Abstract
Polyols are compounds that play various physiological roles in plants. Here we present the identification of four cDNA clones of the model legume Lotus japonicus, encoding proteins of the monosaccharide transporter-like (MST) superfamily that share significant homology with previously characterized polyol transporters (PLTs). One of the transporters, named LjPLT4, was characterized functionally after expression in yeast. Transport assays revealed that LjPLT4 is a xylitol-specific H+-symporter (K m, 0.34 mM). In contrast to the previously characterized homologues, LjPLT4 was unable to transport other polyols, including mannitol, sorbitol, myo-inositol and galactitol, or any of the monosaccharides tested. Interestingly, some monosaccharides, including fructose and xylose, inhibited xylitol uptake, although no significant uptake of these compounds was detected in the LjPLT4 transformed yeast cells, suggesting interactions with the xylitol binding site. Subcellular localization of LjPLT4-eYFP fusions expressed in Arabidopsis leaf epidermal cells indicated that LjPLT4 is localized in the plasma membrane. Real-time RT-PCR revealed that LjPLT4 is expressed in all major plant organs, with maximum transcript accumulation in leaves correlating with maximum xylitol levels there, as determined by GC-MS. Thus, LjPLT4 is the first plasma membrane xylitol-specific H+-symporter to be characterized in plants.
Introduction
Polyols are cyclic or linear, soluble, non-reducing, low molecular weight compounds, derived from the reduction of aldoses and ketoses, which are ubiquitously present in all life forms. In some plant species, polyols are produced directly through photosynthesis and represent major phloem-translocated compounds. The chemically-stable character of these compounds provides several advantages to plants. Polyols can be translocated without being degraded or modified, and serve as carbon and energy source to sustain the growth of non-photosynthetic tissues (Noiraud et al. Citation2001b), and as an efficient carrier improving the mobility of essential microelements like boron (Hu et al. Citation1997, Brown et al. Citation1999). They can accumulate to high (mM) concentrations in the cell cytosol where they cause no damage but instead aid cell survival under stress conditions (Noiraud et al. Citation2001b). By mimicking the structure of water, they act as osmoprotectants against salinity (Everard et al. Citation1994), heat/cold and drought stress (Guo & Oosterhuis Citation1995, Patonnier et al. Citation1999), preventing the inactivation of metabolic processes. They also provide protection against hydroxyl radicals, acting as scavengers of activated oxygen species (Shen et al. Citation1997). In addition to abiotic stresses, polyols are thought to be involved in plant responses in some biotic stresses like pathogen attack (Stoop et al. Citation1996). In polyol accumulating plants, stress conditions result in higher concentrations of these compounds (Guo & Oosterhuis Citation1995, Stoop et al. Citation1996, Patonnier et al. Citation1999, Pommerrenig et al. Citation2007). However, their protective role is also apparent in plant species where polyols are not translocated or are found in trace amounts only. For example, in Arabidopsis thaliana enhanced and specific accumulation of polyols was observed under different stress conditions of growth (Rizhsky et al. Citation2004).
Polyols are produced by the combined activity of sugar phosphate isomerases and reductases and polyol phosphate phosphatases (Rumpho et al. Citation1983) and the most frequently found are the derivatives of glucose (sorbitol or dulcitol) and mannose (mannitol). The first information about polyol transport came from studies of Apium graveolens, a mannitol-synthesizing plant in which mannitol is actively loaded in the phloem by co-transport with protons, which led to the identification and characterization of the first mannitol transporter (AgMaT1) (Noiraud et al. Citation2001a). Since then, cDNAs encoding polyol transporters (PLTs) have been cloned from Malus domestica (MdSOT1, MdSOT2, MdSOT3, MdSOT4 and MdSOT5) (Watari et al. Citation2004), Apium graveolens (AgMAT2) (Juchaux-Cachau et al. Citation2007), Prunus cerasus (PcSOT1 and PcSOT2) (Gao et al. Citation2003), Plantago major (PmPLT1 and PmPLT2) (Ramsperger-Gleixner et al. Citation2004) and Olea europaea (OeMaT1) (Conde et al. Citation2007), plants that translocate polyols in their phloem, and the encoded polypeptides have been extensively characterized. Interestingly, several PLT-like genes have been identified in the genomes of plants that do not translocate polyols in their phloem, including A. thaliana, where six PLT-like transporters have been identified, including AtPLT5, which has been characterized biochemically as a broad-spectrum H+-symporter for linear and cyclic polyols as well as hexoses and pentoses (Klepek et al. Citation2005, Reinders et al. Citation2005). AtPMT1 and AtPMT2 have been characterized recently as monosaccharide transporters with broad substrate range but a preference for fructose and xylitol (Klepek et al. Citation2010). Recent studies reveal that the PLT-encoding genes exhibit tissue- and/or developmental stage-specific regulation (Noiraud et al. Citation2001a, Gao et al. Citation2003, Ramsperger-Gleixner et al. Citation2004, Watari et al. Citation2004) and that their expression is induced in response to stress conditions such as salinity (Conde et al. Citation2007, Pommerrenig et al. Citation2007), mechanical wounding and insect feeding (Reinders et al. Citation2005). Moreover, limited data suggest that these genes are feedback-regulated by the substrates of the encoded transporters (Conde et al. Citation2007). All presently characterized polyol transporters are members of the sugar transporter subfamily of the major facilitator superfamily (MFS) (Marger & Saier Citation1993), however they are not closely related to known sucrose, hexose and myo-inositol transporters (Gao et al. Citation2003). PLTs are integral membrane proteins with hydropathy profiles suggesting 12 membrane-spanning domains, a common characteristic of all members of the MFS. Consensus sequences of the sugar transporter subfamily (Griffith et al. Citation1992) are also generally conserved in polyol transporters. Apart from clues obtained from transcript accumulation data, little is known of the precise physiological role of PLT polyol transporters. Recently, it has been shown that their expression is linked to enhanced resistance of plants to both abiotic (Pommerrenig et al. Citation2007) and biotic stress (Juchaux-Cachau et al. Citation2007).
Here, we report the identification and characterization of four cDNA clones encoding members of the PLT family in the model legume L. japonicus. One of these transporters, LjPLT4 was characterized biochemically following heterologous expression in yeast. In addition, transcript levels of LjPLT4 in various plant organs were quantified by qRT-PCR and related to polyol levels, measured by GC-MS. Finally, data on the subcellular localization of LjPLT4-eYFP polypeptide in plant cells was obtained. Taken together, the results presented below provide important new insights into the substrate specificity and affinity, regulation, location, and physiological role of LjPLT4 in L. japonicus.
Materials and methods
Strains and growth conditions
Saccharomyces cerevisiae (RS453 strain) was grown in synthetic complete liquid medium (SC) supplemented with 2% (w/v) glucose. Yeast cells were transformed using standard lithium acetate methodology.
