can suppress local immunologic reactions and inhibit fibrosis and apoptosis.Citation7 According to these data, the direct transplant of the cultured MSCs may have beneficial effects on kidney repair by repairing damaged renal structures and improving kidney functions. In vivo detection and tracking of transplanted stem cells in target tissues of a treated patient will become an essential tool in the confirmation of cell delivery and assessment of optimal cell doses. Several previous studies have reported encouraging results in tracking of SPIO-labeled stem cells to determine their biodistribution and migration in different organs like brain, heart, musculature, or liver.Citation8–10 Accordingly, labeling of cells with superparamagnetic iron oxide (SPIO) as an MRI-contrast agent allows for effective, non-invasive, specific and non-toxic in vivo detection and serial monitoring of administered cells in MRI.Citation11
The present study was designed to test the utility of MRI to track after intravenous injection in rabbit with AKI as well as in normal animals, and to investigate their distribution and survival kinetics over time. Our data showed that MRI with SPIO was a sensitive and specific tool to track SPIO-MSCs in vivo, demonstrating that transplanted SPIO-MSCs distribute in distinctive patterns when infused intravenously. We did not detect long-term engraftment of a significant number of cells. In conclusion, MRI with SPIO is a sensitive tool that allows the in vivo definition of cellular distribution, thereby substantially aiding in the preclinical testing and optimization of various cell-based treatment protocols.
Methods
Isolation of rabbit MSC
Two healthy New Zealand white rabbits were purchased from animal experiment center of our university and were housed in a standard animal facility with 12-h on/off light conditions, and allowed standard food and water ad libitum. All procedures were approved by the Institutional Animal Care and Use Committee at our institution. Rabbit MSCs were generated from bone marrow of rabbits and grown in standard culture medium containing fetal calf serum (FCS) by standard procedures as previously reported.Citation12 Phenotype and purity of isolated MSCs were confirmed by their morphology and the identification of mesenchymal stem cells surface makers expression. Details of isolation, induction, and identification of BM-MSCs are provided as Supplementary data.
Magnetic cell labeling and validation of labeling
Cell labeling was conducted by incubation of MSCs with the commercially available SPIO contrast (Resovist; Schering AG, Berlin, Germany) for 24 h with an incubation concentration of 25 μg Fe/mL at 37 °C and 5% CO2 without addition of any transfection agent. After incubation with SPIO, the Prussian blue staining was used for the detection of iron after cell culture day 1, day 3, week 1, week 2, week 3, and week 4. SPIO-MSCs were washed twice with D-Hank’s solution to eliminate extracellular SPIO then fixed in 4% paraformaldehyde for 10 min. After washing with distilled water, the cells were incubated for 30 min with 2% potassium ferrocyanide in 2% hydrochloric acid, and then cells were incubated for 10 min by 0.5% neutral red. Labeling efficiency was determined by manual counting of Prussian blue-stained cells, the average efficiency of MSCs was calculated (mean ± SD).
Assessment of cell viability
Trypan blue staining was used to evaluate the viability of SPIO labeled and unlabeled MSCs and the cells were observed by light microscope. Six fields were selected randomly, the number of live cells were counted and then the percentage was calculated.
In vitro MRI of MSCs
Magnetic resonance imaging of sample tubes containing MSCs suspensions in gelatin were performed at 1.5 T (SIMENS Medical Systems, SONATA, Erlangen, Germany). Cell counting and resuspension of 107 cells in 5 mL FCS a series of cell suspensions were generated containing a different number and a different labeled time of MSCs (SPIO labeled 1 × 104 cells/mL, 5 × 104 cells/mL, 1 × 105cells/mL, 5 × 105 cells/mL, 1 × 106 cells/mL, 5 × 106 cells/mL cells after 1d; SPIO labeled 5 × 104 cells/mL, 5 × 105 cells/mL cells after 1d, 1w, 2w, 3w, 4w) and transferred to Falcon tubes. Sample tubes were filled up to a volume of 0.5 mL with culture medium (FCS) and homogeneously mixed with 0.5 mL of gelatin heated to 37 °C. Samples were cooled quickly to 4 °C to create a solid gel phantom with a homogenous distribution of cells (1 mL). Two types of sample tubes were prepared: (1) gelatin/unlabeled MSCs gel alone (without MSCs as control); (2) gelatin gel containing different numbers of SPIO-labeled MSCs.
