Abstract
Purpose: A combination therapy for combined injury (CI) using a non-specific immunomodulator, synthetic trehalose dicorynomycolate and monophosphoryl lipid A (STDCM-MPL), was evaluated to augment oral antimicrobial agents, levofloxacin (LVX) and amoxicillin (AMX), to eliminate endogenous sepsis and modulate cytokine production.
Materials and methods: Female B6D2F1/J mice received 9.75 Gy cobalt-60 gamma-radiation and wound. Bacteria were isolated and identified in three tissues. Incidence of bacteria and cytokines were compared between treatment groups.
Results: Results demonstrated that the lethal dose for 50% at 30 days (LD50/30) of B6D2F1/J mice was 9.42 Gy. Antimicrobial therapy increased survival in radiation-injured (RI) mice. Combination therapy increased survival after RI and extended survival time but did not increase survival after CI. Sepsis began five days earlier in CI mice than RI mice with Gram-negative species predominating early and Gram-positive species increasing later. LVX plus AMX eliminated sepsis in CI and RI mice. STDCM-MPL eliminated Gram-positive bacteria in CI and most RI mice but not Gram-negative. Treatments significantly modulated 12 cytokines tested, which pertain to wound healing or elimination of infection.
Conclusions: Combination therapy eliminates infection and prolongs survival time but does not assure CI mouse survival, suggesting that additional treatment for proliferative-cell recovery is required.
Introduction
Potential nuclear accidents and terrorist attacks pose serious and credible threats to health and safety throughout the world (CitationBenjamin et al. 2009, CitationSingh et al. 2012b). After a nuclear detonation, depending upon yield and altitude, 40–60% of casualties will sustain combined injury (CI; wounds, burns, or blast injuries in addition to radiation exposure) (CitationAlt et al. 1989, CitationDiCarlo et al. 2011). The adverse biological effects induced by CI act synergistically against the host's survival. Polymicrobial septic infections will accompany injuries from the direct radiation exposure or the CI, especially following lethal doses of γ-radiation (CitationBrook and Ledney 1992, CitationBrook et al. 2004). Polymicrobial infections especially challenge effective therapy because multiple microorganisms have different characteristics, means of defense, and antimicrobial susceptibilities.
Sepsis remains the most common cause of death in North American intensive care units from single-agent infections, despite the extensive development of antimicrobial therapy during the past 60 years, which reduced mortality from 50–75% to 30–50% (CitationParrillo 1991). Effective therapy for polymicrobial sepsis following lethal radiation injury (RI) or CI will be even more complex than for single-agent sepsis (CitationBrook 1988). Eliminating polymicrobial sepsis will require antimicrobial agents, which are effective against both Gram-positive and Gram-negative facultative species, while maintaining the integrity of the beneficial anaerobic intestinal resident bacteria (CitationBrook and Ledney 1992). Administration of antimicrobial agents can eliminate polymicrobial infection and extend survival time but they will not assure survival from cellular injuries in proliferative tissues. To date, there are no known United States Food and Drug Administration (US FDA)-approved non-antimicrobial therapies that will alleviate the injurious sequelae induced by CI and promote recovery of proliferative tissue. Yet, not all antimicrobial agents can be used successfully because of variable susceptibility of bacterial species, particularly among the resident intestinal bacteria (CitationBrook et al. 1988, CitationElliott et al. 1995).
Sublethal irradiation increases susceptibility to common exogenous microorganisms in animal models (CitationBrook et al. 2001) as it does in human patients, who are given whole-body radiotherapy prior to bone marrow transplantation (CitationEngelhard et al. 1986). Whole-body radiation exposure depresses innate and acquired immunity and damages intestinal epithelium. As a result, facultative bacteria can translocate from the intestines to cause sepsis. A perennial question is this: When is the optimal time to initiate antimicrobial therapy after RI or CI? We determined and present here the differential periods of highest risk for sepsis after RI and CI. Clinical experience and studies in relevant animal models of CI remain limited (CitationDiCarlo et al. 2010). Wound closure is delayed (CitationLedney and Elliott 2010) and CI-induced polymicrobial sepsis complicates treatment and increases mortality. Nearly all treatment paradigms for RI have been evaluated with radiation alone and only limited studies are published with the treatment for CI with infection.
In addition to eliminating polymicrobial sepsis, stimulation and recovery of radiation injury to proliferative cells in bone marrow and intestinal epithelium with a non-specific immunomodulator (to stimulate the innate immune component and prevent translocation of intestinal bacteria) will be needed for extended and enhanced survival (CitationMadonna et al. 1991b). Earlier, we have shown that natural trehalose dimycolate (TDM) or synthetic trehalose dicorynomycolate (STDCM), which is a synthetic derivative of TDM with diminished toxicity, when either were administered 1 h after 10.25 Gy γ-radiation, reduced endogenous infection, increased survival, and also enhanced hematopoiesis after 6.5 Gy γ photons (CitationMadonna et al. 1989). TDM and STDCM stimulate macrophage activity (CitationYarkoni et al. 1977, CitationMadonna et al. 1989) and enhance IL-6 in circulation (CitationNishizawa et al. 2007). STDCM increases endogenous splenic colony formation in mixed-field-irradiated mice (CitationStewart et al. 1990) and modulates cytokine responses in bone marrow and spleen of mice exposed to sublethal γ-radiation (CitationPeterson et al. 1994).
Furthermore, STDCM together with antimicrobial agents, including ofloxacin (levofloxacin [LVX] is the biologically active enantiomer of the racemate), extended the survival period of lethally irradiated mice with trauma but STDCM alone did not increase survival as a single agent in CI mice (CitationMadonna et al. 1991a). When ofloxacin was administered at the clinical dosage, it extended the survival period and increased survivors in mice exposed to a lethal dose for 40% of mice for 30 days (LD40/30) of γ-radiation (CitationBrook and Elliott 1991) or a sublethal dose of radiation followed by induced exogenous infection (CitationBrook et al. 1990, CitationBrook and Ledney 1990). However, the clinical dosage is not reliably effective against polymicrobial sepsis in lethally irradiated mice and the efficacy at higher doses of LVX has not been reported in RI or CI mice.
Our long-term goal is to develop a therapeutic regimen for CI that stimulates recovery of hematopoietic and gastrointestinal epithelial tissues as well as obviating sepsis. We investigated the possibility that a combination of systemic oral antimicrobial agents, which are active against Gram-positive and Gram-negative bacteria, together with a non-specific immunomodulator, which stimulates proliferative cells, can eradicate polymicrobial sepsis and extend survival time or enhance 30-day survival in a mouse model of lethal γ-radiation exposure followed by skin wounding. Specifically, we evaluated the combined adjuvant, STDCM-MPL, synthetic trehalose dicorynomycolate and monophosphoryl lipid A, to augment the oral antimicrobial agents, LVX and amoxicillin (AMX). The combined adjuvant, STDCM-MPL, has not been evaluated in RI or CI mice.
We also determined cytokine and chemokine responses to systemic bacterial infection in lethally irradiated mice and CI mice, which were treated with STDCM-MPL and LVX.
Materials and methods
Mice
Ten to 12 week-old female, B6D2F1/J specific pathogen-free mice were purchased from the Jackson Laboratory (Bar Harbor, ME, USA) and housed in an air-conditioned facility accredited by the Association for Assessment and Accreditation of Laboratory Animal Care-International. All mice were kept in rooms with a 12-h light/dark cycle. The mouse holding room was maintained at 21 ± 2°C having 10–15 hourly cycles of fresh air and a relative humidity of 50 ± 10%. Upon arrival, the mice were held in quarantine for 10 days. A microbiological examination of representative samples ensured the absence of Pseudomonas aeruginosa. Mice were maintained in polycarbonate boxes with filter covers (Micro-Isolator®, Lab Products, Inc., Maywood, NJ, USA) on sterilized hardwood chip bedding (Sani-Chips, PJ Murphy Forest Products Corp., Montville, NJ, USA). Mice were provided certified rodent rations (Harlan Teklad Rodent Diet, Harlan Teklad, WI, USA) and acidified water (HCl, pH = 2.5–2.8) ad libitum. At the time of our experiment, mice were 20 weeks (wk) old. Experiments with animals were conducted according to the principles stated in the Guide for the Care and Use of Laboratory Animals (CitationNational Research Council of the National Academy of Sciences 2011) prepared by the Institute of Laboratory Animal Resources, National Research Council, and the research protocol was approved by the Institutional Animal Care and Use Committee of the Armed Forces Radiobiology Research Institute (AFRRI).
Irradiation
Mice were placed in perforated, well ventilated acrylic plastic restrainers, four mice per restrainer, and given 9.75 Gy, the lethal dose for 70% of mice for 30 days (LD70/30), or 0 Gy (non-irradiated control) 60Co γ-radiation at 0.4 Gy/min mid-line tissue from bilaterally positioned 60Co sources (Nordion, Inc., Ottawa, Ontario, Canada) as described previously (CitationLedney et al. 1991, CitationMyska et al. 1997). AFRRI dosimetrists mapped the dose rate in the experimental array in detail according to alanine standards traceable to the National Institute of Standards and Technology (NIST, Gaithersburg, MD, USA), and the National Physical Laboratory (Teddington, Middlesex, UK) (CitationInternational Standardization Organization and ASTM International 2013, CitationNagy 2000). Survival was monitored and recorded two or more times daily.
