Abstract
Free fatty acids released during intralumenal digestion of dietary fat must pass through the enterocyte brush border membrane before triacylglycerol reassembly and subsequent chylomicron delivery to the lymph system. In the present work fluorescent BODIPY fatty acid analogs were used to study this membrane passage in organ cultured intestinal mucosal explants. We found that in addition to a rapid uptake into the cytoplasm, a fraction of the fatty acid analogs were inserted directly into the brush border membrane. Furthermore, a brief exposure of microvillar membrane vesicles to a fat mixture mimicking a physiological solution of dietary mixed micelles, rearranged the lipid raft microdomain organization of the membranes. Thus, the fat mixture generated a low-density subpopulation of microvillar detergent resistant membranes (DRMs) highly enriched in alkaline phosphatase (AP). Since this GPI-linked enzyme is the membrane protein in the brush border with the highest affinity for lipid rafts, this implies that free fatty acids selectively insert stably into these membrane microdomains. We have previously shown that absorption of dietary lipids transiently induce a selective endocytosis of AP from the brush border, and from work by others it is known that fat absorption is accompanied by a rise in serum AP and secretion of surfactant-like particles from enterocytes. We propose that these physiological processes may be triggered by the sequestering of dietary free fatty acids in lipid raft microdomains of the brush border.
Introduction
Most of the nutrients of the diet are absorbed in the small intestine by passage through the enterocyte brush border. Accordingly, this highly specialized cell membrane is endowed with an array of digestive enzymes and transporters for the final products of the intralumenal digestion (Maroux et al. Citation1988). To accommodate both these absorptive/digestive activities and fulfill the need to function as a permeability barrier against luminal pathogens, the brush border membrane is organized in lipid raft microdomains (Danielsen and Hansen Citation2006, Citation2008). In contrast to lipid rafts in other cell membranes that are typically dynamic and small (Coskun and Simons Citation2010), those of the brush border are large and stabilized by a high content of glycolipids and carbohydrate-crosslinking lectins, such as members of the galectin family and intelectin. Recently, a morphometric analysis of microvillar membranes revealed the presence of domains of increased thickness in the lipid bilayer, proposed to represent lipid rafts (Kunding et al. Citation2010). Interestingly, these domains were estimated to have a minimum size of about 600 nm2 and to be organized as ‘pinstripes’ along the length of the microvilli.
Conceivably, the high-throughput fluxes of amphiphilic molecules across the brush border that occur during absorption of the lipid components of the diet have the capability to modulate the fluidity and dynamic functioning of the membrane. Thus, fat absorption has been observed to induce a transient, clathrin-dependent selective endocytosis from the brush border of alkaline phosphatase (AP) (Hansen et al. Citation2007), a GPI-linked brush border enzyme that hydrolyzes monophosphate esters and resides in lipid raft microdomains (Danielsen Citation1995, Engle et al. Citation1995). This finding implies that the digestive/absorptive process involved in fat assimilation affects apical membrane trafficking in the enterocytes. Furthermore, it has long been known that dietary fat absorption is accompanied by a rise of intestinal AP in the serum (Keiding Citation1966, Young et al. Citation1981). This release of soluble AP to the blood has been shown to reflect its incorporation into basolaterally secreted surfactant-like particles which become deposited on the luminal surface and beneath the basal membrane of the epithelial cells (DeSchryver-Kecskemeti et al. Citation1989, Eliakim et al. Citation1989). Studies on AP knockout mice indicate a physiological role of this enzyme in a regulatory step of the fat absorption, and that metabolic abnormalities leading to hepatic steatosis and visceral fat accumulation may be caused by its absence (Nakano et al. Citation2006, Citation2007). Along these lines, intestinal AP has recently been proposed to play multiple biological roles in maintenance of intestinal homeostasis (Lalles Citation2010).