MRI data were acquired using a human head coil, slice thickness (SL) = 3.0 mm, matrix size = 512 × 224, field of view (FOV) = 6 cm × 6 cm × 8 cm, the signal intensity were calculated, and the intermediate layer of EP cross section was selected as interesting region (homogeneous signal part), 0.5 mm2 areas were selected. Imaging sequence: (1) Spin Echo (SE):T1-weight imaging (T1WI) TR500 ms, TE7.7 ms; (2) Fast Spin Echo (FSE): T2-weigt imaging (T2WI) TR4000 ms, TE9.5 ms; (3) Gradient Eeho (GRE):T2*-weight imaging (T2*WI) TR800 ms, TE26 ms, FA = 20°. The scan was prepared in cross-sectional orientation to the sample tubes. Mean T1WI, T2W2, and T2*WI signal intensity were calculated, respectively.
Animal model and experiments
Animal examinations were approved by the Institutional Animal Care and Use Committee at our institution. Rabbits (body weight 2.8–3.0 kg) were maintained in the animal facility with free access to standard laboratory diet and water. All surgical and MRI procedures were conducted under intravenous anesthesia using 3% pentobarbital sodium (30 mg/kg). I/R AKI was induced in all animals (N = 15) using a transabdominal approach by clamping left renal artery for 40 min with non-traumatic microvascular clamps followed by visually confirmed blood reperfusion. To compensate for intraoperative fluid losses and to reduce susceptibility artifacts from intraperitoneal air in subsequent MRI examinations, 4 mL of tempered saline was administered intraperitoneally before abdominal closure. Animals were divided into two groups: (1) SPIO-MSCs-treated group (N = 10, injection of 5 × 105 SPIO-MSCs taken from the identical cell population as described and analyzed above, injection volume = 0.5 mL); and (2) untreated control group with medium (PBS) injection alone (N = 6, injection volume = 0.5 mL). After blood reflow, infusion of SPIO-MSCs or PBS was performed by intravenous administration. MRI scans were performed before transplantation, and 0 h (baseline, N = 12: six SPIO-MSCs, six PBS animal), 24, 72, 120, and 192 h after transplantation (N = 12: six SPIO-MSCs, six PBS animal). Kidneys were removed at corresponding time spots to MRI scans (five SPIO-MSCs and three PBS group animals) after transplantation for histological preparation.
Histological ex vivo detection of SPIO-labeled cells
Histological analyses of kidneys were conducted after organ removal, tissue fixation by immersion in 4% buffered formalin, and embedding in paraffin. Sections of the kidneys were examined for injury scoring after standard hematoxylin and eosin (H&E) stain. SPIO labeled MSCs in kidneys were identified with standard Prussian blue stain. All micrographs were taken by a light microscope.
In vivo MRI
Magnetic resonance imaging was performed on the MR scanner, using a custom-made knee coil. MR images were acquired for anatomic depiction of the abdomen using a moderately T2*WI gradient echo sequence (TR/TE = 800 ms/26 ms, FA = 20°, FOV = 60 mm × 48 mm, matrix size = 256 × 256) in transverse and coronal orientation. Signal intensities of kidneys were measured at each time point independently using the standard software implemented on the MR unit.
Statistical analysis
All data are expressed as means ± SD. Comparisons of T2*WI signal intensity between the cell-treated (SPIO-MSCs) and PBS control group were performed using the unpaired Student’s t-test for every time point separately. Statistical analysis was performed by using SPSS 13.0 software for windows (SPSS Inc., Chicago, IL). p Value of < 0.05 was considered to indicate a statistically significant difference.