Skin wounding
The method of skin wounding was performed as previously reported (CitationLedney et al. 1990). One to three days before irradiation, mice were anesthetized by inhalation of methoxyflurane (Anafane®, Medical Development International Ltd., Springvale, Victoria, Australia) and the hair of the dorsal skin was shaved using electric clippers. Within 1 h after irradiation or sham irradiation, mice were anesthetized by methoxyflurane inhalation for approximately 3.5 min prior to wounding. A non-lethal, approximately 15% total-body-surface-area wound was administered approximately 20 mm from the occipital bone and between the scapulae using a sharp 9/16-inch-diameter (14.29 mm) stainless steel punch on a Teflon-covered board cleaned with 70% alcohol before each use. The panniculus carnosus muscle and overlying skin were removed. Mice that received only sham treatments were manipulated identically to other groups but were neither irradiated nor wounded. Mice that received only wounds also were handled identically to other groups but were not irradiated. All mice were given an injection of 0.5 ml sterile isotonic 0.9% sodium chloride intraperitoneally (ip) as fluid therapy immediately after sham handling, irradiation, and/or wounding.
Drug preparation and administration
The synthetic trehalose dicorynomycolate (STDCM) and monophosphoryl lipid A (MPL) Sigma-Aldrich (St. Louis, MO, USA) adjuvant system, which contained 0.5 mg STDCM and 0.5 mg MPL in 44 μl sterile 2% squalene (SQL)-0.2% Tween 80-water per vial (S6322-1VL, Sigma-Aldrich) is a non-specific biological response modifier and was prepared according to the manufacturer's instructions. The reconstituted product (100 μg/0.2 ml) was administered to mice ip 1 h after irradiation and wounding. Vehicle 1 (2% SQL-0.2% Tween 80-water) was prepared by warming sterile 2% Squalene (S3626, Sigma-Aldrich)-0.2% Tween 80-saline emulsion to 40–45°C and mixing by vortex for 2 min.
Levofloxacin (LVX), LEVAQUIN® Oral Solution (25 mg/ml, Ortho-McNeil, Titusville, NJ; NDC 0045-1515-01), is a 4-fluoroquinolone antimicrobial agent. It was diluted to a concentration of 10.4 mg/ml in sterile water to deliver a dose of 90 mg/kg every day (q.d., quaque die) in 0.2 ml given orally (po, per os). Among fluoroquinolones, levofloxacin has improved activity against Gram-positive as well as Gram-negative bacteria (CitationBergogne-Bérézin 2002).
Amoxicillin (AMX), for oral suspension USP (U.S. Pharmacopeia), (250 mg per 5 ml, 150 ml, Novopharm Ltd., Toronto, Ontario, Canada, manufactured for TEVA Pharmaceuticals USA, Sellersville, PA, NDC0093-4155-80), is a semisynthetic aminopenicillin antibiotic. It was diluted to a concentration of 37.5 mg/ml in sterile water to deliver a dose of 300 or 325 mg/kg q.d. to mice in 0.2 ml po. The corresponding vehicle, vehicle 2, for LVX- and AMX-treated mice was sterile water (H2O).
In a preliminary experiment for the purpose of evaluating LVX in irradiated mice, a high conventional dosage regimen of 60 mg/kg twice daily (b.i.d., bis in die) was selected to achieve a higher maximum concentration (Cmax) and area under the curve (AUC) in irradiated mice than in non-irradiated mice or in sublethally irradiated mice given a clinical dosage of 20 mg/kg in previous experiments. Similarly, a dose of 200 mg/kg three times daily (t.i.d., ter in die) was selected to evaluate AMX in irradiated mice. However, those doses given once daily extended survival time but they were not adequate to assure increased survival rates in lethally irradiated mice. Thus, for the treatment experiments reported here, 90–100 mg/kg q.d. for quinolones was selected to achieve a still higher Cmax with a single daily dose that eradicated infection and improved survival. Similarly, a dose of 300–325 mg/kg q.d. for AMX, as an auxiliary component, was selected to achieve a greater time above minimum inhibitory concentration (t > MIC) with a single daily dose to better eradicate infection and improve 30-day survival. A single, high dose daily was used to reduce irritation to irradiated tissues in the mouth and throat by the feeding needle. Oral dosage was used to avoid subcutaneous bleeding following daily skin injections.
To determine the Cmax of the antimicrobial agents, which were achieved in serum, concentrations were determined in six irradiated mice 15 and 30 min after oral administration of LVX or AMX. Six doses of LVX (60 mg/kg po in 0.2 ml) were administered q12h (every 12 hours) for three days starting on day 3 after 9.75 Gy irradiation. On day 6 after irradiation, blood was collected by intracardiac puncture at timed intervals from six mice anesthetized by inhalation of methoxyflurane. Similarly, in CI mice, six doses of LVX were administered q12h for three days beginning 12 h after CI. Blood serum was collected from eight anesthetized CI mice at each time interval after the sixth dose on day 3. To determine the concentration of the antimicrobial agent, serum samples were analyzed by a validated high-performance liquid-chromatography (HPLC) assay with fluorescence detection as described earlier (CitationNix et al. 1997). The lowest detectable concentration of the assay was 0.05 μg/ml LVX. The assay measured the total drug in the sample (free plus bound). Protein-binding of LVX was determined by adding precise quantities to aliquots of 1.2 ml pooled normal mouse serum to concentrations of 8.00, 2.00, and 0.60 μg/ml compared to a six-point standard curve, 0.2–15 μg/ml, and analyzed.
Six doses of AMX (200 mg/kg po in 0.2 ml) were administered q8h for two days starting on day 4 after 9.75-Gy irradiation. On day 6 after irradiation, blood was collected by intracardiac puncture at timed intervals from six mice anesthetized by inhalation of methoxyflurane. To determine the concentration of the antimicrobial agent that was achieved, serum samples were analyzed by a validated HPLC as described earlier (CitationNix et al. 1997). The lowest detectable concentration of the assay was 0.02 μg/ml AMX.
Evaluation of intestinal bacterial translocation
To evaluate facultative bacterial translocation from the intestinal tract, bacteria circulating in the blood are detectable in samples of heart ventricular blood, liver, and/or spleen, particularly when bacteria accumulate in liver and spleen in acutely septic animals (CitationBrook et al. 2004). These samples were collected aseptically from euthanized moribund or recently deceased (< 2 h) mice. The samples were immediately inoculated onto 5% sheep blood agar (SBA), colistin-nalidixic acid in 5% sheep-blood agar (CNA), and xylose-lysine-desoxycholate agar (XLD) media. Cultures were evaluated in the manner as previously described (CitationSingh et al. 2013b). Inoculated specimens were distributed by four-dilution streaking with a sterilized bacteriological loop onto the agar media. SBA and CNA were incubated for 18–24 h at 35°C in 5% CO2 and XLD was incubated at 35°C. SBA is an enriched, non-selective medium, whereas CNA is selective for Gram-positive bacteria and XLD is selective for Gram-negative bacteria. Culture media without observable growth after 24 h were re-incubated for an additional 24 h. Colony morphologies were observed and recorded. Individual colonies were differentiated and selected for subculture using Gram staining. Pure subcultures were identified using a Vitek 2 Compact automated instrument (bioMérieux, Durham, NC, USA). The term, bacterial isolate, is used here to mean a single strain of a bacterial species, which is isolated from a single mouse.
Blood and spleen collection for cytokine analysis
Blood was collected from mice, which were anesthetized by inhalation of methoxyflurane, by cardiac puncture with a 23-gauge needle at 4–5 h, and 1, 3, and 7 days after irradiation (n = 5 mice per group per time point). Blood samples from unirradiated mice served as controls. After collection, blood was transferred to microfuge tubes with gel separators (Capiject® T-MG; Terumo Medical Corp., Elkton, MD, USA), allowed to clot for 30 min, and centrifuged at 1200 g for 10 min. The serum was collected and stored at − 70°C until analyzed.
Immediately following cardiac puncture, the abdomen was opened. The spleen was excised, homogenized promptly, and frozen in liquid nitrogen (the process took 65–70 sec per spleen). The spleens were homogenized for 3 sec in 2 ml of ice-cold phosphate-buffered saline (0.9% sodium chloride) containing 1% bovine serum albumin to which 220 μl of 0.1 M phenylmethanesulfonyl fluoride (P93482 Sigma- Aldrich) in ethanol was added immediately before homogenization to inhibit protease action. Each sample was aliqoted (1 ml per tube into two snap-topped 1.7-ml microfuge tubes) to which 110 μl of 10% 3-[(3-cholamidopropyl)dimethylammonio]-1-propanesulfonate (C3023 Sigma- Aldrich) in phosphate buffered saline had been added at room temperature before sampling began to remove proteins bound to cell membranes, and the two tubes were immersed above the meniscus for 35 sec in liquid nitrogen, then stored on dry ice until stored in a freezer at − 70°C. Prior to analysis for cytokines, the homogenates were thawed on ice, mixed gently by vortex, incubated for 30 min on wet ice (0°C), and then centrifuged for 15 min at 2000 g and 4°C.