The aim of the present work was to study in closer detail the impact of free fatty acids on the enterocyte brush border membrane. By use of a mucosal explants culture system, we found that fluorescent BODIPY fatty acid analogs are rapidly taken up into the cytoplasm of the enterocytes. However, a fraction of the analogs was not transported further into the cell, but instead inserted directly into the brush border membrane, a process that occurs within a time frame of ∼5 s. In addition, short incubations of microvillar membrane vesicles with a fat mixture solution mimicking dietary mixed micelles rearranged the density profile of detergent resistant membranes (DRMs). In particular, a low-density population specifically enriched in AP was generated. We propose that this spontaneously occurring membrane remodeling of the brush border is the underlying cause of the selective endocytic trafficking of AP from the brush border membrane shown previously (Hansen et al. Citation2007) to accompany ingestion of fatty meals.
Materials and methods
Materials
4,4-difluoro-5-methyl-4-bora-3a, 4a-diaza-s-indacene-3-dodecanoic acid (BODIPY 500/510 C1, C12), 4,4-difluoro-5-octyl-4-bora-3a, 4a-diaza-s-indacene-3-pentanoic acid (BODIPY 500/510 C8, C5), secondary Alexa 594-conjugated anti-rabbit antibodies, and ProLong Gold antifade reagent with DAPI were purchased from Invitrogen (www.invitrogen.com), a rabbit antibody to intestinal alkaline phosphatase from AbD Serotec (www.biogenesis.co.uk/), a goat antibody to annexin A2 from Santa Cruz Biotechnology (www.scbt.com), a rabbit antibody to apolipoprotein B and secondary antibodies for immunoblotting and immunogold electron microscopy from DAKO (www.dako.dk), and bovine bile and pancreatin from porcine pancreas (containing a mixture of digestive enzymes) from Sigma-Aldrich (www.sigmaaldrich.com). A rabbit antibody to intestinal aminopeptidase N was previously described (Hansen et al. Citation1987). Pig small intestines were surgically removed from anaesthetisized animals by licensed staff of the Department of Experimental Medicine, the Panum Institute, Copenhagen, Denmark.
Organ culture of mucosal explants
Freshly obtained sections of jejunum were immediately rinsed in ice-cold RPMI-medium. Mucosal explants weighing ∼50 mg were rapidly excised, placed on stainless steel grids in culture dishes, and cultured at 37°C or 4°C in RPMI medium, essentially as described previously (Danielsen et al. Citation1982). For experiments with uptake of fluorescent fatty acid analogs, stock solutions of BODIPY 500/510 C1, C12 or BODIPY 500/510 C8, C5 in ethanol (5 mM) were prepared. The analogs were added to the culture medium to obtain a final concentration of 50 μM, and labeling was performed for periods of ∼5 s –10 min. In some experiments, the medium was then quickly replaced by analog-free medium (‘chase’), and further culture for 1 h.
Fluorescence microscopy
Immediately after organ culture, mucosal explants were briefly rinsed with fresh medium and fixed in 4% paraformaldehyde in 0.1 M sodium phosphate (PB), pH 7.2, for 2 h at 4°C. After a rinse in PB, they were frozen in precooled 2-methylbutane and sectioned in a Leica CM1850 cryostat at −20°C. For immunolabeling of aminopeptidase N and apolipoprotein B, sections were incubated for 1 h at room temperature with the primary antibodies (1:1000 and 1:200 dilution, respectively) in 50 mM Tris-HCl, 150 mM NaCl, 0.5% ovalbumin, 0.1% gelatine, 0.05% Tween 20, 0.2% teleostean gelatine, pH 7.2 (buffer A), followed by a second incubation for 1 h at room temperature with Alexa-conjugated antibodies to rabbit IgG (1:200 dilution in buffer A). Control experiments with omission of the primary antibodies were performed in all experiments. The labeled sections were mounted in antifade medium with DAPI and examined in a Leica DM 4000 B microscope fitted with a Leica DC 300 FX digital camera.