Results
Morphology and phenotypes of cultured BM-MSCs
After isolation from rabbit’s bone marrow, MSCs were cultured in growth medium. By 14 days of culturing, cell morphology was characterized by spindle-shaped under a light microscope and these MSCs were active proliferation capacity in vitro (). The FACS analysis () showed that these cultured cells were positive for CD44 and CD71 while negative for CD34 (endothelial cell marker) and CD45 (pan-leukocyte marker), the phenotype is similar to that reported by Dominici et al.Citation12
In vitro cell labeling
Prussian blue staining of cultured SPIO-MSCs after incubation for 24 h in the suspension of carboxy dextran-coated SPIO particles (25 μg Fe/mL) revealed an effective intracytoplasmatic uptake of iron oxide nanoparticles (100% of cells) indicated by blue stained vesicles within the cells (), although the visually estimated amount of particles per cell was heterogeneous. Trypan blue stains assays showed that SPIO loading had no adverse effects when compared to unlabeled MSCs (viability for both >90%) ().
Figure 2. Prussian blue staining of SPIO labeled BM-MSCs, and the efficiency is up to 100%. Note: Scale bar, 50 μm.
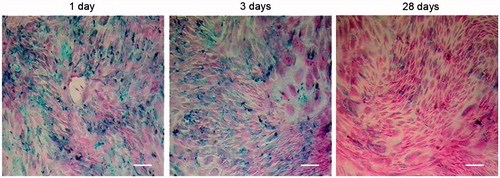
Table 1. The average viability of SPIO-MSCs by Trypan blue staining test.
In vitro MRI of cells
Labeled cells showed obviously lower signal intensity on T2WI and T2*WI. This signal feature was consistent with the presence of SPIO particles inside the cytoplasm. Because of greater T2 shortening effect of SPIO, the T2*WI achieved greater sensitivity to demonstrate SPIO labeled cells than T2WI (Supplementary Figure 1). The effect of the SPIO-labeled MSCs on the signal intensity of the samples in T1WI, T2WI, and T2*WI images are demonstrated (Supplementary Figure 1).
Subsequently, only labeled cells of T2*WI were evaluated and further measured. Signal intensity of SPIO-MSCs (5 × 104 cells/mL, 1 × 105 cells/mL, 5 × 105 cells/mL, 1 × 106 cells/mL, 5 × 106 cells/mL cells after 1 d), was significantly lower than those of control (p < 0.05). However, the signal intensity of 1 × 104 cells/mL MSCs cannot be observed and 5 × 106 cells/mL MSCs can be observed significantly. These suggested that 5 × 104 cells/mL SPIO-MSCs was the minimum imaging cells concentration in 1.5 T MRI (Supplementary Figure 1).
We also compared the signal intensity of different labeled time (1d, 1w, 2w, 3w, 4w) of SPIO-MSCs (5 × 104 cells/mL and 5 × 105 cells/mL cells, respectively). When the cells number was 5 × 104 cell/mL, the difference among different labeled time of the cells was statistically significant (p < 0.05, the labeled time ≤ 2w) (). When the cells number was 5 × 105 cell/mL, the difference among different labeled time of the cells was statistically significant (p < 0.05, the labeled time ≤ 3w) ().
Figure 3. Detectable time point of labeled cells on MRI in vitro (T2*WI). Notes: An increasing signal intensity of labeled cells is suspended in gelatin. Number of labeled cells are suspended in 50 L gelatin. On the T2*WI, there is a significantly lower signal intensity in 5 × 104, cells/mL (A) and 5 × 105 cells/mL SPIO-MSCs compared with unlabeled counterparts, but the signal intensity of week 3 with 5 × 104 cells/mL and week 4 with 5 × 105 cells/mL is almost the same as that of control group.