Luminex analysis of cytokine, chemokine, and growth factor concentrations
Luminex 200 (Luminex Corp., Austin, TX, USA) was used to detect 12 cytokines, chemokines, and growth factors. Mouse serum and homogenized spleen samples were analyzed for interleukin-1α (IL-1α), IL-1β, IL-6, IL-10, granulocyte-colony-stimulating factor (G-CSF), granulocyte-macrophage colony-stimulating factor (GM-CSF), interferon-γ (IFN-γ), keratinocyte-derived chemokine (KC, now known as CXCL1 [chemokine (C-X-C motif) ligand 1]), monocyte chemotactic protein-1 (MCP-1), macrophage inflammatory protein-1 α (MIP-1α, now known as CCL3 [chemokine (C-C motif) ligand 3]), MIP-1β (now CCL4), and tumor necrosis factor-α (TNF-α). A cytokine analysis kit was custom ordered (M20006245K, Bio-Rad Inc., Hercules, CA, USA) and analysis was performed as described earlier (CitationSingh et al. 2012a). Cytokine quantification was performed using Bio-Plex Manager software, version 6.1 (Bio-Rad Inc.).
Statistical analysis
For survival data, a log-rank test was used to compare survival curves. Fisher's exact test was used to compare survival rates at the end of 30 days, with a Bonferroni correction used to control for type-I error if multiple comparisons were used. For analyses of cytokines, mean values with standard errors (SE, when applicable) were reported. Analysis of variance (ANOVA) was performed to determine if there were significant differences between experimental groups. When significance was indicated, a Tukey's post-hoc test was used to determine where significant differences occurred between the treatment groups. All statistical tests were two-sided with a 5% significance level. Statistical software SPSS version 19 IBM was used for analyses.
Results
Determination of LD50/30 for female B6D2F1/J mice to 60Co γ-radiation by probit analysis
Eight groups of mice received different doses of whole-body 60Co γ-radiation (8.0 Gy: 40 mice, 8.5 Gy: 40 mice, 9.0 Gy: 32 mice, 9.5 Gy: 28 mice, 10.0: 28 mice, 10.5 Gy: 32 mice, 11.0 Gy: 40 mice, 11.5 Gy: 40 mice, and 12.0 Gy: 40 mice) at a dose rate of 0.4 Gy/min. Survival was monitored two or more times daily and survival at 30 days was analyzed by probit analysis. The LD50/30 was found to be 9.42 Gy with 95% confidence interval 9.28–9.58 and the LD70/30 was 9.76 Gy [9.60–9.95] based on probit analysis ().
Figure 1. Probit plot of radiation dose response of female B6D2F1/J mice exposed to 60Co γ-radiation. Groups of mice were given 8.0 (40 mice), 8.5 (40 mice), 9.0 (32 mice), 9.5 (28 mice), 10.0 (28 mice), 10.5 (32 mice), 11.0 (40 mice), 11.5 (40 mice), or 12.0 Gy (40 mice) at 0.4 Gy/min mid-line tissue from bilaterally positioned Co-60 gamma-radiation sources in the AFRRI cobalt high-dose-rate irradiation facility. Survival at 30 days was evaluated by probit analysis.
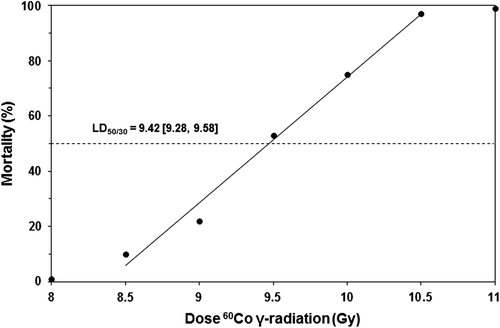
Bacterial translocation in irradiated and CI mice
The range of facultative Gram-negative and Gram-positive bacterial species were isolated and identified by standard microbiological procedures with time after irradiation from heart blood, liver, and/or spleen of a total of 32 euthanized, moribund mice of the 36 irradiated mice (four mice were not suitable for bacterial culture), which were given either 9.50 or 9.75 Gy radiation (n = 18 per dose). Four species of Gram-negative bacteria were isolated from 23 of 32 mice (25 bacterial isolates), Klebsiella oxytoca (13 isolates), Enterobacter cloacae (9), Escherichia coli (2), and Pasteurella trehalosi (1), and seven species of Gram-positive bacteria were isolated from 13 of 32 mice (14 bacterial isolates), Enterococcus faecalis (6), E. gallinarum (2), E. faecium (1), Staphylococcus cohnii (2), S. saprophyticus (1), S. simulans (1), and Lactobacillus sp. (1). Polymicrobial infections were detected in 12 of the 32 mice (37.5%): One Gram-negative and one Gram-positive species were isolated from nine mice, two Gram-negative species were isolated from two mice, and two Gram-positive species were isolated from one mouse. Notably, Gram-negative bacteria predominated beginning on day 9 in moribund mice and declined among the isolated species after day 16, whereas, Gram-positive bacteria appeared on day 10 and became predominant among the isolated species beginning on day 17. We continued to observe such a pattern of translocation in subsequent experiments. No bacteria were isolated from two moribund mice. (Moribundity and bacterial translocation occur randomly over time after irradiation depending upon the susceptibility of individual animals to radiation-induced injuries.) depicts the incidence of composite Gram-negative and Gram-positive bacteria detected vs. time after irradiation, that is, the period of highest risk for sepsis and mortality and the transition from predominantly Gram-negative bacteria to predominantly Gram-positive bacteria.
Figure 2. Incidence of Gram-negative and Gram-positive bacteria detected in ventricular heart blood, liver, and/or spleen of euthanized, moribund B6D2F1/J mice vs. time (days) post-irradiation (9.5 Gy or 9.75 Gy Co-60 gamma-radiation).
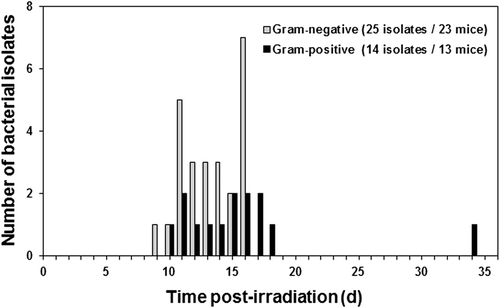
In a separate experiment, incidence of translocated bacteria in CI mice was compared with mice exposed only to radiation (RI, 9.75 Gy) (). Bacteria were isolated and identified from tissues of 41 of 44 RI mice and 45 out of 46 CI mice (no bacteria were detected in 3 RI and 1 CI mice). Polymicrobial infections were detected in 15 RI mice (37%) and 25 CI (56%) mice.
Table I. Bacterial translocation in combined injured (CI) and radiation injured (RI) mice given 9.75 Gy 60Co gamma-photon radiation.
The range of bacterial species isolated during the same period from RI and CI mice are similar and are compared graphically in . In RI mice (), both Gram-negative species (31 isolates), E. coli (30), E. cloacae (1), and Gram-positive species (27 isolates), E. faecalis (13), S. xylosus (13), and S. cohnii (1), were isolated. Similarly, in CI mice () Gram-negative species (47 isolates): E. coli (42), E. cloacae (5) and Gram-positive species (32 isolates): E. faecalis (15), E. gallinarum (8), Staphylococcus xylosus (7), and Lactobacillus sp. (2) were isolated. It should be noted that moribundity and detection of bacteria in CI mice began on day 5 (five days earlier than in RI mice) and continued through day 14 whereas moribundity and detection of bacteria in RI mice began on day 10 and continued through day 22. In both groups, Gram-negative bacteria predominated among the isolated species during the first few days and the proportional cumulative incidence of Gram-negative and Gram-positive bacteria among the isolated species became similar after day 13 in RI mice (19 and 20 isolates, respectively) and after day 8 in CI mice (10 and 11 isolates, respectively) during the periods of mortality.
Figure 3. Incidence of isolates of bacterial species from ventricular heart blood, liver, and/or spleen of lethally irradiated (RI, 9.75 Gy Co-60 gamma-radiation) mice compared to CI (RI + skin wound) mice vs. time (days) post-irradiation. Numbers (e.g., 2/0) above the bars indicate total number of infected mice from which the indicated bacterial species were isolated/number of mice with no detectable bacterial growth on each day.
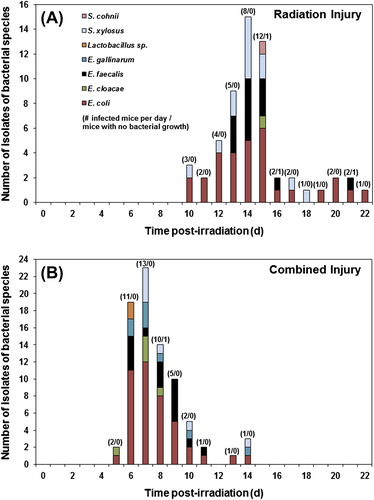
Serum concentrations of antimicrobial agents
Mean peak serum concentration (Cmax) of LVX following 9.75-Gy irradiation was 21.94 ± 0.76 μg/ml ± SEM (standard error of the mean) at 15 min and 17.77 ± 1.56 μg/ml at 30 min compared to 15.91 ± 2.59 μg/ml at 15 min and 14.41 ± 2.20 μg/ml at 30 min in non-irradiated mice and 11.63 ± 1.04 μg/ml at 15 min and 12.95 ± 1.01 μg/ml at 30 min in CI mice. Freely available LVX was measured to be 83.61%, 96.81%, and 97.91% in samples of pooled normal mouse serum, which contained 0.60, 2.00, and 8.00 μg/ml, respectively. The quantity of free drug increased with increasing concentration of total drug. Therefore, bound drug was minimal in the test samples collected at 15 and 30 min. The Cmax of AMX following 9.75-Gy irradiation was 82.91 ± 13.00 μg/ml at 15 min and 91.13 ± 8.86 μg/ml at 30 min compared to 108.00 ± 6.55 μg/ml at 15 min and 89.73 ± 8.78 μg/ml at 30 min in non-irradiated mice (the Cmax of AMX was not determined in CI mice). Protein binding of AMX was approximately 20–30% in mouse serum (CitationMoine et al. 1997).