L. japonicus (Gifu B-129) seeds were kindly provided by Dr Jens Stougaard (University of Aarhus, Denmark). The plants were grown in a controlled environment (18 h-day/6 h-night cycle, a 22°C-day/18°C-night regime and 70% humidity) as previously described (Efrose et al. Citation2008). Prior to germination, seeds were scarified for 5 min with H2SO4, sterilized for 20 min in a solution containing 2% (v/v) NaOCl-0.02% (v/v) Tween 20, and finally rinsed three times in sterile, distilled water. Seeds were pre-germinated at 18°C in the dark for 72 h and the small plants were grown in B&D nutrient solution (Broughton & Dilworth, Citation1971). For the inoculation with rhizobia, 72 h-old seedlings were spot-infected with an A 600 of 0.1 suspension culture of Mesorhizobium loti (strain R7A) and the plants were grown in nitrogen-free B&D nutrient solution.
Arabidopsis thaliana plants were grown in a growth chamber on potting soil under 16 h-day/8 h-night cycle, 22°C regime, 60% relative humidity.
Plasmid vectors and plasmid construction
Plasmid Ljnest8c2r carries the putative entire nucleotide sequence of LjPLT4 cDNA. For the amplification of LjPLT4 coding sequence two sets of primers were used. The one amplified the authentic stop codon containing fragment: sense, 5′-ACGGCTCGAGTCTAGAAAAATGACTGAAGGCAAG-3′ and antisense, 5′-TCGGCTCGAGGTTTAAACTTGGCCTGCTTG-3′. The other amplified the fragment that did not carry the stop codon, using the antisense primer: 5′-TCGTTGCTCGAGGAACTTGGCCTGCTTG-3′. All primers contain an XhoI restriction site. PCR conditions were as follows: 94°C for 3 min, followed by 20 cycles of 94 °C (1 min), 55°C (1 min), 72°C (1 min 50 sec) and a final extension of 72°C (10 min). Each amplified fragment was cloned to the 3′-T overhangs of the pGEM-T Easy plasmid vector (Promega, Madison, WI, USA), under T7 promoter, giving rise to pGEM-LjPLT4(stop) and pGEM-LjPLT4(XhoI), respectively.
Plasmid pAVA554 is a plant vector expressing eYFP under the transcriptional control of the dual 35S CaMV promoter. pAVA554-LjPLT4 expressing LjPLT4-YFP fusion protein was constructed as follows: LjPLT4 nucleotide sequence was amplified by PCR from pGEM-LjPLT4(stop) using the primers: sense, 5′-AAAACCATGGCTACTGAAGGCAAGGTAG-3′ and antisense, 5′-TCTACCATGGCAACTTGGCCTGCTTG-3′, that contain an NcoI restriction site. The PCR conditions were as above. The PCR product was digested with NcoI and cloned into the NcoI restriction site of pAVA554.
Plasmid pDR196 is a yeast expression vector. pDR196-LjPLT4, expressing LjPLT4 transporter, under the transcriptional control of PMA1 promoter, was constructed by cloning the XhoI-XhoI fragment of pGEM-LjPLT4(stop) into the XhoI site of pDR196. pDR196-LjPLT4-GFP was constructed as follows: The XhoI-XhoI fragment of pGEM-LjPLT4(XhoI) was digested and inserted in the SalI site of pOLGFP65C. Then, the XbaI-XbaI fragment, of the constructed plasmid, was inserted into the SpeI site of pDR196. pDR196-GFP was constructed by cloning the GFP nucleotide sequence containing SpeI-XbaI fragment of pOLGFP65C into the SpeI site of pDR196. All plasmid constructs were confirmed by sequencing.
Uptake of radio-labelled substrates
S. cerevisiae RS453 strain (Mat a, ade2-1, trp1-1, can1-100, leu2-3, his3-1, ura3-52) was used for the uptake experiments. This strain is characterized by a rather low background of glucose uptake, inability to grow on synthetic complete (SC) medium with sorbitol as a sole carbon source, and undetectable endogenous sorbitol dehydrogenase activity (Sauer & Stadler Citation1993), and it has been successfully used to express other plant polyol transporters. Transformed cultures were grown in synthetic complete-uracil liquid medium (SC-uracil) supplemented with 2% (w/v) glucose. Prior to the uptake experiments, the yeast cultures were grown in SC-uracil liquid medium supplemented with 3% (v/v) glycerol and 0.05% (w/v) glucose, in order to reduce glucose suppression of the expression of LjPLT4 (Noiraud et al. Citation2001a), until the early logarithmic phase (an A 600 of 0.6), subsequently washed two times with ddH2O and suspended in the appropriate uptake buffer to a final A 600 of 3. As uptake buffer was used either the 25 mM MES of fixed pH or the SC-uracil medium containing 25 mM MES of fixed pH. Aliquots (100 μl) of the suspended cells were mixed with 100 μl of the corresponding, substrate containing, uptake buffer and incubated at 28°C, in a water bath. The concentration of the substrate and the time of incubation were as indicated. The radioactive substrates used were 14C-sucrose, 3H-glucose, 14C-glycerol, 3H-mannitol, 3H-myo-inositol (Perkin Elmer, Waltham MA, USA), 14C-fructose, 14C-xylose (Amersham Vienna, Austria), 14C-sorbitol (Sigma, St Louis MO, USA), 3H-galactitol and 3H-xylitol (ARC), and the radioactivity of each reaction mixture was 0,67 μCi of 14C- or 3H- per ml. The inhibitors carbonyl cyanide m-chlorophenylhydrazone (CCCP) and p-chloro mercury benzene sulfonic acid (PCMBS) were added to the uptake reaction, when necessary, at a concentration of 50 μM and 100 μM, respectively. Uptake procedure was stopped by the addition of 4 ml of ice-cold ddH2O and the uptake mixture was filtered on glass fiber filters, in a vacuum filtration apparatus. After two rapid washes of the cells with 4 ml of ice-cold ddH2O, the yeast carrying filters were transferred to liquid scintillation vials and the accumulated radioactivity was counted. For determination of the uptake rates, the suspended yeast cells were lysed under alkaline-boiling conditions, treated with Triton-X and the concentration of proteins was determined by the Bradford assay (Biorad, Hercules, CA, USA).
Particle bombardment and eYFP/GFP imaging
Microprojectile bombardment of A. thaliana Columbia young rosette leaves with the pAVA554-LjPLT4 or pAVA554 plasmids was performed using a Bio-Rad PDS-1000 biollistic system according to the manufacturer's standard protocols. Imaging of eYFP and chlorophyll autofluorescence was performed by confocal microscopy using a Leica TCS-SP microscope 6 to 24 h after bombardment. The argon lazer line (488 nm) was used for excitation of eYFP and GFP while the photo multiplier tube settings were adjusted to eliminate the cholrophyl autofluorescence when imaging eYFP.