Preparation of microvillar membranes
Microvillar right-side-out membrane vesicles were prepared by the divalent cation precipitation method from pig jejunum (Booth and Kenny Citation1974). Briefly, the mucosa was scraped off and gently homogenized in a manually operated Potter-Elvehjem homogenizer in 2 mM Tris-HCl, 50 mM mannitol, pH 7.1, containing 10 μg/ml aprotinin and leupeptin. After centrifugation at 500 g, 5 min, MgCl2 was added to the supernatant to a final concentration of 10 mM. After 10 min incubation on ice, the preparation was centrifuged at 1500 g, 10 min, to pellet intracellular and basolateral membranes. A pellet of microvillar membrane vesicles was obtained by centrifugation of the supernatant at 48.000 g, 30 min.
Treatment of microvillar membranes with a fat mixture
A fat mixture mimicking a physiological solution of dietary mixed micelles was prepared by mixing rape oil (10%), containing 8% saturated, 63% monounsaturated, and 29% polyunsaturated fatty acids, bile (1%) and pancreatin (1%) in 25 mM HEPES-HCl, 150 mM NaCl, pH 7.1, (HB) and incubated overnight at room temperature. 50 μl of this mixture was then added to 450 μl of microvillar membrane vesicles in HB and incubated for 30 min at 37°C. The membranes were pelleted by centrifugation at 48,000 g, 20 min, resuspended in 1 ml of HB and immediately after subjected to detergent resistant membrane (DRM) analysis as described below. Control experiments were performed in parallel, where either none or only one of the components of the fat mixture (corn oil, bile or pancreatin) was present in the lipid solution used for incubation with the microvillar membrane vesicles.
DRM analysis
For DRM analysis, fat mixture-treated- and control microvillar membrane vesicles suspended in HB were extracted with 1% Triton X-100 for 10 min on ice. The extracts were then subjected to sucrose gradient ultracentrifugation as described previously (Danielsen Citation1995). For electron microscopy, the low-density floating fractions of the gradient were pooled, diluted five times with HB and centrifuged at 48,000 g, 1 h, to obtain a pellet of DRMs.
SDS/PAGE and immunoblotting
SDS/PAGE in 10% gels was performed according to Laemmli (Laemmli Citation1970). After electrophoresis and electrotransfer of proteins onto Immobilon PVDF membranes, immunoblotting was performed by incubation with antibodies to intestinal alkaline phosphatase, intestinal aminopeptidase N, and annexin A2, followed by incubation with horseradish peroxidase-conjugated secondary antibodies. The blots were developed by use of an electrochemiluminescence reagent, using a protocol supplied by the manufacturer (GE Healthcare, www.gehealthcare.com). After immunoblotting, total protein was visualized by staining with Coomassie brilliant blue R250 (0.2% dissolved in an ethanol/H2O/acetic acid mixture (50:43:7).
Electron microscopy
Pellets of DRMs were fixed in 2.5% glutaraldehyde in PB for 2 h at 4°C. After a rinse in PB they were postfixed in 1% osmium tetroxide in PB for 1 h at 4°C, dehydrated in acetone, and finally embedded in Epon. Ultrathin sections were cut in a LKB Ultratome III, and for some experiments, sections were incubated with antibodies to AP (dilution 1: 1000), followed by labeling with gold-conjugated secondary antibodies, as previously described (Hansen et al. Citation1992). Finally, the sections were examined in a Zeiss EM 900 electron microscope equipped with a Mega View II digital camera system.
Results
Free fatty acids rapidly insert into the enterocyte brush border membrane
Lipids, mostly in the form of triacylglycerol, provide a substantial caloric contribution to our daily diet, and following intralumenal emulsification with bile and digestion, primarily by pancreatic lipase, free fatty acids are released for enterocytic uptake (Iqbal and Hussain Citation2009, Mansbach and Siddiqi Citation2010). Intracellularly, they are reassembled with monoacylglycerol to form triacylglycerol in the endoplasmic reticulum, then assembled in chylomicrons, and finally discharged basolaterally to the lymph system.