Effects of antimicrobial therapy on survival after lethal irradiation in mice
Antimicrobial therapy was initiated in groups of mice (n = 20 per group) on day 8 post-irradiation (9.75 Gy), anticipating bacterial translocation, and administered for a duration of 14 days through day 21 (during the period of highest risk for morbidity and sepsis). LVX alone (90 mg/kg q.d. po; 85% survival) and the combination given in the order of LVX first followed by AMX (300 mg/kg q.d. po; 80% survival) improved survival (p ≤ 0.01 and p ≤ 0.05, respectively) compared to control mice administered water (40% survival) (). When AMX was administered alone, it increased survival marginally (50% survival) compared to control group and this difference was not significant. LVX afforded significant protection compared to the AMX group (p ≤ 0.05). Overall, there was no survival benefit demonstrated by combining LVX with AMX. AMX administered either before or after LVX was not beneficial over LVX treatment alone. The time interval between LVX and AMX administration in combination was approximately 20 min as a practical experimental procedure, which allowed absorption of the first drug to reach peak concentration in blood. (After delivery of AMX, the pink suspension was observed to coat the lining of the stomach and intestinal tract.)
Figure 4. Survival of lethally irradiated (RI, 9.75 Gy 60Co gamma-radiation) mice (n = 20) administered oral antimicrobial therapy with LVX (90 mg/kg q.d. po) and AMX (300 mg/kg q.d. po) for 14 days from day 8 through day 21 after irradiation. *p < 0.01 (RI + water vs. RI + LVX), #p < 0.05 (RI + AMX vs. RI + LVX), @p < 0.05 (RI + water vs. RI + LVX AMX).
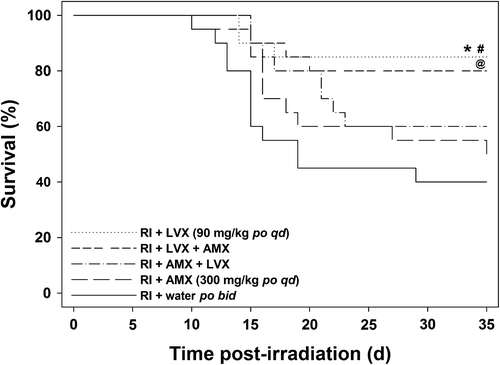
Effects of combined therapy with STDCM-MPL and antimicrobial agents in CI mice
Within 1 h after a lethal dose of radiation (9.75 Gy), groups of mice (n = 20 per group) were wounded or sham wounded. STDCM-MPL or SQL vehicle was administered ip immediately after wounding and fluid therapy. Antimicrobial therapy was initiated in CI mice on day 1 to anticipate bacterial translocation and given daily thereafter through day 21 for a duration of 21 days (during the period of highest risk for morbidity and sepsis). The combination therapy of STDCM-MPL, LVX, and AMX did not increase cumulative 30-day survival in CI mice. Irrespective of treatment provided, all CI mice died by day 18. Nevertheless, the treatment with STDCM-MPL in conjunction with LVX and AMX extended survival time of CI mice by 3–5 days compared to the control group receiving SQL and LVX plus AMX (). The differences were significant on days 8, 9, 10, and 11 post-irradiation. The table associated with demonstrates significant differences between the various paired groups on different days post-irradiation.
Figure 5. Survival of CI (lethally irradiated, 9.75 Gy, then wounded) B6D2F1/J mice (n = 20) administered an immunomodulator (STDCM-MPL, 100 μg, ip, 1 h after irradiation), and antimicrobial agents (LVX, 90 mg/kg q.d. po and AMX, 325 mg/kg q.d. po). Antimicrobial agents were administered for 21 days from day 1 through day 21 after irradiation. *p < 0.01 (CI + STDCM-MPL + LVX + AMX vs. CI + SQL + LVX + AMX).
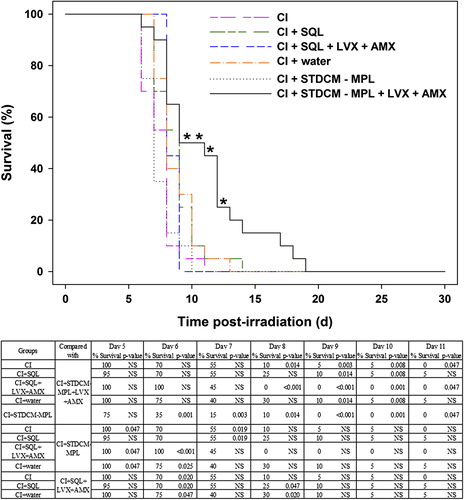
Elimination of bacterial sepsis in RI and CI mice
Bacteria were isolated and identified from tissues of moribund or recently deceased (< 2 h) mice in each treatment group to compare incidence of bacterial species among treatment groups (). The combination of LVX and AMX eliminated bacterial sepsis in 34 out of 34 CI mice and in 19 out of 22 RI mice. E. coli was isolated from one RI mouse that died early on day 8, the first day of antimicrobial therapy, and E. faecalis was isolated from two RI mice on days 25 and 29 (several days after the end of therapy) that were treated with the combination of STDCM-MPL, LVX, and AMX. Also, in 15 CI mice treated with only STDCM-MPL, no Gram-positive bacteria were isolated and in 10 RI mice treated only with STDCM-MPL, Gram-positive species were found in only two mice (E. faecalis in one and E. faecalis and S. xylosus in the other) but STDCM-MPL did not prevent infection by Gram-negative bacteria. As an ancillary observation, Candida parapsilosis (a yeast) was isolated from two CI mice on days 12 and 16 that were treated with STDCM-MPL, LVX, and AMX and from one RI mouse on day 20 that was treated with SQL vehicle, LVX, and AMX.
Induction of cytokines, chemokines, and growth factors after therapy with STDCM-MPL adjuvant and LVX in serum samples and spleen lysate
Based on the encouraging results with antimicrobial agents and an immunomodulator (STDCM-MPL) in RI and CI mice survival and bacterial sepsis studies, we determined the cytokine and chemokine profile in serum samples and spleen tissue of mice receiving various treatments at different time points in CI compared to RI mice to demonstrate whether STDCM-MPL and LVX will modulate early cytokine responses provoked by CI as a means of monitoring recovery and efficacy. We hypothesized that treatment with STDCM-MPL and LVX will promote an early adjustment of the cytokine responses as the treatment reduces severity of infection. The following twelve groups of animals were used in this study: (1) Radiation alone (R, 9.75 Gy); (2) R + wound (W); (3) R + water, 4. R + W + water; (5) R + LVX; (6) R + W + LVX; (7) R + SQL; (8) R + W + SQL; (9) R + STDCM-MPL; (10) R + W + STDCM-MPL; (11) Sham R; (12) Sham R + W. Blood and spleen samples were collected from terminally anesthetized and euthanized animals at various time points (4–5 h, 1, 3, and 7 days after irradiation) for cytokine analysis by multiplex Luminex (n = 5 mice per group per time point). We evaluated serum and spleen lysate for 12 cytokines, chemokines, and growth factors: IL-1α, IL-1β, IL-6, IL-10, G-CSF, GM-CSF, IFN-γ, KC (CXCL1), MCP-1, MIP-1α (CCL3), MIP-1β (CCL4), and TNF-α. Most of the cytokines/chemokines and growth factors in our list are pro-inflammatory agents (CitationCavaillon 2001, CitationScheller et al. 2011). Statistically significant concentrations (pg/ml ± SEM, standard error of the mean) of various cytokines in response to different treatments compared to respective controls are presented in Figure legends (Figures 6A, 6B, 7A and 7B). The results demonstrate that STDCM-MPL stimulated most of the cytokines tested in serum and spleen on day 0 (4–5 h after irradiation) and/or day 1 post-irradiation in RI as well as in CI mice compared to respective control groups receiving SQL in both experimental sets (RI and CI). In particular, stimulation of G-CSF at 4–5 h and day 1 after irradiation and stimulation of MIP-1β at 4–5 h after irradiation was remarkable. Overall, the cytokine stimulation patterns in serum samples and spleen lysates were similar except that the background levels of cytokines in spleen lysate were lower. The levels of some cytokines, specifically G-CSF and KC, were higher in serum samples compared to spleen lysates.
Discussion
Our results demonstrate the complexity of treating polymicrobial infections and cellular injuries in tissues following lethal irradiation alone (LD70/30) and CI. We determined the definitive differential periods of detected sepsis in lethally irradiated and combined injured B6D2F1 mice, which provide the basis for appropriate timing of systemic oral antimicrobial therapy for each condition.