RNA extraction and Real-Time RT-PCR
Organs of L. japonicus plants were harvested and ground in liquid nitrogen. Total RNA was isolated according to Brusslan and Tobin (Citation1992) and quantified by spectrophotometry and agarose gel electrophoresis. Prior to RT-PCR, the total RNA samples were treated with DNase I (Promega, Madison, WI, USA) at 37°C for 45 min, in order to eliminate any traces of contaminating genomic DNA. First-strand cDNA was reverse transcribed from 2 μg of DNase-treated total RNA using SuperScript II reverse transcriptase (Invitrogen, Paisley, UK). The reaction mixture was incubated at 42°C for 50 min, followed by heat inactivation at 70°C for 15 min. The resulting first-strand cDNA was diluted to a final volume of 200 μl, and target cDNAs were amplified using gene specific primers LjPLT4-F (5′-ACTTCTTCCAACAAGCCTCCG-3′) and LjPLT4-R (5′-CGGCTTTTTCGAAGATGGTG-3′), designed from the transcribed region of LjPLT4 gene using Primer Express 1.5 software (Applied Biosystems, Darmstadt, Germany). Quantitative RT-PCR reactions were performed on the ABI PRISM 7900HT Sequence Detection System using Power SYBR Green master mix (Applied Biosystems), gene-specific primers at a final concentration of 0.2 μM each and 1 μl of the cDNA as template. PCR cycling started with the initial polymerase activation at 95°C for 10 min, followed by 40 cycles of 95°C for 15 sec and 60°C for 1 min. The primer specificity and the formation of primer-dimers were monitored by dissociation curve analysis and agarose gel electrophoresis on a 4% (w/v) gel. The expression levels of a L. japonicus ubiquitin gene were used as internal standards to normalize small differences in cDNA template amounts using LjUBQF (5′-TTCACCTTGTGCTCCGTCTTC-3′) and LjUBQR (5′-ACCACCAGCACACACACAGACAATCC-3′) primers. For the relative quantification of gene expression, a modification of the comparative threshold cycle method was used. In the case of symbiotic and non-symbiotic organs, relative transcript levels of the gene of interest (X) were calculated as a ratio to the ubiquitin gene transcripts (U), as (1+E)-ΔCt, where ΔCt was calculated as (Ct X-Ct U). PCR efficiency (E) for each amplicon was calculated employing the linear regression method on the Log(Fluorescence) per cycle number data, using the LinRegPCR software (Ramakers et al. Citation2003). All real-time qPCR reactions were performed as triplicates on independent biological repeats.
Metabolite extraction, derivatization and GC-MS analysis
Mature leaves, stems, primary and lateral roots without nodules, and mature pink nodules were harvested from 5-week-old L. japonicus plants, immediately frozen in liquid nitrogen and stored at –70°C. Approximately, 60 mg fresh weight of tissue was ground in liquid nitrogen. Ground samples were extracted with 360 μl methanol and 20 μl ribitol in methanol (0.2 mg ml-1). Samples were incubated at 70°C for 15 min with continuous shaking. After the addition of 200 μl chloroform, the samples were further incubated at 37°C for 5 min under continuous shaking. After addition of 400 μl ddH2O, samples were vortexed and then centrifuged at 18,000 g for 5 min at room temperature. The aqueous phase containing the polar metabolite fraction was transferred into new eppendorf tubes and dried by vacuum centrifugation. For derivatization, dried samples were re-suspended in 40 μl methoxyamine-HCl (MOX) (20 mg ml-1 in pyridine), and incubated at 30°C for 90 min with continuous gentle agitation. This was followed by addition of 70 μl of N-methyl-N-(trimethylsilyl)-trifluoroacetamide (MSTFA) together with 10 μl of n-alkane mix for determination of retention indexes (RIs). Gas-chromatography coupled to Mass-spectrometry (GC-MS) measurement were performed in a HP6890 GC coupled to a HP 5973 MS. For all organs tested, five biological replications were conducted. The chromatograms were evaluated automatically using the AMDIS software and metabolites were identified using the Golm metabolome database (Kopka et al. Citation2005, Schauer et al. Citation2005). Xylitol content was confirmed manually. Results were expressed as a response that corresponds to the ratio between the areas of the target metabolite divided by the area of the reference metabolite (ribitol, m/z 319) and reported relative to the fresh weight.
Results and discussion
L. japonicus cDNA clones coding for putative polyol/H+ co-transporters
BLAST searches of EST databases derived from L. japonicus cDNA libraries revealed the presence of four tentative consensus (TC) sequences with high similarity to PLT monosaccharide transporter genes. The largest cDNA clone corresponding to each TC was obtained, their complete nucleotide sequences determined, and named LjPLT1 (AM084328), LjPLT2 (AM084329), LjPLT3 (AM084330) and LjPLT4 (AM084331). The nucleotide sequences matched the corresponding TC contig sequences. LjPLT1 encodes a polypeptide of 490 amino acids (aa), LjPLT2 495 aa, LjPLT3 501 aa, and LjPLT4 519 aa, with the N- and C-termini harbouring the greatest differences in length and aa sequence. LjPLT1, LjPLT3 and LjPLT4 clones correspond to full-length cDNAs, since a stop codon is present upstream of the putative methionine start codon in each case, LjPLT2 cDNA may lack the true start codon, although it encodes a protein of about the same length as the other three transporters. Sequences up-stream (5') of the putative initiation codons of LjPLT1, LjPLT3 and LjPLT4, are rich in A/C nucleotides and, therefore, provide a good Kozak frame for the translation machinery. Hydropathy analysis of the deduced aa sequences revealed a common structure for all L. japonicus PLTs, namely 12 trans-membrane helices with a cytoplasm-exposed loop of approximately 70 aa between helices 6 and 7. These features are typical of MFS transporters. The deduced aa sequences of all four LjPLTs contain most of the consensus sequences and charges conserved between members of the MFS and the aa signatures of sugar transporters () (Griffith et al. Citation1992). Single aa substitutions in these motifs include the first Pro of the PESPRXL motif, present at the 6th trans-membrane helix, which is substituted by Gly and Val in the aa sequences of LjPLT2 and LjPLT3, respectively. Also, Lys in the LPETKGXXXE motif, present at the cytoplasmic C-terminus, is substituted by Arg in the aa sequence of LjPLT4. Finally, a GRR motif is present between the 2nd and 3rd and between the 8th and 9th trans-membrane helixes in all four LjPLTs.
Figure 1. Comparison of the deduced aa sequences of L. japonicus LjPLT1, LjPLT2, LjPLT3 and LjPLT4 with previously characterized monosaccharide transporters. Sequences shown include: AtPLT5, Arabidopsis thaliana polyol transporter (NP_188513); AgMAT1, Apium graveolens mannitol transporter (AAG43998); PmPLT1, Plantago major polyol transporter 1 (CAD58709); PcSOT1, Prunus cerasus sorbitol transporter 1 (AAO39267). Identical residues are boxed in black, whereas conservative changes are shown in grey. Dashes represent gaps inserted to optimize the alignment. Sequences were aligned using ClustalW with PAM250 residue weight table. Transmembrane helices of LjPLT4 as predicted by HMMTOP software are indicated by the black line. The dashed lines indicate PESPRXL, LPETKGXXXE and two GRR conserved motifs.