Cellular uptake studies of free fatty acids have previously been performed using fluorescent BODIPY fatty acid analogs (Faergeman et al. Citation1997, Stahl et al. Citation1999). In BHK cells they were mainly (>90%) incorporated into phosphatidylcholine with small amounts also detected in other phosphoglycerides, but not in sphingomyelin (Kasurinen Citation1992). In addition, incorporation into tri- and diglycerides was observed, but not into cholesteryl esters, and over time, they accumulated in cytoplasmic lipid droplets. The overall length of both analogs used in the present study, BODIPY 500/510 C1, C12 and BODIPY 500/510 C8, C5, is equivalent to that of an 18-carbon fatty acid and they only differ in the position of the fluorophore relative to the carboxylate group. Organ culture of mucosal explants is an established model for in vivo-like intestinal uptake studies across the brush border (Danielsen et al. Citation1982), and as shown in , a widespread cytoplasmic fluorescence was evident in the enterocytes by 1 min of labeling with the fatty acid analog BODIPY 500/510 C1, C12. The labeling was most intense in the subapical part of the cytoplasm, indicating a rapid transfer from the medium into the cells. But in addition, a distinct labeling of the brush border was seen as well, separated from the cytoplasmic fluorescence by a narrow dark streak over the terminal web region situated just below the brush border. In contrast, no fluorescence was evident along the basolateral sides of the enterocytes, indicating that penetration through tight junctions did not occur, and goblet cells showed no labeling either. Double labeling with the brush border enzyme aminopeptidase N confirmed the presence of the fatty acid analog in the brush border membrane, and this labeling pattern remained largely unchanged after 10 min of incubation with the analog, and also after 1 h of ‘chase’ in analog-free medium (). The second fatty acid analog used, BODIPY 55/510 C8, C5, exhibited a similar labeling profile (data not shown).
Figure 1. Uptake of BODIPY fatty acid analogs into enterocytes. Mucosal explants were organ cultured in the presence of BODIPY 500/510 C1, C12 for the indicated periods of time. After culture and fixation, cryosections were labeled with antibodies to the brush border enzyme aminopeptidase N (ApN) as described in Methods. The arrows indicate the labeling of the brush border of four neighbouring enterocytes. In addition, the fatty acid analog mainly labeled the apical cytoplasm (C) of the enterocytes, whereas the enterocyte nuclei (N), goblet cells (G), and the underlying lamina propria (LP) were all devoid of labeling. Bars, 20 μm.
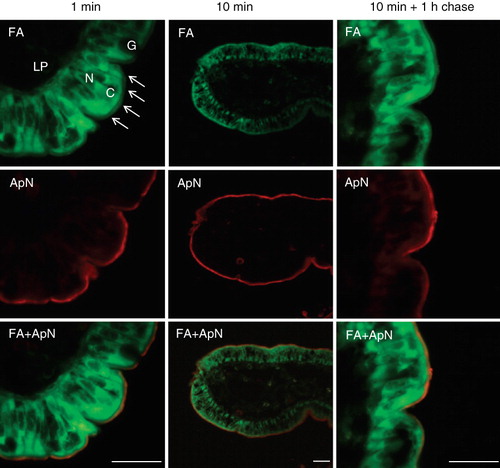
As shown in , apolipoprotein B was mainly localized in a few intracellular punctae in the enterocytes, likely to represent lipoproteins destined for secretion from the basolateral membrane (Iqbal and Hussain Citation2009). The fatty acid analog did not accumulate in these punctae, neither by 10 min of labeling or after 1 h of chase, indicating that it was not measurably incorporated into triacylglycerol within this period of time.