The combined adjuvant, STDCM-MPL, when combined with oral antimicrobial agents LVX and AMX, did extend the survival period of CI mice by 3–5 days. This increase in survival time by a few days is important because such an increase in the survival window may provide extra time to introduce additional therapeutic interventions in CI victims. Further, STDCM-MPL alone prevented Gram-positive infections and, thus, contributed to antimicrobial therapy, perhaps by stimulating the macrophage function (CitationMadonna et al. 1989) and hematopoiesis (CitationMadonna et al. 1989) and by modulating cytokine gene expression in spleen and bone marrow and serum concentration (CitationPeterson et al. 1994, CitationNishizawa et al. 2007). Although LVX, with or without AMX, improved survival time from bacterial sepsis after lethal irradiation, this antimicrobial agent (with or without AMX) at the high dosage used did not increase total 30-day survival of CI mice even though the infecting microorganisms were eliminated. Also, although there was no survival benefit by combining LVX and AMX in RI mice, there was a difference in 30-day survival depending upon the order in which LVX and AMX were administered in combined treatment. Survival was higher when LVX was administered prior to AMX. Perhaps this difference was because the coating of AMX on the lining of the stomach and intestinal tract slowed the absorption of LVX and decreased its Cmax. A thorough pharmacokinetic study might elucidate this observation.
We observed polymicrobial sepsis several days earlier in B6D2F1 CI mice compared directly with mice given only a lethal dose of radiation. In combination with a sublethal dose of radiation, a sublethal wound synergistically increases translocation of intestinal bacteria, leading to higher mortality (CitationYan et al. 1995). Madonna et al. reported that death associated with bacterial translocation occurred 2–4 days after CI in a radiation dose-dependent manner in C3H/HeN mice (CitationMadonna et al. 1991a). While the evidence clearly indicates that trauma increases the likelihood of sepsis by intestinal flora, the mechanism is not well understood and usually controversial. CitationCarter et al. (1990) argued that cutaneous trauma has a causative link to increased gut permeability to bacteria in rats, whereas, others suggested against such a link in humans (CitationSteinberg 2003, CitationWiest and Rath 2003). Instead of changes in gut permeability, these authors have suggested that resultant sepsis may be more related to alterations in the gut-associated lymphoid tissue and its interaction with the rest of the body.
Although the mechanism for increased lethality associated with CI remains not well understood, we hypothesize that not only is early bacterial translocation from the intestinal tract a major contributor to the synergism of CI because sepsis is one of the major causes of mortality in individuals with CI (CitationReid et al. 1955, CitationKiang et al. 2010) but also, the wound induces a remote effect on proliferative cells of the intestinal tract to exacerbate bacterial translocation caused directly by ionizing radiation. On the one hand, sublethal doses of radiation or wounds alone do not elicit bacterial translocation leading to infection, sepsis, and death in mice. On the other hand, lethal RI and CI cause significant, overwhelming bacterial translocation and polymicrobial sepsis, depending upon the quality of radiation (CitationElliott et al. 1995). This also correlates with the onset and severity of neutropenia and thrombocytopenia (CitationMadonna et al. 1991a). In addition, the decreasing concentration of citrulline in plasma as a biomarker of intestinal radiation injury correlated with loss of crypts and increasing dose of γ-photon radiation in irradiated Göttingen minipigs, which developed polymicrobial sepsis (CitationElliott et al. 2014).
Appropriate antimicrobial therapy is critical because use of inappropriate agents exacerbates injury and antimicrobial resistance. Effective therapy should eradicate only the bacteria causing sepsis but not the beneficial anaerobic intestinal microflora promoting colonization resistance against potentially pathogenic bacteria (CitationElliott and Ledney 2012). Adequate antimicrobial therapy will cover a range of both Gram-positive and Gram-negative microorganisms that cause polymicrobial sepsis. Currently, in the event of a radiological exposure, conventional clinical doses of systemic antimicrobial agents would be used as signs of infection develop. However, anticipating the initiation of bacterial translocation and preventing translocation of potentially pathogenic bacteria from the intestinal tract, with higher doses to achieve higher serum concentrations and consequent greater areas under the curve or longer times above minimum inhibitory concentrations for the infecting bacteria, will reduce incidence of bacteremia, sepsis, and multi-organ dysfunction. We have demonstrated definitively here and previously (CitationKiang et al. 2010) that initiation of moribundity and incidence of sepsis occurs several days earlier in CI mice compared with RI mice. We began antimicrobial therapy to cover the periods of highest risk for sepsis that resulted in increased 30-day survival in RI mice and increased the survival period by several days in CI mice. In another study, skin wounding exacerbated not only RI but also radiation-induced anemia, which, together with bone-marrow and intestinal injuries, was mitigated by oral ciprofloxacin treatment (90 mg/kg), another quinolone related to levofloxacin, leading to increased survival (CitationKiang et al. 2012, CitationFukumoto et al. 2013).
It is well known that immunocompromised individuals are at an increased risk for developing infections. Treatment with an immunomodulator stimulates the immune system and boosts immunity to fight infections (CitationAgarwal and Singh 1999). STDCM-MPL is a combined non-specific immunomodulator and it was used to stimulate the immune system of immunocompromised mice (RI and CI). This immunomodulator increased the survival period of CI mice. Thus, we were provoked to study its effects on cytokine responses. Our results demonstrated that STDCM-MPL stimulates most of the pro- and anti-inflammatory cytokines tested in serum samples and spleen lysates on day 0 (4–5 h after irradiation) and/or day one post-irradiation in RI as well as in CI mice compared to respective control groups receiving the vehicle, SQL, in both experimental sets. In particular, stimulation of G-CSF at 4–5 h and day 1 after irradiation and of MIP-1β at 4–5 h after irradiation was remarkable. Overall, the cytokine stimulation patterns in serum samples and spleen lysates were similar. Levels of G-CSF and KC were higher in serum samples compared to spleen lysates.
Various cytokines and growth factors have been investigated as radiation countermeasures in different animal models (CitationSingh et al. 2014a). G-CSF has been found to be effective as a radiomitigator in four different animal models and has been used for treating victims of several radiological accidents (CitationSingh et al. 2014b, Citation2015). This agent is available in the Strategic National Stockpile to be used during any radiological/nuclear emergency (US FDA 2014). Stimulation of G-CSF by STDCM-MPL suggests that this agent has a mitigating role in RI and CI mice. G-CSF stimulated by STDCM-MPL likely contributed to eliminating infecting bacteria, since G-CSF is produced by epithelial cells as well as macrophages and other immune cells and induces proliferation and differentiation of bone marrow progenitor cells into mature neutrophilic granulocytes, which migrate to the wound site and sites of endothelial inflammation. Furthermore, STDCM-MPL stimulated KC (CXCL1) and IL-6 as did several other radiation countermeasures recently evaluated (CitationSingh et al. 2012b, Citation2013a). Chemokines regulate the migration of resident and inflammatory cells during complex interactions of the wound-healing processes (CitationZaja-Milatovic and Richmond 2008). CXCL1, produced by keratinocytes and released by wounding, is one of the principal chemokines, which recruit neutrophils to the wound site. This process is further induced by IL-1 and TNF-α.
Further, in other studies, G-CSF and interleukin-6 have been identified as candidate biomarkers for the radioprotective and radio-mitigating efficacy of CBLB502 (CitationKrivokrysenko et al. 2012). Increasing doses of tocopherol succinate induced higher levels of G-CSF and provided higher levels of protection in irradiated mice (CitationSingh et al. 2013a) suggesting that G-CSF can be used as an efficacy biomarker for this radiation countermeasure also. Administration of a G-CSF antibody exacerbates radiation injury, which further indicates the radioprotective role of G-CSF (CitationSingh et al. 2012c) and administration of a G-CSF antibody completely abrogates the radioprotective efficacy of some radiation countermeasures such as 5-androstenediol (androst-5-ene-3β,17β-diol: 5-AED) (CitationGrace et al. 2012), CBLB502 (CitationKrivokrysenko et al. 2012), δ-tocotrienol (CitationSingh et al. 2014c), γ-tocotrienol (CitationKulkarni et al. 2013), and α-tocopherol succinate (CitationSingh et al. 2010, Citation2011) in mice, suggesting that this growth factor plays an important role in the radioprotective efficacy of these agents. These biomarkers may be useful for accurately predicting radiation injury and the status of recovery during therapy.
In future studies, it would be appropriate to evaluate the hematological and hematopoietic responses to treatment with STDCM-MPL and LVX to compliment the initial evaluation of the early cytokine/chemokine responses and, also, to evaluate cytokine/chemokine responses between days 12–16 in RI mice and 6–10 in CI mice during the course of sepsis in response to combination therapy. In a preliminary study (data not shown) in sublethally irradiated (7 Gy) mice, which were challenged on day 4 by subcutaneous injection of Klebsiella pneumoniae, IL-1α and IL1-β in spleen lysates were highly elevated at 24–36 h and declining at 48 h after bacterial challenge, IL-6 was only slightly elevated between 4 and 48 h, and TNF-α and GM-CSF concentrations were similar to those in saline-challenged controls between 4 and 48 h.