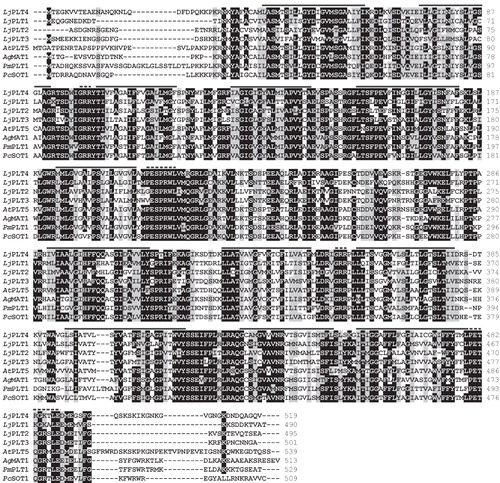
Phylogenetic analysis using monosaccharide, polyol, and sucrose transporters, places LjPLT1, LjPLT2, LjPLT3 and LjPLT4 in two different branches of the clade formed by polyol transporter (). LjPLT1, LjPLT2, and LjPLT3 group together and exhibit between 51–65% identity. LjPLT4 is in a separate branch together with AtPLT5, AgMAT1 and other previously characterized PLT family members. Interestingly, LjPLT4 is more similar to the well characterized AtPLT5 (70.5% identity) (Klepek et al. Citation2005, Reinders et al. Citation2005), than to the other three L. japonicus PLTs.
Figure 2. Phylogenetic relationships of L. japonicus LjPLT1, LjPLT2, LjPLT3 and LjPLT4 amino acid sequences to other sucrose, monosaccharide and polyol transporters. Full length amino acid sequences were aligned with the ClustalW program. Evolutionary history was inferred using the Neighbor-Joining method and evolutionary distances were computed using the Poisson correction method. The tree is drawn to scale, with branch lengths in the same units as those of the evolutionary distances used to infer the phylogenetic tree. Bootstrap values from 1,000 replicates are given at each node. Phylogenetic analyses were conducted using MEGA4 (Tamura et al. Citation2007). Sequences included as follows: AtPLT5, Arabidopsis thaliana PLT5 (NP_188513); AtPLT1, A. thaliana PLT1 (NP_179209); AgMAT1, Apium graveolens mannitol transporter (AAG43998); PmPLT1, Plantago major polyol transporter 1 (CAD58709); MdSOT1, Malus domestica sorbitol transporter 1 (AAO88964); PcSOT2, Prunus cerasus putative sorbitol transporter (AAM44082); OsPST2, Oryza sativa putative sugar transporter (AAL14615); AtPLT6, A. thaliana putative mannitol transporter (NP_195385.1); LbXYLT, Lactobacillus brevis D-xylose proton-symporter (AAC95127.1); AtMSS1, A. thaliana carbohydrate transmembrane transporter (NP_198006); VvMST, Vitis vinifera putative hexose transporter (XP_002281683); AtSTP3, A. thaliana monosaccharide transporter (CAA05384); AtSTP4, A. thaliana sugar transporter 4 (NP_188627); OsMST3, O. sativa monosaccharide transporter 3 (BAB19864); NtMSTP1, Nicotiana tabacum monosaccharide transporter (CAA47324.1); MtMST1, Medicago truncatula sugar transporter (AAB06594); AtSTP1, A. thaliana sugar transporter 1 (NP_172592); DgMST, Datisca glomerata monosaccharide-H+ symporter (CAD30830.1); OsSUC, O. sativa putative sucrose transporter (ABF94212.1); AtSUT2, A. thaliana sucrose transporter 2 (NP_973404); LjSUT4, Lotus japonicus sucrose transporter 4 (CAD61275); VvSUC, V. vinifera sucrose transporter (AAD55269); AtSUT4, A. thaliana sucrose transporter (AAG09191); PmSUC1, P. major sucrose transporter (CAI59556); NtSUT1a, N. tabacum sucrose transporter (CAA57727); AtSUC2, A. thaliana sucrose-proton symporter 2 (NP_173685.1); AtSUC9, A. thaliana sucrose-proton symporter 9 (NP_196235.1).
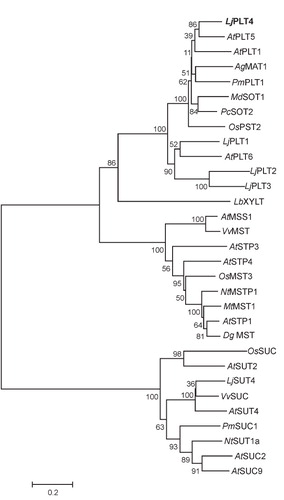
Functional characterization of LjPLT4 in yeast
The repression of polyol uptake and metabolism by Saccharomyces cerevisiae grown on medium containing glucose as the carbon source has made this system suitable for biochemical studies of plant polyol transporters. However, prior to functional characterization of LjPLT4 transporter, its subcellular localization in the yeast cells was determined. To this end, LjPLT4 was expressed in yeast as an N-terminal fusion to green fluorescence protein (GFP). S. cerevisiae (strain RS453) cells were transformed either with pDR196-LjPLT4-GFP or pDR196-GFP plasmids, driving the expression of LjPLT4-GFP fusion or GFP polypeptides, respectively. Confocal microscopy of serial optical sections through a number of GFP-expressing cells showed GFP fluorescence in both the cytoplasm and nucleus of the expressing cells (). In contrast, in LjPLT4-GFP expressing cells fluorescence was associated with the plasma membrane (), suggesting that LjPLT4 is targeted to the plasma membrane of transformed yeast cells. Thus, S. cerevisiae was considered an appropriate expression system for functional characterization of LjPLT4.
Figure 3. Subcellular localization of LjPLT4-GFP fusion protein in Saccharomyces cerevisiae and of LjPLT4-eYFP transiently expressed in Arabidopsis thaliana leaf epidermal cells. GFP and eYFP fluorescence was detected by confocal microscopy. Localization of GFP (A) and LjPLT4-GFP (B) in yeast cells and localization of eYFP (C) and LjPLT4-eYFP (D) in Arabidopsis epidermal cells are presented in colour in the online version. n: nucleus; p: plasma membrane.