Figure 2. Localization of apolipoprotein B in enterocytes. Sections of mucosal explants, cultured in the presence of a BODIPY fatty acid analog for the indicated periods of time, were labeled with an antibody to apolipoprotein B. Labeling for this protein was mainly localized in intracellular punctae of the enterocytes, likely to represent lipoproteins. The fatty acid analog did not accumulate in the apolipoprotein B-positive punctae, neither by 10 min nor after 1 h of chase. Nuclei were visualized by DAPI staining in the merged images. Bars, 20 μm.
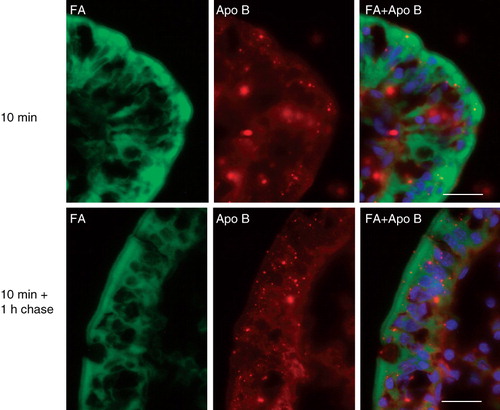
As shown in , cytoplasmic, as well as brush border labeling was clearly visible even after exposure to the fatty acid analog for only ∼5 s, indicative of an extremely rapid uptake. At the very short incubation periods the labeling of the brush border occasionally appeared dense and patchy.
Figure 3. Rapid insertion of BODIPY fatty acids into the brush border. Fluorescent images of short-term labeled explants. Labeling of the enterocyte cytoplasm and brush border was evident after only few seconds of exposure to the analog. The fluorescence frequently appeared patch or punctate. Bars, 20 μm.
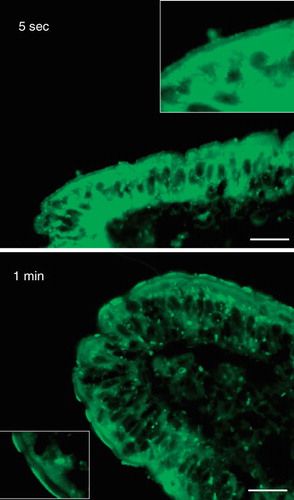
Collectively, the above results testify to the efficiency by which free fatty acids are taken up by enterocytes from the lumen of the gut. But in addition, they also show that a fraction of the luminal fatty acids are inserted into the brush border membrane rather than being directly transported into the cytoplasm. As judged by the unchanged relative labeling of the brush border over the time course studied, the fatty acids seemed to have a prolonged residency in the apical membrane. However, it cannot be excluded that incorporation of BODIPY fatty acids into phospholipids, notably phosphatidylcholine (Kasurinen Citation1992), may contribute to the brush border labeling seen after the 1 h chase period since this time period would be sufficient for phospholipid synthesis in the endoplasmic reticulum and subsequent membrane trafficking to the brush border (Danielsen Citation1982).
Dietary free fatty acids form microvillar DRMs enriched in alkaline phosphatase
When subjected to a DRM analysis, including extraction with ice-cold Triton X-100 followed by centrifugation through a 35–15% sucrose gradient, a substantial fraction of the microvillar membrane proteins appeared in the floating fractions of the gradient, reflecting that the brush border is composed of lipid raft microdomains (). For control membranes, the DRMs appeared mainly in the high-density part of the gradient (fractions 4–8) with only little material present in the low-density fractions (fractions 9–12), but the overall protein patterns of high- and low density DRMs were very similar. In contrast, microvillar membrane vesicles pretreated by incubation with a ‘physiological’ fat mixture, exhibited a strikingly different DRM pattern. Here, the four low density gradient fractions harboured most of the microvillar AP whereas the transmembrane aminopeptidase N and the peripheral annexin A2 were mainly found in the high density fractions (). This distribution change indicates that exposure to the fat mixture induces a remodeling of the brush border membrane that specifically sequesters AP in low-density DRMs. This remodeling was not observed when the microvillar membranes were pretreated with only one of the components of the fat mixture (i.e., either corn oil, bile or pancreatin), implying that the digestion products formed in the mixture, primarily free fatty acids, are the active components (data not shown).