Further, to augment the combination therapy investigated in the present study, we also hypothesize that incorporating 5-AED and δ-tocotrienol regimens 2 h after irradiation to augment STDCM-MPL and antimicrobial therapy would contribute to increased survival time and 30-day survival in irradiated and CI mice. 5-AED increased 30-day survival in irradiated mice, which were subsequently challenged subcutaneously with K. pneumoniae (CitationWhitnall et al. 2000). We recently demonstrated that δ-tocotrienol protected intestinal epithelium and reduced bacterial translocation when injected subcutaneously 24 h before lethal irradiation (CitationLi et al. 2013).
In summary, lethal, whole-body, ionizing radiation exposure together with a skin wound induces polymicrobial sepsis as a result of bacterial translocation from radiation-induced intestinal epithelium injury earlier than after only irradiation alone. Combined injury, therefore, presents a critical challenge to appropriate therapy and ultimate survival. An optimal therapy after radiation exposure would: (1) Enhance resistance to bacterial infections, reduce translocation of bacteria, and eliminate infections leading to increased survival and a longer window of opportunity for additional intervention to military and civilian casualties, and (2) promote prompt recovery of injured proliferative cells and tissues in the intestinal tract and bone marrow. We elucidated and defined that the period of highest risk for sepsis occurs earlier in CI mice compared with RI mice. Thus, antimicrobial therapy needs to be initiated early in the case of CI injury scenarios but somewhat later in RI to anticipate the inception of polymicrobial sepsis. We also demonstrated that a combination of a non-specific immunomodulator with high-dose oral antimicrobial agents can eliminate infections in CI mice and extend survival by a few days, providing extra time for additional interventions required to induce recovery. The regimen used for therapy significantly modulated pro- and anti-inflammatory cytokines and chemokines in RI and CI mice, particularly G-CSF and KC (CXCL1), which contribute to recovery from sepsis and wounding.
Figure 6. (A) Induction of cytokines in serum (IL-1α, IL-1β, IL-6, IL-10, G-CSF, and GM-CSF) by STDCM-MPL and LVX in irradiated and CI mice. Mice were given 9.75 Gy Co-60 gamma-radiation (or sham irradiated) and wounded within 1 h of irradiation. Mice received treatments of STDCM-MPL (100 μg, ip, 1 h after irradiation and wounding) and/or LVX (90 mg/kg q.d., po, days 1–7). Blood samples were collected (n = 5 mice per group per time point) at indicated time points (day 0 [4–5 h], 1, 3, 7 d after irradiation) and serum samples were analyzed by multiplex Luminex assay for cytokines (pg/ml ± SEM). Numbers above bars denote significant difference (p < 0.05) compared to respective controls: 1 signifies a statistically significant difference between groups R and R + W; 2, between R + W + water and R + W + LVX; 3, between R + SQL and R + STDCM-MPL; 4, between R + W + SQL and R + W + STDCM-MPL; 5, between R + STDCM-MPL and R + W + STDCM-MPL; 6, between Sham R and Sham R + W; and 7, between R + LVX and R + W + LVX. The apostrophe (’) indicates significant reduction of cytokine or chemokine production compared to the respective control. (B) Induction of cytokines in serum (IFN-γ, KC, MCP-1, MIP-1α, MIP-1β, and TNF-α) by STDCM-MPL and LVX in irradiated and CI mice. Mice were given 9.75 Gy Co-60 gamma-radiation (or sham irradiated) and wounded within 1 h of irradiation. Mice received treatments of STDCM-MPL (100 μg, ip, 1 h after irradiation and wounding) and/or LVX (90 mg/kg q.d., po, days 1–7). Blood samples were collected (n = 5 mice per group per time point) at indicated time points (day 0 [4–5 h], 1, 3, 7 d after irradiation) and serum samples were analyzed by multiplex Luminex assay for cytokines (pg/ml ± SEM). Numbers above bars denote significant difference (p < 0.05) compared to respective controls: 1 signifies a statistically significant difference between groups R and R + W; 2, between R + W + water and R + W + LVX; 3, between R + SQL and R + STDCM-MPL; 4, between R + W + SQL and R + W + STDCM-MPL; 5, between R + STDCM MPL and R + W + STDCM-MPL; 6, between Sham R and Sham R + W; and 7, between R + LVX and R + W + LVX. The apostrophe (’) indicates significant reduction of cytokine or chemokine production compared to the respective control.
![Figure 6. (A) Induction of cytokines in serum (IL-1α, IL-1β, IL-6, IL-10, G-CSF, and GM-CSF) by STDCM-MPL and LVX in irradiated and CI mice. Mice were given 9.75 Gy Co-60 gamma-radiation (or sham irradiated) and wounded within 1 h of irradiation. Mice received treatments of STDCM-MPL (100 μg, ip, 1 h after irradiation and wounding) and/or LVX (90 mg/kg q.d., po, days 1–7). Blood samples were collected (n = 5 mice per group per time point) at indicated time points (day 0 [4–5 h], 1, 3, 7 d after irradiation) and serum samples were analyzed by multiplex Luminex assay for cytokines (pg/ml ± SEM). Numbers above bars denote significant difference (p < 0.05) compared to respective controls: 1 signifies a statistically significant difference between groups R and R + W; 2, between R + W + water and R + W + LVX; 3, between R + SQL and R + STDCM-MPL; 4, between R + W + SQL and R + W + STDCM-MPL; 5, between R + STDCM-MPL and R + W + STDCM-MPL; 6, between Sham R and Sham R + W; and 7, between R + LVX and R + W + LVX. The apostrophe (’) indicates significant reduction of cytokine or chemokine production compared to the respective control. (B) Induction of cytokines in serum (IFN-γ, KC, MCP-1, MIP-1α, MIP-1β, and TNF-α) by STDCM-MPL and LVX in irradiated and CI mice. Mice were given 9.75 Gy Co-60 gamma-radiation (or sham irradiated) and wounded within 1 h of irradiation. Mice received treatments of STDCM-MPL (100 μg, ip, 1 h after irradiation and wounding) and/or LVX (90 mg/kg q.d., po, days 1–7). Blood samples were collected (n = 5 mice per group per time point) at indicated time points (day 0 [4–5 h], 1, 3, 7 d after irradiation) and serum samples were analyzed by multiplex Luminex assay for cytokines (pg/ml ± SEM). Numbers above bars denote significant difference (p < 0.05) compared to respective controls: 1 signifies a statistically significant difference between groups R and R + W; 2, between R + W + water and R + W + LVX; 3, between R + SQL and R + STDCM-MPL; 4, between R + W + SQL and R + W + STDCM-MPL; 5, between R + STDCM MPL and R + W + STDCM-MPL; 6, between Sham R and Sham R + W; and 7, between R + LVX and R + W + LVX. The apostrophe (’) indicates significant reduction of cytokine or chemokine production compared to the respective control.](/cms/asset/0af47156-a7cb-4b33-b933-b5a76cfdf967/irab_a_1054526_f0006_oc.jpg)
Figure 7. (A) Induction of cytokines in spleen lysates (IL-1α, IL-1β, IL-6, IL-10, G-CSF, and GM-CSF) by STDCM-MPL and LVX in irradiated and CI mice. Mice were given 9.75 Gy Co-60 gamma-radiation (or sham irradiated) and wounded within 1 h of irradiation. Mice received treatments of STDCM-MPL (100 μg, ip, 1 h after irradiation and wounding) and/or LVX (90 mg/kg q.d., po, days 1–7). Spleen lysates were collected (n = 5 mice per group per time point) at indicated time points (day 0 [4–5 h], 1, 3, 7 d after irradiation) and spleen lysate samples were analyzed by multiplex Luminex assay for cytokines (pg/ml ± SEM). Numbers above bars denote significant difference (p < 0.05) compared to respective controls: 1 signifies a statistically significant difference between groups R and R + W; 2, between R + W + water and R + W + LVX; 3, between R + SQL and R + STDCM-MPL; 4, between R + W + SQL and R + W + STDCM-MPL; 5, between R + STDCM-MPL and R + W + STDCM-MPL; 6, between Sham R and Sham R + W. The apostrophe (’) indicates significant reduction of cytokine or chemokine production compared to the respective control. (B) Induction of cytokines in spleen lysates (IFN-γ, KC, MCP-1, MIP-1α, MIP-1β, and TNF-α) by STDCM-MPL and LVX in irradiated and CI mice. Mice were given 9.75 Gy Co-60 gamma-radiation (or sham irradiated) and wounded within 1 h of irradiation. Mice received treatments of STDCM-MPL (100 μg, ip, 1 h after irradiation and wounding) and/or LVX (90 mg/kg q.d., po, days 1–7). Spleen lysates were collected (n = 5 mice per group per time point) at indicated time points (day 0 (4–5 h), 1, 3, 7 d after irradiation) and spleen lysate samples were analyzed by multiplex Luminex assay for cytokines (pg/ml ± SEM). Numbers above bars denote significant difference (p < 0.05) compared to respective controls: 1 signifies a statistically significant difference between groups R and R + W; 2, between R + W + water and R + W + LVX; 3, between R + SQL and R + STDCM-MPL; 4, between R + W + SQL and R + W + STDCM-MPL; 5, between R + STDCM-MPL and R + W + STDCM-MPL; 6, between Sham R and Sham R + W. The apostrophe (’) indicates significant reduction of cytokine or chemokine production compared to the respective control.