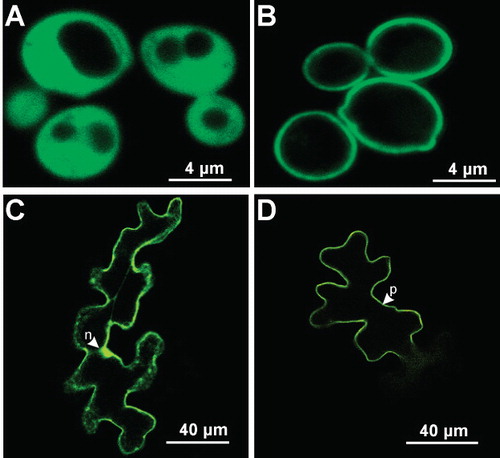
To determine the functional properties of LjPLT4, its coding region was cloned into plasmid pDR196, and yeast cells were transformed with the pDR196-LjPLT4 construct. Yeast cells transformed with the empty pDR196 vector were used as a negative control. LjPLT4 transport activity was initially examined at pH 4.5, a value commonly used for the characterization of H+-symporters, using a number of different polyols and sugars as potential substrates. summarizes the polyols/sugars tested, their concentration in the uptake reaction, and their relative rate of uptake by LjPLT4-expressing cells compared to pDR196-transformed control cells. Yeast cells expressing LjPLT4 exhibited rates of 3H-xylitol uptake that were 5- to 6-fold higher than yeast cells transformed with pDR196 alone (). In contrast, uptake rates for all other radiolabelled substrates tested were similar in LjPLT4-transformed and control cells. 3H-xylitol uptake via LjPLT4 was apparent even when a 10-fold lower concentration of xylitol was used, indicating that the transporter has a relatively high affinity for this substrate. Increasing pH from 4.5 to 6.0 resulted in a decrease in the rate of xylitol uptake, as expected from a H+-symporter. To our knowledge, this is the first report of a xylitol-specific polyol transporter. Moreover, with the exception of the sorbitol-specific transporters, PcSOT1 and PcSOT2 (Gao et al. Citation2003), which have been implicated in fruit maturation, no other PLTs exhibiting high substrate specificity are known. Although our phylogenetic analysis indicated that LjPLT4 is a close homologue of AtPLT5, with 70.5% identity at the aa level, they have markedly different transport activities, as AtPLT5 is a non-specific monosaccharide transporter able to transport both linear and cyclic polyols as well as different hexoses and pentoses (Klepek et al. Citation2005; Reinders et al. Citation2005). Similarly, AtPMT1 and AtPMT2 are promiscuous monosaccharide transporters, although xylitol and fructose were found to be transported at higher rates than other substrates (Klepek et al. Citation2010). Therefore, it appears likely that substrate specificity of the PLT family members may be determined by a small number of aa substitutions, probably located in the transmembrane domains and/or extra-cellular loops. Clearly, further work, such as site-directed mutagenesis coupled to transport assays, is required to understand better the relationship between structure and function of PLT proteins.
Figure 4. Relative uptake levels of various radiolabelled substrates in LjPLT4 yeast expressing cells (pDR196+LjPLT4 transformed). Relative uptake levels are expressed as percentage of the uptake of the tested substrate by LjPLT4-expressing yeast cells, in relation to the uptake of the respective substrate by yeast cells transformed with the empty pDR196 vector (arbitrary set as 100%). The pH conditions, the different polyols/sugars tested and their concentration in the uptake reaction are shown below the bar graph. The incubation time of the uptake reactions was 3 min. The results are means ± SE of four replicates (n = 4).
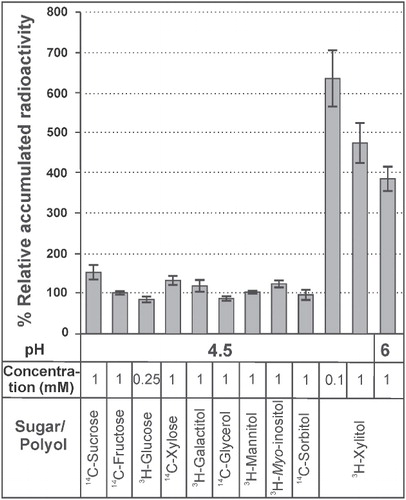
The pH-dependency of the xylitol transport by LjPLT4 was tested further, using a pH range from 4 to 8 (). Control cells exhibited negligible transport rates of 3H-xylitol at all pH values. The pH of the medium remained unchanged, both in cell suspensions prior to use and during the uptake reactions, as monitored in a parallel experiment with non-radioactive xylitol. LjPLT4 exhibited maximal xylitol uptake activity at pH 4.0 with a 75% decline of activity at pH 5.0 and lowest activity at pH 6.0. However, transport remained active over the entire pH range tested and actually increased slightly at pH 8.0 compared to pH 6.0 and 7.0. A similar slight increase of activity at elevated pH was also found for AgMAT1 (Noiraud et al. Citation2001a). However, the increase of activity of these transporters under alkaline pH values in planta remains an open question. The protonophore CCCP had a strong inhibitory effect on xylitol uptake by LjPLT4 (). This finding coupled with the observed pH dependence of xylitol transport activity are consistent with a xylitol/H+ co-transport mechanism, and indicate that LjPLT4 mediated energy-dependent transport of xylitol across the yeast plasma membrane. In contrast, the -SH group inhibitor PCMBS had only a slight inhibitory effect on the LjPLT4-mediated xylitol uptake (). Similar results with PCMBS have been obtained with polyol transporters of other plant species (Noiraud et al. Citation2001a, Gao et al. Citation2003, Klepek et al. Citation2005, Juchaux-Cachau et al. Citation2007). The only PCMBS-sensitive polyol transporter characterized so far is PmPLT1 (Ramsperger-Gleixner et al. Citation2004). The inhibitory effect of PCMBS on PmPLT1 transport activity was attributed to Cys61, which is exposed to the predicted extracellular side of the protein. As shown in , this Cys residue has been substituted by Ile51 in LjPLT4, a substitution which could explain the insensitivity of LjPLT4 xylitol transport activity to PCMBS.
Table I. Inhibition of 3H-xylitol transport by various potential competitors and inhibitors. Uptake of 3H-xylitol was determined in the presence of other substrates. External xylitol concentration was 0.7 mM at pH 4 and 3 mM at pH 8, and a 10-fold excess of the other compounds was used. The incubation time of each uptake reaction was 3 min. Results are means ±SE of four replicates. n.d.: not determined.
Figure 5. pH-dependence of xylitol uptake by transformed yeast cells expressing LjPLT4. Reactions were performed at the pH values indicated using 1 mM xylitol. Incubation time was 3 min. Results represent the net uptake of xylitol by the LjPLT4 expressing cells. The results are the means ± SE of four replicates (n = 4).