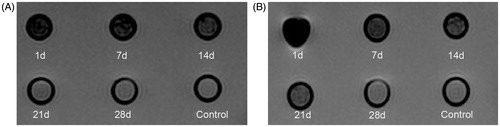
In vivo MRI
T2*WI of the kidneys demonstrated a significant signal loss in renal medulla after intravenous administration of 5 × 105 cells/mL SPIO-MSCs in comparison to PBS control group (). During the following days (after intravenous administration in 1d, 3d, 5d, 8d), the signal intensity of renal cortex and medulla were observed. At no time point, one decrease in T2*WI signal intensity could be observed in the renal cortices and medulla of the PBS control animals and the right renal cortex and medulla of SPIO-MSCs transplanted animals. However, the injured left renal medulla showed a maximal decrease in T2*WI signal intensity at day 3 after transplantation and the T2*WI low signal intensity region of the renal medulla revealed a decrease at day 5 after administration, but no significant differences between SPIO-MSCs and PBS groups at day 8 (). These results demonstrated that 5 × 105 cells/mL SPIO-MSCs transplantation can be detected by MR T2*WI after AKI, and the best detected time is the day 3 after administration. We speculated that the labeled MSCs have migrated into injured left kidney from day 0 to day 8, a part of labeled cells divided rapidly after day 3, and results in the increased T2*WI signal intensity and decreased low signal intensity region area.
Figure 4. Histological detection of labeled SPIO-MSCs in kidneys. Prussian blue and H&E stains of kidney after AKI and application of labeled SPIO-MSCs, all pictures showed a predominant presence of cells in the renal medulla corresponding to the regions of signal loss in MRI. The regions of signal intensity in MRI is the lowest at 72 h after SPIO-MSCs administration. The kidneys show more uptake of iron oxide particles in the renal medulla cells caused by uptake of circulating iron oxide particles, the Scale bar, 50 μm.
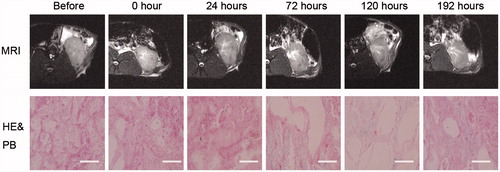
Histology and MRI
Histological analyses (H&E and Prussian blue stains) showed that all groups have been inducted into AKI and severe renal damage (loss of brush border in proximal tubules) (). After application of the magnetically-labeled MSCs showed a predominant location of cells in the renal medulla of injured kidneys (), corresponding to the signal intensity reduction in MRI. In correlation to the lack of a signal intensity loss in the uninjured kidneys of the SPIO-MSCs group, no significant iron-oxide concentration or cells containing iron oxide particles (e.g., macrophages) were found ().
Discussion
In current clinical practice, AKI is a common clinical condition that is associated with high rates of morbidity and mortality, particularly in patients admitted to the ICU.Citation13–15 In addition, emerging evidence indicates that AKI in humans is closely associated with chronic kidney disease if maladaptive repair process is under development;Citation16 however, current therapeutic approaches remain limited. MSCs transplantation is a potential therapy to promote renal tissue regeneration due to their pluripotency and ease of isolation. Using these cells also avoids the ethical ambiguities like using embryonic stem cells.Citation17 Several previous studies also demonstrated that mesenchymal stem cells could recruit to injured tissue and generate high levels of cytokines and growth factorsCitation18,Citation19 and play a repair role. Therefore, it is interest to determine whether MSCs can be tracked in vivo with non-invasive imaging after AKI stem cells treatment, which will provide a tool for the development and testing of optimal AKI-specific treatment protocols.
In the present study, we obtained MSCs using typical methods and cultured these cells for five passages before used in our experiments. Light microscopy and FCS showed that these cells had typical spindle-shaped morphology and express mesenchymal stem cells surface markers.
Magnetic labeling of cells with iron oxide nanoparticles prior to their administration has been demonstrated to be a non-toxic method for non-invasive imaging of cells in MRI, including stem cells.Citation20 In our study, we choose the Resovist, a clinically approved SPIO particle used in our study, has been demonstrated as a nanoparticle with high uptake and long persistence in human MSC throughout a period of several weeks,Citation21 our MSCs can be well labeled with 25 μg/mL SPIO particles without affecting the viability and labeling efficiency.