![Figure 7. (A) Induction of cytokines in spleen lysates (IL-1α, IL-1β, IL-6, IL-10, G-CSF, and GM-CSF) by STDCM-MPL and LVX in irradiated and CI mice. Mice were given 9.75 Gy Co-60 gamma-radiation (or sham irradiated) and wounded within 1 h of irradiation. Mice received treatments of STDCM-MPL (100 μg, ip, 1 h after irradiation and wounding) and/or LVX (90 mg/kg q.d., po, days 1–7). Spleen lysates were collected (n = 5 mice per group per time point) at indicated time points (day 0 [4–5 h], 1, 3, 7 d after irradiation) and spleen lysate samples were analyzed by multiplex Luminex assay for cytokines (pg/ml ± SEM). Numbers above bars denote significant difference (p < 0.05) compared to respective controls: 1 signifies a statistically significant difference between groups R and R + W; 2, between R + W + water and R + W + LVX; 3, between R + SQL and R + STDCM-MPL; 4, between R + W + SQL and R + W + STDCM-MPL; 5, between R + STDCM-MPL and R + W + STDCM-MPL; 6, between Sham R and Sham R + W. The apostrophe (’) indicates significant reduction of cytokine or chemokine production compared to the respective control. (B) Induction of cytokines in spleen lysates (IFN-γ, KC, MCP-1, MIP-1α, MIP-1β, and TNF-α) by STDCM-MPL and LVX in irradiated and CI mice. Mice were given 9.75 Gy Co-60 gamma-radiation (or sham irradiated) and wounded within 1 h of irradiation. Mice received treatments of STDCM-MPL (100 μg, ip, 1 h after irradiation and wounding) and/or LVX (90 mg/kg q.d., po, days 1–7). Spleen lysates were collected (n = 5 mice per group per time point) at indicated time points (day 0 (4–5 h), 1, 3, 7 d after irradiation) and spleen lysate samples were analyzed by multiplex Luminex assay for cytokines (pg/ml ± SEM). Numbers above bars denote significant difference (p < 0.05) compared to respective controls: 1 signifies a statistically significant difference between groups R and R + W; 2, between R + W + water and R + W + LVX; 3, between R + SQL and R + STDCM-MPL; 4, between R + W + SQL and R + W + STDCM-MPL; 5, between R + STDCM-MPL and R + W + STDCM-MPL; 6, between Sham R and Sham R + W. The apostrophe (’) indicates significant reduction of cytokine or chemokine production compared to the respective control.](/cms/asset/88e807c2-5d49-41ae-a913-e401129a1700/irab_a_1054526_f0007_oc.jpg)
Table II. Bacterial isolation from ventricular heart blood, liver, and/or spleen from euthanized moribund or recently deceased (< 2 h) irradiated (RI) or combined injured (CI) B6D2F1/J mice.
Acknowledgements
The opinions or assertions contained herein are the private views of the authors and are not necessarily those of the Armed Forces Radiobiology Research Institute, the Uniformed Services University of the Health Sciences, or the Department of Defense. We gratefully acknowledge Mr Francis Duffy for editorial help and AFRRI's Veterinary Science Department and Cobalt Radiation Facility for their support during animal experimentation. We appreciate the technical expertise of David P. Nicolau and Christina Sutherland of Hartford Hospital, Hartford, CT, for analyzing the antimicrobial agents in serum samples; and Charles Peloquin and Behrang D. Mahjoub of the University of Florida, Gainesville, FL, for estimating the protein-binding of LVX in mouse serum. We are thankful to Wan Jiao, Joan Smith, Risaku Fukumoto, Min Zhai, True Burns, and Neil Agravante for their technical assistance. This work was supported by Defense Technical Objective MD.29 Medical Countermeasures against Radiation-Induced Enteric Bacterial Sepsis of the U.S. Department of Defense (RAB3AA (RI01) to TBE), AFRRI intramural award (RBB3AI and RBB3AK to TBE).
Declaration of interest The authors report no conflicts of interest. The authors alone are responsible for the content and writing of the paper.
References
- Agarwal SS, Singh VK. 1999. Immunomodulators: A review of studies on Indian medicinal plants and synthetic peptides: Part I: Medicinal plants. Proc Ind Nat Sci Acad B 65:179–204.
- Alt L, Forcino CD, Walker RI. 1989. Nuclear Events and their consequences. In: Zaitchuk R, editor. Textbook of Military Medicine, Part 1, Warefare, Weaponary, and Casualty. Falls Church, Virginia: TMM Publications, Office of the Surgeon General, Department of Army.
- Benjamin GC, McGeary M, McCutchen SR.Assessing medical preparedness to respond to a terrorist nuclear event: Workshop Report. 2009. Washington, DC: The National Academies Press.
- Bergogne-Bérézin E. 2002. Clinical role of protein binding of quinolones. Clin Pharmacokin 41:741–750.
- Brook I. 1988. Use of antibiotics in the management of postirradiation wound infection and sepsis. Radiat Res 115:1–25.
- Brook I, Elliott TB. 1991. Quinolone therapy in the prevention of mortality after irradiation. Radiat Res 128:100–103.
- Brook I, Elliott TB, Harding RA, Bouhaouala SS, Peacock SJ, Ledney GD, Knudson GB. 2001. Susceptibility of irradiated mice to Bacillus anthracis Sterne by the intratracheal route of infection. J Med Microbiol 50:702–711.
- Brook I, Elliott TB, Ledney GD. 1990. Quinolone therapy of Klebsiella pneumoniae sepsis following irradiation: Comparison of pefloxacin, ciprofloxacin, and ofloxacin. Radiat Res 122:215–217.
- Brook I, Elliott TB, Ledney GD, Shoemaker MO, Knudson GB. 2004. Management of postirradiation infection: Lessons learned from animal models. Mil Med 169:194–197.
- Brook I, Ledney GD. 1990. Oral ofloxacin therapy of Pseudomonas aeruginosa sepsis in mice after irradiation. Antimic Agents Chemother 34:1387–1389.
- Brook I, Ledney GD. 1992. Quinolone therapy in the management of infection after irradiation. Crit Rev Microbiol 18:235–246.
- Brook I, Walker RI, MacVittie TJ. 1988. Effect of antimicrobial therapy on bowel flora and bacterial infection in irradiated mice. Int J Radiat Biol Relat Stud Phys Chem Med 53:709–716.
- Carter EA, Tompkins RG, Schiffrin E, Burke JF. 1990. Cutaneous thermal injury alters macromolecular permeability of rat small intestine. Surgery 107:335–341.
- Cavaillon JM. 2001. Pro- versus anti-inflammatory cytokines: Myth or reality. Cell Mol Biol (Noisy-le-grand) 47:695–702.
- DiCarlo AL, Maher C, Hick JL, Hanfling D, Dainiak N, Chao N, Bader JL, Coleman CN, Weinstock DM. 2011. Radiation injury after a nuclear detonation: Medical consequences and the need for scarce resources allocation. Disaster Med Public Health Preparedness 5(Suppl. 1):S32–44.
- DiCarlo AL, Ramakrishnan N, Hatchett RJ. 2010. Radiation combined injury: Overview of NIAID research. Health Phys 98:863–867.
- Elliott TB, Deutz NE, Gulani J, Koch A, Olsen CH, Christensen C, Chappell M, Whitnall MH, Moroni M. 2014. Gastrointestinal acute radiation syndrome in gottingen minipigs (Sus scrofa domestica). Comp Med 64:456–463.
- Elliott TB, Ledney GD. 2012. Therapy for bacterial infections following ionizing radiation injury. In: Mickelson AB, editor. Medical consequences of radiological and nuclear weapons. Fort Detrick, Maryland: Office of The Surgeon General, Department of the Army, USA.
- Elliott TB, Ledney GD, Harding RA, Henderson PL, Gerstenberg HM, Rotruck JR, Verdolin MH, Stille CM, Krieger AG. 1995. Mixed-field neutrons and gamma photons induce different changes in ileal bacteria and correlated sepsis in mice. Int J Radiat Biol 68:311–320.
- Engelhard D, Marks MI, Good RA. 1986. Infections in bone marrow transplant recipients. J Pediatr 108:335–346.
- Fukumoto R, Cary LH, Gorbunov NV, Lombardini ED, Elliott TB, Kiang JG. 2013. Ciprofloxacin modulates cytokine/chemokine profile in serum, improves bone marrow repopulation, and limits apoptosis and autophagy in ileum after whole body ionizing irradiation combined with skin-wound trauma. PLoS One 8:e58389.
- Grace MB, Singh VK, Rhee JG, Jackson WE 3rd, Kao TC, Whitnall MH. 2012. 5-AED enhances survival of irradiated mice in a G-CSF-dependent manner, stimulates innate immune cell function, reduces radiation-induced DNA damage and induces genes that modulate cell cycle progression and apoptosis. J Radiat Res 53:840–853.
- International Standardization Organization and ASTM International. Standard Practice for Use of an Alanine-EPR Dosimetry System, 2013. Geneva, Switzerland. ASTM International, ISO and West Conshohocken (US:PA):7.
- Kiang JG, Garrison BR, Burns TM, Zhai M, Dews IC, Ney PH, Cary LH, Fukumoto R, Elliott TB, Ledney GD. 2012. Wound trauma alters ionizing radiation dose assessment. Cell Biosci 2:20.