Figure 4. DRM analysis of lipid-treated microvillar membrane vesicles. Microvillar membrane vesicles were prepared, treated with a fat mixture, extracted with Triton X-100, and fractionated by sucrose gradient centrifugation as described in Methods. In the control, most of the protein in DRMs, including aminopeptidase N (ApN), alkaline phosphatase (AP), and annexin A2 (An A2) were mainly seen in the high-density floating fractions (4–8). The fat mixture treatment caused a specific redistribution of AP to the low-density floating fractions (9–12).
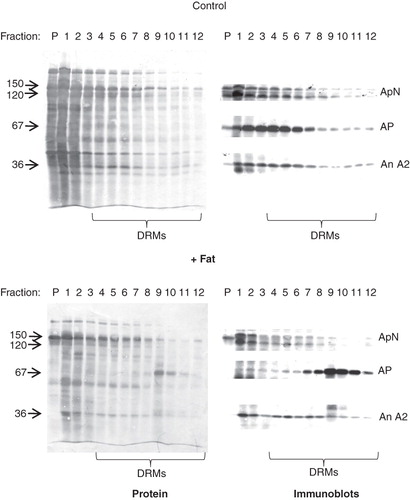
shows the morphology of the low-density DRMs (fractions 9–12) from control- and fat mixture-pretreated microvillar membrane vesicles. In the former, DRMs were very heterogeneous in size and pleiomorphic, and relatively few appeared vesicle-like. In contrast, DRMs from fat mixture-pretreated membranes were relatively small and more uniformly vesicle-like with a diameter of about 100 nm. By immunogold labeling, the latter clearly contained an increased amount of AP. These morphological changes thus confirm the notion that free fatty acids remodel the molecular composition of DRMs derived from the brush border membrane.
Figure 5. Morphology of microvillar low-density DRMs. Electron micrographs of low-density DRMs from control- and fat mixture-pretreated microvillar membranes. Whereas the former were relatively large and pleiomorphic, the latter were small and more uniformly vesicle-like. A markedly increased density of immunogold labeling for AP was seen after the fat treatment (C and D). Bars, 500 nm.
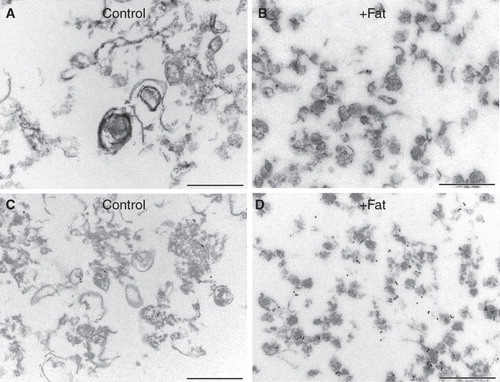
Discussion
Free fatty acids are generally not considered to be major components of cell membranes. But the reported levels present in brush border membranes usually exceed the trace amounts found in other tissues and have been suggested to represent, at least in part, exogenously-derived lipid accumulated during fat absorption (Proulx Citation1991). Thus, an analysis of the lipid composition of porcine microvillar membranes revealed the presence of 3.2% free fatty acids (Christiansen and Carlsen Citation1981), and we have previously observed an increase from 2.6% to 7.5% when mucosal explants were exposed to fat absorption (Hansen et al. Citation2007). The data obtained in the present work corroborate these earlier findings, but more importantly they indicate that the free fatty acids are selectively inserted into the lipid raft microdomains of the brush border. Furthermore, their insertion rearranges the protein distribution of these microdomains, most notably by creating low-density subdomains higly enriched in AP. As a GPI-anchored protein, AP resides more exclusively in DRMs than the other major brush border enzymes (for instance aminopeptidase N and sucrase-isomaltase) that are single-spanning transmembrane proteins and only partially insoluble in cold detergent (Danielsen Citation1995). From previous work, lipid rafts in the intestinal brush border are known to rely on the high content of glycolipids together with a number of cross-linking lectins, rather than on cholesterol (Danielsen and Hansen Citation2003, Citation2006). At present, we can only speculate as to why free fatty acids seem to insert preferentially near AP in these areas of the membrane. Conceivably, they may have a relative affinity for the glycosylphosphatidylinositol anchor of AP or, alternatively, be repelled from the transmembrane anchors of the other digestive enzymes of the brush border. In either case, subdomains of relative low density and enriched in AP will be generated.