Previous studies have shown the feasibility of MRI for in vitro imaging and in vivo detection of SPIO-labeled cells using T2*WI gradient echo sequences.Citation22,Citation23 The results of our in vitro experiments confirm that an efficient magnetic labeling of MSC of rabbits (MSCs) is achieved by simple incubation with clinically approved SPIO particles (25 μg/mL). Furthermore we have shown, that labeled MSCs can be detected in vitro in a clinically MR scanner (1.5 T) down to a concentration of approximately 5 × 104 cells/mL (50 cells/L) after labeled 1 day, 1 week and 2 weeks. Additionally, the labeled 5 × 104 cells/mL (500 cells/L) MSCs could be detected in vitro in 1.5 T MR imaging up to 3 weeks.
In our in vivo experiments, we demonstrated that MSCs, which were systemically infused 24 h after kidney injury were selectively recruited to injured left kidneys, which were tracked by MR up to 3 day after transplantation. This recruitment might be associated with enhanced repair of the microvasculature and tubules, improved kidney function, increased survival, promoted proliferation of parenchymal cells, and decreased apoptotic cells, but up to day 8, the SPIO labeled MSCs might result an imaging signal intensity decrease. In contrast, PBS treatment and the uninjured kidney did not have any signal intensity decrease, suggested that MSCs did not migrate to uninjured kidney significantly after transplantation, the histological stain also indicated these results.
In summary, our data demonstrates the potential of MRI for in vitro detection at least for three weeks and the safe non-invasive in vivo monitoring of magnetically-labeled cells for at least eight days in one rabbit model of I/R AKI. The development of the scan protocols on a clinical whole-body MR scanner allows an easy adaptation to application in humans in future.
Conclusion
In conclusion, we conclude that cell-tracking and monitoring of kidney distribution of intravenous injected SPIO-MSCs after AKI is feasible in MRI at 1.5 T in vitro and in vivo.
Its application provides a possibility of monitoring cell-based therapies (e.g., stem cells) in therapeutic treatment models in renal diseases and has the potential to be implemented in clinical cell transplantation protocols in the future.
Key messages
5 × 104 cells/mL SPIO-MSCs may be the minimum imaging cell concentration in 1.5 T MRI.
SPIO-MSCs transplantation can be detected by MR T2*WI after AKI.
There is a significant correlation between low-signal intensity region and the homing of the stem cells.
Supplementary material available online
Supplementary Figure 1.
SUPPLEMENTAL
Download Zip (2 MB)Acknowledgments
We are indebted to Xin Lei and Jing Li for their help with data collection. In addition, we are very grateful to Professor Jun Xie for their laboratory assistance for this manuscript and Ruifeng Liang for help with statistical analysis.
Declaration of interest
The authors declare that they have no competing interests.
This study was supported by grants from the National Natural Science Foundation of China (Grant number: 81371628) and Postdoctoral Science Foundation of China (Grant number: 2013M541206).