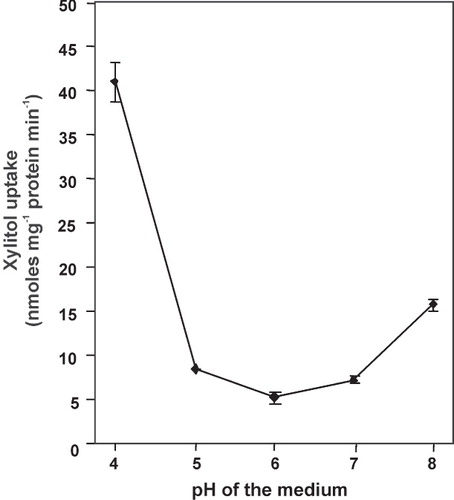
As LjPLT4 exhibited maximal activity at pH 4 but transported xylitol even at pH 8 (), the properties of the transporter were analysed at both pH values. 3H-xylitol uptake rates were linear for at least 5 min at both pH values in the reaction medium (data not shown). The concentration-dependence of xylitol uptake was determined within the linear phase of uptake, by terminating reactions after 3 min, using xylitol concentrations ranging from 0.1–15 mM. The concentration-dependence of xylitol uptake rate was well described by the Michaelis-Menten equation at both pH 4 and 8 ( and ). Lineweaver/Burk plots yielded a K m of 0.34 ± 0.02 mM and a V max of 52.86 ± 0.63 nmoles mg protein-1 min-1 at pH 4, and of a K m of 1.49 ± 0.12 mM and a V max of 40.11 ± 1.03 nmoles mg protein-1 min-1 at pH 8. The decrease in V max at pH 8 may be attributed to the lower concentration of H+ ions at this pH compared to pH 4. Interestingly, the K m values of LjPLT4 expressed in yeast are similar to K m values reported for other polyol transporters including AtPLT5 (0.5 ± 0.1 mM for sorbitol) (Klepek et al. Citation2005), AgMAT1 (0.34 mM for mannitol) (Noiraud et al. Citation2001a), and PcSOT1 and PcSOT2 (0.8 and 0.6 mM for sorbitol, respectively) (Gao et al. Citation2003). Thus, it is not unlikely that xylitol is an in vivo substrate of the LjPLT4 transporter. In fact, given the apparent high-specificity of the transporter (), xylitol may be the primary substrate of LjPLT4.
Figure 6. Concentration dependence of xylitol uptake in LjPLT4-expressing yeast cells at pH 4 (A) and at pH 8 (B). The incubation time of the uptake reaction was 3 min. Results are means ± SE of four replicates (n = 4). Lineweaver-Burk plots of uptake rates are shown in the insets.
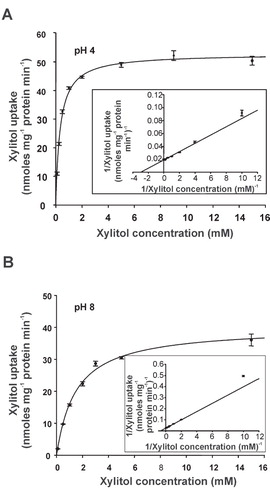
Finally, uptake of 3H-xylitol was determined in the presence of other polyols and sugars at pH 4 and pH 8, using near-saturating concentrations of xylitol (0.7 mM at pH 4 and 3 mM at pH 8) and a 10-fold excess of the tested compounds. Oligosaccharides like raffinose and sucrose had no influence on the xylitol transport rate at pH 4 (). In contrast to all the other plant PLTs tested in yeast so far, mannitol had little effect on xylitol transport by LjPLT4. On the other hand, xylose and fructose inhibited xylitol transport substantially. Other polyols including sorbitol, myo-inositol, galactitol and ribitol inhibited xylitol transport, although to a lesser extent than the sugars fructose and xylose. Since the above inhibitory compounds were not transported by LjPLT4 in yeast, we hypothesize that they compete with xylitol for binding to the active site of LjPLT4. The strong inhibition of LjPLT4 by non-transported monosaccharides is similar to that observed for celery AgMAT1 and AgMAT2 (Noiraud et al. Citation2001a, Juchaux-Cachau et al. Citation2007) and sour cherry PcSOT1 and PcSOT2 (Gao et al. Citation2003). Concerning AgMAT1, although glucose is not transported, it strongly inhibits mannitol transport in the competition studies (Juchaux-Cachau et al. Citation2007). Furthermore, the expression of PcSOT1 and PcSOT2 in yeast does not result in an elevated transport activity of glucose, however a strong inhibition of sorbitol transport was observed in the presence of glucose (Gao et al. Citation2003). An alternative explanation that the authors proposed was that the above inhibitory effect could be an artifact of the yeast expression system, as transporter expression may be repressed by the addition of these sugars (Horak & Wolf Citation1997). However, the existence and significance of this inhibition in planta remains to be elucidated. As expected, 3H-xylitol uptake was most strongly inhibited by a 10-fold excess of unlabelled xylitol at both pH values tested. Interestingly the inhibitory effects of all compounds tested, apart from xylitol, were greater at pH 4 than at pH 8.
Subcellular localization of LjPLT4-eYFP fusion protein in planta
To identify the subcellular location of LjPLT4 in planta, the protein was expressed as an N-terminal fusion to eYFP, using the pAVA554 plasmid vector. eYFP was used as negative control. Plasmid constructs were bombarded into the lower epidermis of young rosette leaves of A. thaliana plants, as transformation of L. japonicus leaf cells was hampered by the thick cell wall, and the localization of proteins was determined by analyses of serial optical sections, using confocal microscopy. Cells expressing eYFP exhibited a fluorescent nucleus, cytoplasm and cytoplasmic bridges (). In contrast, LjPLT4-eYFP fluorescence associated exclusively with the cell periphery and was never observed inside or around the nucleus or in any other cell organelle (). Additionally, no fluorescent cytoplasmic bridges were observed in these cells. These data, together with the localization of LjPLT4-GFP in the yeast plasma membrane () and the observed transport activity in yeast plasma membrane, clearly support a plasma membrane localization for the LjPLT4 polypeptide. Plasma membrane localization has also been reported for AtPLT5-GFP in Arabidopsis and onion epidermis cells (Klepek et al. Citation2005), for AtPMT1- and AtPMT2-GFP fusions in Arabidopsis cells (Klepek et al. Citation2010), and for the AgMAT2 mannitol transporter in celery, using specific antibodies (Juchaux-Cachau et al. Citation2007). Although polyols are known to accumulate in vacuoles, either by active transport (Yamaki Citation1987) or by a facilitated diffusion mechanism (Greutert et al. Citation1998), only the myo-inositol transporters Mitr1 and Mitr2 from Mesembryanthemum crystalinum (Chauhan et al. Citation2000) and AtINT1 from A. thaliana (Schneider et al. Citation2008) have been shown to be targeted to the tonoplast membrane.