Even in experiments with the shortest possible labeling time (∼5 s), BODIPY fatty acids were easily detected both in the cytoplasm and brush border of the enterocytes, indicating an extremely fast assimilation. Intriguingly, a consensus amongst researchers in the field concerning the passage of long-chain free fatty acids across cell membranes has yet to be established. Thus, as compelling arguments for both protein-dependent and protein-independent models have been presented, it is conceivable that both candidate lipid transporters (FABPs, FATPs, CD36, caveolin-1) as well as diffusion by a flip-flop mechanism may contribute to the overall uptake (Glatz et al. Citation2010). It seems reasonable to assume that the observed free fatty acid insertion in the lipid raft microdomains of the brush border stems from uptake via the latter mechanism. Here, the first step, binding to the outer leaflet (adsorption), is now considered to occur extremely fast, limited only by diffusion. The second step, the flip-flop from the outer to the inner leaflet with reorientation of the carboxyl head group from the outer to the inner lipid-water interface, is also thought to occur relatively fast (on a second scale) (Hamilton Citation2007, Glatz et al. Citation2010). This fast flip-flop rate is thought to rely on the local membrane environment that may shift the apparent pK a of the free fatty acid carboxyl group from ∼4.5 to ∼7.6, rendering about half the amount of fatty acids in the membrane uncharged. If correct, this model implies that the flip-flop rate and the ensuing desorption from the inner leaflet would be relatively slower from within a microdomain which stabilizes the ionized form of the free fatty acid, leading to a longer local membrane residency. Hypothetically, this scenario may explain the selective and seemingly stable insertion into the low-density, AP-enriched DRMs, and such a type of model was previously proposed to explain the role of caveolin-1 and cholesterol in transmembrane fatty acid movement across cell membranes (Meshulam et al. Citation2006). Finally, it is interesting to note that in a thirty year-old study on membrane perturbations induced by free fatty acids, it was concluded that saturated fatty acids partition into solid-like domains and stabilize them whereas cis-unsaturated fatty acids partition into fluid domains and disrupt their membrane packing (Klausner et al. Citation1980).
The lipid composition is a crucial parameter for cell membrane functioning, and for instance, it has long been known that the glycolipid content of the intestinal brush border increases both during development and crypt-villus differentiation, leading to a decrease in membrane fluidity (Proulx Citation1991). No doubt these changes occur to ensure that the brush border of mature enterocytes becomes a robust digestive/absorptive surface maximally resistant to lysis by bile and pancreatic enzymes as well as endocytic internalization of pathogens. Likewise, previous studies have documented that the lipid composition of brush border membranes can be altered, both directly by exposure to various lipids in vitro, as well as by dietary supplementation (Proulx Citation1991). Although a causal relationship has not been established, it seems fair to speculate that the formation of AP-enriched DRMs shown in the present work underlies the previously described selective endocytosis of brush border AP taking place during fat absorption (Hansen et al. Citation2007). Thus, the negative membrane curvature required for formation of an endocytic vesicle might be energetically more favourable by insertion in the outer leaflet of free fatty acids with the small carboxyl polar head group. Since the endocytosis is part of a physiological fat absorption-dependent process that leads to incorporation of AP into surfactant-like particles and ultimately to its release into the blood in vivo, the data presented here provide a possible link between fat absorption and these processes.