References
- Hoste EA, Kellum JA. Incidence, classification, and outcomes of acute kidney injury. Contrib Nephrol. 2007;156:32–38
- Parmar A, Langenberg C, Wan L, May CN, Bellomo R, Bagshaw SM. Epidemiology of septic acute kidney injury. Current Drug Targets. 2009;10(12):1169–1178
- Stengel B, Billon S, Van Dijk PC, et al. Trends in the incidence of renal replacement therapy for end-stage renal disease in Europe, 1990-1999. Nephrol Dial Transplant. 2003;18(9):1824–1833
- Wang Y, Chen X, Cao W, Shi Y. Plasticity of mesenchymal stem cells in immunomodulation: Pathological and therapeutic implications. Nature Immunol. 2014;15(11):1009–1016
- Duffield JS, Park KM, Hsiao LL, et al. Restoration of tubular epithelial cells during repair of the postischemic kidney occurs independently of bone marrow-derived stem cells. J Clin Invest. 2005;115(7):1743–1755
- Duffield JS, Bonventre JV. Kidney tubular epithelium is restored without replacement with bone marrow-derived cells during repair after ischemic injury. Kidney Inter. 2005;68(5):1956–1961
- Liang X, Ding Y, Zhang Y, Tse HF, Lian Q. Paracrine mechanisms of mesenchymal stem cell-based therapy: Current status and perspectives. Cell Transplant. 2014;23(9):1045–1059
- Peldschus K, Kaul M, Lange C, Nolte-Ernsting C, Adam G, Ittrich H. [Magnetic resonance imaging of single SPIO labeled mesenchymal stem cells at 3 Tesla]. RoFo: Fortschritte auf dem Gebiete der Rontgenstrahlen und der Nuklearmedizin. 2007;179(5):473–479
- Bos C, Delmas Y, Desmouliere A, et al. In vivo MR imaging of intravascularly injected magnetically labeled mesenchymal stem cells in rat kidney and liver. Radiology. 2004;233(3):781–789
- Riviere C, Boudghene FP, Gazeau F, et al. Iron oxide nanoparticle-labeled rat smooth muscle cells: Cardiac MR imaging for cell graft monitoring and quantitation. Radiology. 2005;235(3):959–967
- Walczak P, Bulte JW. The role of noninvasive cellular imaging in developing cell-based therapies for neurodegenerative disorders. Neuro-degener Dis. 2007;4(4):306–313
- Zhang R, Li J, Li J, Xie J. Efficient in vitro labeling rabbit bone marrow-derived mesenchymal stem cells with SPIO and differentiating into neural-like cells. Mol Cells. 2014;37(9):650–655
- Cantaluppi V, Biancone L, Romanazzi GM, et al. Macrophage stimulating protein may promote tubular regeneration after acute injury. J Am Soc Nephrol. 2008;19(10):1904–1918
- Nash K, Hafeez A, Hou S. Hospital-acquired renal insufficiency. Am J Kidney Dis. 2002;39(5):930–936
- Mehta RL, Pascual MT, Soroko S, et al. Program to improve care in acute renal D: Spectrum of acute renal failure in the intensive care unit: the PICARD experience. Kidney Inter. 2004;66(4):1613–1621
- Ishani A, Xue JL, Himmelfarb J, et al. Acute kidney injury increases risk of ESRD among elderly. J Am Soc Nephrol. 2009;20(1):223–228
- Yeagy BA, Cherqui S. Kidney repair and stem cells: A complex and controversial process. Pediatr Nephrol. 2011;26(9):1427–1434
- Brown PT, Squire MW, Li WJ. Characterization and evaluation of mesenchymal stem cells derived from human embryonic stem cells and bone marrow. Cell Tissue Res. 2014;358(1):149–164
- Yeatts AB, Choquette DT, Fisher JP. Bioreactors to influence stem cell fate: Augmentation of mesenchymal stem cell signaling pathways via dynamic culture systems. Biochim Biophys Acta. 2013;1830(2):2470–2480
- Frank JA, Miller BR, Arbab AS, et al. Clinically applicable labeling of mammalian and stem cells by combining superparamagnetic iron oxides and transfection agents. Radiology. 2003;228(2):480–487
- Daadi MM, Hu S, Klausner J, et al. Imaging neural stem cell graft-induced structural repair in stroke. Cell Transplant. 2013;22(5):881–892
- Bulte JW, Duncan ID, Frank JA. In vivo magnetic resonance tracking of magnetically labeled cells after transplantation. J Cerebral Blood Flow Metab. 2002;22(8):899–907
- Bulte JW, Ben-Hur T, Miller BR, et al. MR microscopy of magnetically labeled neurospheres transplanted into the Lewis EAE rat brain. Mag Resonance Med. 2003;50(1):201–205