Accumulation of LjPLT4 transcripts and xylitol in various L. japonicus organs
The accumulation of LjPLT4 transcripts in different L. japonicus organs of 5-week-old plants was measured by quantitative RT-PCR. Total RNA was isolated from different L. japonicus organs (mature nodules at 28 days-post-inoculation, roots, stems, and leaves) and subjected to reverse-transcription and real-time quantitative polymerase chain reaction (RT-qPCR) analysis. The highest levels of LjPLT4 transcripts were detected in leaves, while almost 2-fold lower transcript levels were detected in nodules, roots and stems (). LjPLT4 was expressed at relatively low levels compared to the reference gene ubiquitin, in all organs tested. Similar results were obtained for the close homologue AtPLT5, which was found to accumulate at low levels in most organs and tissues, using a different range of techniques including GUS, GFP and microarray analysis (Klepek et al. Citation2005, Reinders et al. Citation2005). AtPLT5 has been proposed to participate in the re-uptake of multiple substrates from the apoplast in many tissues. LjPLT4 may play a similar role, albeit with a much narrower substrate range. Interestingly, recent transcriptomic studies concerning the effect of salinity stress in L. japonicus plants revealed that LjPLT4 transcript accumulation increased up to 3-fold in the stressed plants. Furthermore, in both experimental approaches applied (initial and gradual acclimatization of plants) LjPLT4 induction followed the stress dose. The reprogramming of LjPLT4 gene expression under salinity conditions may imply a role of this transporter in maintaining the ionic and osmotic homeostasis of the plant cell (Sanchez et al. Citation2008).
Figure 7. Quantitative RT-PCR analysis of LjPLT4 mRNA expression and GC-MS analysis of xylitol in L. japonicus organs. (A) Accumulation of LjPLT4 gene transcripts in different plant organs. Total RNA was isolated and poly(A)+-RNA was reverse transcribed to cDNA, and subjected to real-time quantitative PCR using gene specific primers. Relative mRNA level was calculated relative to the level of the ubiquitin transcripts. Bars show means +SD (n = 4). (B) Xylitol accumulation in different plant organs. Metabolites were extracted, derivatized, and analyzed by gas chromatography coupled to mass spectrometry (GC-MS), as described in Material and methods. Xylitol accumulation is expressed as a relative response ratio (Desbrosses et al. Citation2005). Bars represent the average response of five independent biological repeats +SD (n = 5).
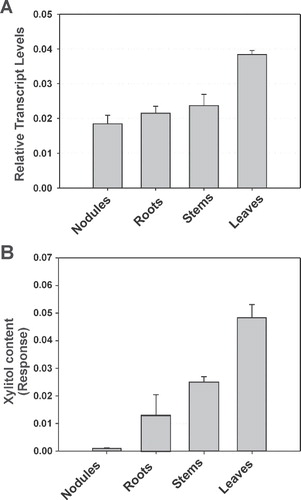
To test the hypothesis that xylitol is the natural substrate of LjPLT4 in planta, GC-MS was used to profile metabolites in nodules, roots, stems and leaves of L. japonicus plants. Evaluation of chromatographs obtained from these organs revealed that xylitol accumulated to different levels in all organs tested (). Interestingly, although xylitol levels were lower than those of other polyols, including mannitol, myo-inositol and pinitol (data not shown), the pattern of xylitol accumulation resembled that of LjPLT4 gene expression. In particular, the highest xylitol levels were observed in leaves, while 2-fold lower levels were measured in stems. Very low levels of xylitol were detected in mature nitrogen fixing nodules. In all cases, the relative amounts of xylitol was found to be up to 6-fold lower than mannitol, and comparable to other polyols including sorbitol, galactitol and arabitol (data not shown). The exact physiological role of xylitol in plants remains obscure. However Arabidopsis plants grown under drought or heat and drought conditions, were found to accumulate xylitol, among other metabolites, indicating a possible protective role of xylitol against these abiotic stresses (Rizhsky et al. Citation2004).
Conclusions
We identified four members of the polyol transporter family in the model legume L. japonicus and determined that one of them, namely LjPLT4, is a plasma membrane xylitol-specific H+-symporter, ubiquitously expressed in all plant organs. When expressed in yeast, LjPLT4 mediated uptake of xylitol alone, with an affinity similar to that described for other polyol transporters. Although no other compounds where found to be transported by LjPLT4, some were found to inhibit xylitol uptake in yeast, possibly by competing with xylitol for binding to the active site. The narrow substrate range of LjPLT4 makes it an interesting model for future studies on the relationship between structure and substrate specificity of the members of the polyol transporter family. Further work is required to determine the exact physiological role(s) of LjPLT4 in planta, and whether xylitol transport and accumulation are related to stress responses in L. japonicus. We plan to use reverse genetics, including TILLING and RNA interference, to clarify the role of LjPLT4 in L. japonicus. Advances in all relevant knowledge could eventually be exploited in crop breeding for resistance against various stresses.
Acknowledgements
This work was co-funded by the European Social fund and Greek national resources – project PYTHAGORAS II (funding of research groups in Agricultural University of Athens) (EF), and by the bi-lateral French-Greek program, Platon (Molecular and biochemical characterization of monosaccharide transporters in root nodules) (RL and EF). The work was also supported, in part, by the Max Planck Society (MKU).
Declaration of interest: The authors report no conflicts of interest. The authors alone are responsible for the content and writing of the paper.
References
- Broughton WJ, Dilworth MJ. 1971. Control of leghaemoglobin synthesis in snake beans. Biochem J 125:1075–1080.
- Brown PH, Bellaloui N, Hu H, Dandekar A. 1999. Transgenically enhanced sorbitol synthesis facilitates phloem boron transport and increases tolerance of tobacco to boron deficiency. Plant Physiol 119:17–20.
- Brusslan JA, Tobin EM. 1992. Light-independent developmental regulation of cab gene expression in Arabidopsis thaliana seedlings. Proc Natl Acad Sci USA 89:7791–7795.
- Chauhan S, Forsthoefel N, Ran Y, Quigley F, Nelson DE, Bohnert HJ. 2000. Na+/myo-inositol symporters and Na+/H+-antiport in Mesembryanthemum crystallinum. Plant J 24:511–522.
- Conde C, Silva P, Agasse A, Lemoine R, Delrot S, Tavares R, Geros H. 2007. Utilization and transport of mannitol in Olea europaea and implications for salt stress tolerance. Plant Cell Physiol 48:42–53.
- Desbrosses GG, Kopka J, Udvardi MK. 2005. Lotus japonicus metabolic profiling. Development of gas chromatography-mass spectrometry resources for the study of plant-microbe interactions. Plant Physiol 137:1302–1318.
- Efrose RC, Flemetakis E, Sfichi L, Stedel C, Kouri ED, Udvardi MK, Kotzabasis K, Katinakis P. 2008. Characterization of spermidine and spermine synthases in Lotus japonicus: induction and spatial organization of polyamine biosynthesis in nitrogen fixing nodules. Planta 228:37–49.