- Kiang JG, Jiao W, Cary LH, Mog SR, Elliott TB, Pellmar TC, Ledney GD. 2010. Wound trauma increases radiation-induced mortality by activation of iNOS pathway and elevation of cytokine concentrations and bacterial infection. Radiat Res 173:319–332.
- Krivokrysenko VI, Shakhov AN, Singh VK, Bone F, Kononov Y, Shyshynova I, Cheney A, Maitra RK, Purmal A, Whitnall MH, Gudkov AV, Feinstein E. 2012. Identification of granulocyte colony-stimulating factor and interleukin-6 as candidate biomarkers of CBLB502 efficacy as a medical radiation countermeasure. J Pharmacol Exp Ther 343:497–508.
- Kulkarni S, Singh PK, Ghosh SP, Posarac A, Singh VK. 2013. Granulocyte colony-stimulating factor antibody abrogates radioprotective efficacy of gamma-tocotrienol, a promising radiation countermeasure. Cytokine 62:278–285.
- Ledney GD, Elliott TB. 2010. Combined injury: Factors with potential to impact radiation dose assessments. Health Phys 98:145–152.
- Ledney GD, Madonna GS, Elliott TB, Moore MM, Jackson WE 3rd. 1991. Therapy of infections in mice irradiated in mixed neutron/photon fields and inflicted with wound trauma: A review of current work. Radiat Res 128:S18–28.
- Ledney GD, Madonna GS, McChesney DG, Elliott TB, Brooks I. 1990. Complications of combined injury: Radiation damage and skin wound trauma in mouse models. In: Brown D, Weiss JF, MacVittie TJ, Pillai MV, editors. Treatment of radiation injuries. New York: Plenum Press.
- Li XH, Ghosh SP, Ha CT, Fu D, Elliott TB, Bolduc DL, Villa V, Whitnall MH, Landauer MR, Xiao M. 2013. Delta-tocotrienol protects mice from radiation-induced gastrointestinal injury. Radiat Res 180:649–657.
- Madonna GS, Ledney GD, Elliott TB, Brook I, Ulrich JT, Meyers KR, Patchen ML, Walker RI. 1989. Trehalose dimycolate enhances resistance to infection in neutropenic animals. Infect Immun 57:2495–2501.
- Madonna GS, Ledney GD, Moore MM, Elliott TB, Brook I. 1991a. Treatment of mice with sepsis following irradiation and trauma with antibiotics and synthetic trehalose dicorynomycolate (S-TDCM). J Trauma 31:316–325.
- Madonna GS, Ledney GD, Walker RI. 1991b. Immunomodulators: A key to controlling infections in military personnel? Mil Med 156:578–579.
- Moine P, Sauve C, Vallee E, Bedos J-P, Azoulay-Dupuis E. 1997. In vivo efficacy of cefotaxime and amoxicillin against penicillin-susceptible, penicillin-resistant and penicillin-cephalosporin-resistant strains of Streptococcus pneumoniae in a mouse pneumonia model. Clin Microbiol Infect 3:608–615.
- Myska JC, Adams TL, Bhatt RC, Broom JG, Pitcher CD, Sharpnack FM, St. John TJ, Torres BA, Vavrina G, Gerstenberg HM. 1997. Arrays for use at the cobalt irradiation facility. Technical Report 97-2. Bethesda, MD: Armed Forces Radiobiology Research Institute.
- Nagy V. 2000. Accuracy considerations in EPR dosimetry. Appl Radiat Isotop 52:1039–1050.
- National Research Council of the National Academy of Sciences. 2011. Guide for the Care and Use of Laboratory Animals, Washington, DC: National Academies Press.
- Nishizawa M, Yamamoto H, Imagawa H, Barbier-Chassefiere V, Petit E, Azuma I, Papy-Garcia D. 2007. Efficient syntheses of a series of trehalose dimycolate (TDM)/trehalose dicorynomycolate (TDCM) analogues and their interleukin-6 level enhancement activity in mice sera. J Org Chem 72:1627–1633.
- Nix DE, Wilton JH, Hyatt J, Thomas J, Strenkoski-Nix LC, Forrest A, Schentag JJ. 1997. Pharmacodynamic modeling of the in vivo interaction between cefotaxime and ofloxacin by using serum ultrafiltrate inhibitory titers. Antimicrob Agents Chemother 41:1108–1114.
- Parrillo JE. 1991. Management of septic shock: Present and future. Ann Intern Med 115:491–493.
- Peterson VM, Adamovicz JJ, Elliott TB, Moore MM, Madonna GS, Jackson III WE, Ledney GD, Gause WC. 1994. Gene expression of hematoregulatory cytokines is elevated endogenously after sublethal gamma irradiation and is differentially enhanced by therapeutic administration of biologic response modifiers. J Immunol 153:2321–2330.
- Reid JD, Brooks JW, Ham WT, Evans EI. 1955. The influence of x-radiation on mortality following thermal flash burns: The site of tissue injury as a factor determining the type of invading bacteria. Ann Surg 142:844–850.
- Scheller J, Chalaris A, Schmidt-Arras D, Rose-John S. 2011. The pro- and anti-inflammatory properties of the cytokine interleukin-6. Biochim Biophys Acta 1813:878–888.
- Singh PK, Wise SY, Ducey EJ, Brown DS, Singh VK. 2011. Radioprotective efficacy of tocopherol succinate is mediated through granulocyte-colony stimulating factor. Cytokine 56:411–421.
- Singh VK, Beattie LA, Seed TM. 2013a. Vitamin E: Tocopherols and tocotrienols as potential radiation countermeasures. J Radiat Res 54:973–988.
- Singh VK, Brown DS, Kao TC. 2010. Alpha-tocopherol succinate protects mice from gamma-radiation by induction of granulocyte-colony stimulating factor. Int J Radiat Biol 86:12–21.
- Singh VK, Christensen J, Fatanmi OO, Gille D, Ducey EJ, Wise SY, Karsunky H, Sedello AK. 2012a. Myeloid progenitors: A radiation countermeasure that is effective when initiated days after irradiation. Radiat Res 177:781–791.
- Singh VK, Ducey EJ, Brown DS, Whitnall MH. 2012b. A review of radiation countermeasure work ongoing at the Armed Forces Radiobiology Research Institute. Int J Radiat Biol 88:296–310.
- Singh VK, Fatanmi OO, Singh PK, Whitnall MH. 2012c. Role of radiation-induced granulocyte colony-stimulating factor in recovery from whole body gamma-irradiation. Cytokine 58:406–414.
- Singh VK, Newman VL, Romaine PL, Wise SY, Seed TM. 2014a. Radiation countermeasure agents: An update (2011–2014). Expert Opin Ther Pat 24:1229–1255.
- Singh VK, Newman VL, Seed TM. 2015. Colony-stimulating factors for the treatment of the hematopoietic component of the acute radiation syndrome (H-ARS): A review. Cytokine 71:22–37.
- Singh VK, Romaine PL, Newman VL. 2014b. Biologics as countermeasures for acute radiation syndrome: Where are we now? Expert Opin Biol Ther15:465–471.
- Singh VK, Wise SY, Scott JR, Romaine LP, Newman VL, Fatanmi OO. 2014c. Radioprotective efficacy of delta-tocotrienol, a vitamin E isoform, is mediated through granulocyte colony-stimulating factor. Life Sci 98:113–122.
- Singh VK, Wise SY, Singh PK, Posarac A, Fatanmi OO, Ducey EJ, Bolduc DL, Elliott TB, Seed TM. 2013b. Alpha-tocopherol succinate-mobilized progenitors improve intestinal integrity after whole body irradiation. Int J Radiat Biol 89:334–345.
- Steinberg SM. 2003. Bacterial translocation: What it is and what it is not. Am J Surg 186:301–305.
- Stewart DA, Ledney GD, Madonna GS, Stiefel SM, Moore MM. 1990. Synthetic trehalose dicorynomycolate (S-TDCM) increases hematopoietic cell proliferation in fission neutron (n/g = 1) irradiated mice. Military Medical Laboratory Science 19:208–213.
- U.S. Food and Drug Administration. 2014. About the Pandemic and All-Hazards Preparedness Reauthorization Act of 2013 (PAHPRA), Emergency Preparedness and Response [Online]. Available at: http://www.fda.gov/emergencypreparedness/medicalcountermeasures/ucm346195.htm [Accessed 15 February 2014].
- Whitnall MH, Elliott TB, Harding RA, Inal CE, Landauer MR, Wilhelmsen CL, McKinney L, Miner VL, Jackson III WE, Loria RM, Ledney GD, Seed TM. 2000. Androstenediol stimulates myelopoiesis and enhances resistance to infection in gamma-irradiated mice. Int J Immunopharmacol 22:1–14.
- Wiest R, Rath HC. 2003. Gastrointestinal disorders of the critically ill. Bacterial translocation in the gut. Best Pract Res Clin Gastroenterol 17:397–425.
- Yan Y, Ran X, Wei S. 1995. Changes of immune functions after radiation, burns and combined radiation-burn injury in rats. Chin Med Sci J 10:85–89.
- Yarkoni E, Wang L, Bekierkunst A. 1977. Stimulation of macrophages by cord factor and by heat-killed and living BCG. Infect Immun 16:1–8.
- Zaja-Milatovic S, Richmond A. 2008. CXC chemokines and their receptors: A case for a significant biological role in cutaneous wound healing. Histol Histopathol 23:1399–1407.