- Everard JD, Gucci R, Kann SC, Flore JA, Loescher WH. 1994. Gas exchange and carbon partitioning in the leaves of celery (Apium graveolens L.) at various levels of root zone salinity. Plant Physiol 106:281–292.
- Gao Z, Maurousset L, Lemoine R, Yoo SD, van Nocker S, Loescher W. 2003. Cloning, expression, and characterization of sorbitol transporters from developing sour cherry fruit and leaf sink tissues. Plant Physiol 131:1566–1575.
- Greutert H, Martinola E, Keller F. 1998. Mannitol transport by vacuoles of storage parenchyma of celery petioles operates by facilitated diffusion. J Plant Physiol 153:91–96.
- Griffith JK, Baker ME, Rouch DA, Page MG, Skurray RA, Paulsen IT, Chater KF, Baldwin SA, Henderson PJ. 1992. Membrane transport proteins: implications of sequence comparisons. Curr Opin Cell Biol 4:684–695.
- Guo CX, Oosterhuis DM. 1995. Pinitol occurrence in soybean plants as affected by temperature and plant-growth regulators. J Exp Bot 46:249–253.
- Horak J, Wolf DH. 1997. Catabolite inactivation of the galactose transporter in the yeast Saccharomyces cerevisiae: ubiquitination, endocytosis, and degradation in the vacuole. J Bacteriol 179:1541–1549.
- Hu HN, Penn SG, Lebrilla CB, Brown PH. 1997. Isolation and characterization of soluble boron complexes in higher plants – The mechanism of phloem mobility of boron. Plant Physiol 113:649–655.
- Juchaux-Cachau M, Landouar-Arsivaud L, Pichaut JP, Campion C, Porcheron B, Jeauffre J, Noiraud-Romy N, Simoneau P, Maurousset L, Lemoine R. 2007. Characterization of AgMaT2, a plasma membrane mannitol transporter from celery, expressed in phloem cells, including phloem parenchyma cells. Plant Physiol 145:62–74.
- Klepek YS, Geiger D, Stadler R, Klebl F, Landouar-Arsivaud L, Lemoine R, Hedrich R, Sauer N. 2005. Arabidopsis Polyol Transporter5, a new member of the monosaccharide transporter-like superfamily, mediates H+-Symport of numerous substrates, including myo-inositol, glycerol, and ribose. Plant Cell 17:204–218.
- Klepek Y-S, Volke M, Konrad KR, Wippel K, Hoth S, Hedrich R, Sauer N. 2010. Arabidopsis thaliana Polyol/Monosaccharide Transporters 1 and 2: fructose and xylitol/H+ symporters in pollen and young xylem cells. J Exp Bot 61:537–550.
- Kopka J, Schauer N, Krueger S, Birkemeyer C, Usadel B, Bergmuller E, Dormann P, Weckwerth W, Gibon Y, Stitt M, Willmitzer L, Fernie AR, Steinhauser D. 2005. [email protected]: the Golm Metabolome Database. Bioinformatics 21:1635–1638.
- Marger MD, Saier MH, Jr. 1993. A major superfamily of transmembrane facilitators that catalyse uniport, symport and antiport. Trends Biochem Sci 18:13–20.
- Noiraud N, Maurousset L, Lemoine R. 2001a. Identification of a mannitol transporter, AgMaT1, in celery phloem. Plant Cell 13:695–705.
- Noiraud N, Maurousset L, Lemoine R. 2001b. Transport of polyols in higher plants. Plant Physiol Biochem 39:717–728.
- Patonnier MP, Peltier JP, Marigo G. 1999. Drought-induced increase in xylem malate and mannitol concentrations and closure of Fraxinus excelsior L-stomata. J Exp Bot 50:1223–1229.
- Pommerrenig B, Papini-Terzi FS, Sauer N. 2007. Differential regulation of sorbitol and sucrose loading into the phloem of plantago major in response to salt stress. Plant Physiol 144:1029–1038.
- Ramakers C, Ruijter JM, Deprez RH, Moorman AF. 2003. Assumption-free analysis of quantitative real-time polymerase chain reaction (PCR) data. Neurosci Lett 339:62–66.
- Ramsperger-Gleixner M, Geiger D, Hedrich R, Sauer N. 2004. Differential expression of sucrose transporter and polyol transporter genes during maturation of common plantain companion cells. Plant Physiol 134:147–160.
- Reinders A, Panshyshyn JA, Ward JM. 2005. Analysis of transport activity of Arabidopsis sugar alcohol permease homolog AtPLT5. J Biol Chem 280:1594–1602.
- Rizhsky L, Liang HJ, Shuman J, Shulaev V, Davletova S, Mittler R. 2004. When defense pathways collide. The response of Arabidopsis to a combination of drought and heat stress. Plant Physiol 134:1683–1696.
- Rumpho ME, Edwards GE, Loescher WH. 1983. A pathway for photosynthetic carbon flow to mannitol in celery leaves – Activity and localization of key enzymes. Plant Physiol 73:869–873.
- Sanchez DH, Lippold F, Redestig H, Hannah MA, Erban A, Krämer U, Kopka J, Udvardi MK. 2008. Integrative functional genomics of salt acclimatization in the model legume Lotus japonicus. Plant J 53:973–987.
- Sauer N, Stadler R. 1993. A sink-specific H+/monosaccharide co-transporter from Nicotiana tabacum: cloning and heterologous expression in baker's yeast. Plant J 4:601–610.
- Schauer N, Steinhauser D, Strelkov S, Schomburg D, Allison G, Moritz T, Lundgren K, Roessner-Tunali U, Forbes MG, Willmitzer L, Fernie AR, Kopka J. 2005. GC-MS libraries for the rapid identification of metabolites in complex biological samples. FEBS Lett 579:1332–1337.
- Schneider S, Beyhl D, Hedrich R, Sauer N. 2008. Functional and physiological characterization of Arabidopsis Inositol Transporter1, a novel tonoplast-localized transporter for myo-inositol. Plant Cell 20:1073–1087.
- Shen B, Jensen RG, Bohnert HJ. 1997. Mannitol protects against oxidation by hydroxyl radicals. Plant Physiol 115:527–532.
- Stoop JMH, Williamson JD, Pharr DM. 1996. Mannitol metabolism in plants: A method for coping with stress. Trends Plant Sci 1:139–144.
- Tamura K, Dudley J, Nei M, Kumar S. 2007. MEGA4: Molecular Evolutionary Genetics Analysis (MEGA) software version 4.0. Mol Biol Evol 24:1596–1599.
- Watari J, Kobae Y, Yamaki S, Yamada K, Toyofuku K, Tabuchi T, Shiratake K. 2004. Identification of sorbitol transporters expressed in the phloem of apple source leaves. Plant Cell Physiol, 45:1032–1041.
- Yamaki S. 1987. ATP-promoted sorbitol transport into vacuoles isolated from apple fruit. Plant Cell Physiol 28:557–564.