Declaration of interest: The work was supported by grants from the Beckett Foundation, the Augustinus Foundation, Aase og Ejnar Danielsens Fond, and Brødrene Hartmanns Fond. The authors report no conflicts of interest. The authors alone are responsible for the content and writing of the paper.
References
- Booth AG, Kenny AJ. 1974. A rapid method for the preparation of microvilli from rabbit kidney. Biochem J 142:575–581.
- Christiansen K, Carlsen J. 1981. Microvillus membrane vesicles from pig small intestine. Purity and lipid composition. Biochim Biophys Acta 647:188–195.
- Coskun U, Simons K. 2010. Membrane rafting: From apical sorting to phase segregation. FEBS Lett 584:1685–1693.
- Danielsen EM. 1982. Biosynthesis of intestinal microvillar proteins. Pulse-chase labelling studies on aminopeptidase N and sucrase-isomaltase. Biochem J 204:639–645.
- Danielsen EM. 1995. Involvement of detergent-insoluble complexes in the intracellular transport of intestinal brush border enzymes. Biochemistry 34:1596–1605.
- Danielsen EM, Hansen GH. 2003. Lipid rafts in epithelial brush borders: Atypical membrane microdomains with specialized functions. Biochim Biophys Acta 1617:1–9.
- Danielsen EM, Hansen GH. 2006. Lipid raft organization and function in brush borders of epithelial cells. Mol Membr Biol 23:71–79.
- Danielsen EM, Hansen GH. 2008. Lipid raft organization and function in the small intestinal brush border. J Physiol Biochem 64:377–382.
- Danielsen EM, Sjostrom H, Noren O, Bro B, Dabelsteen E. 1982. Biosynthesis of intestinal microvillar proteins. Characterization of intestinal explants in organ culture and evidence for the existence of pro-forms of the microvillar enzymes. Biochem J 202:647–654.
- DeSchryver-Kecskemeti K, Eliakim R, Carroll S, Stenson WF, Moxley MA, Alpers DH. 1989. Intestinal surfactant-like material. A novel secretory product of the rat enterocyte. J Clin Invest 84:1355–1361.
- Eliakim R, DeSchryver-Kecskemeti K, Nogee L, Stenson WF, Alpers DH. 1989. Isolation and characterization of a small intestinal surfactant-like particle containing alkaline phosphatase and other digestive enzymes. J Biol Chem 264:20614–20619.
- Engle MJ, Mahmood A, Alpers DH. 1995. Two rat intestinal alkaline phosphatase isoforms with different carboxyl-terminal peptides are both membrane-bound by a glycan phosphatidylinositol linkage. J Biol Chem 270:11935–11940.
- Faergeman NJ, DiRusso CC, Elberger A, Knudsen J, Black PN. 1997. Disruption of the Saccharomyces cerevisiae homologue to the murine fatty acid transport protein impairs uptake and growth on long-chain fatty acids. J Biol Chem 272:8531–8538.
- Glatz JF, Luiken JJ, Bonen A. 2010. Membrane fatty acid transporters as regulators of lipid metabolism: Implications for metabolic disease. Physiol Rev 90:367–417.
- Hamilton JA. 2007. New insights into the roles of proteins and lipids in membrane transport of fatty acids. Prostaglandins Leukot Essent Fatty Acids 77:355–361.
- Hansen GH, Niels-Christiansen LL, Immerdal L, Nystrom BT, Danielsen EM. 2007. Intestinal alkaline phosphatase: Selective endocytosis from the enterocyte brush border during fat absorption. Am J Physiol Gastrointest Liver Physiol 293:G1325–1332.
- Hansen GH, Sjostrom H, Noren O, Dabelsteen E. 1987. Immunomicroscopic localization of aminopeptidase N in the pig enterocyte. Implications for the route of intracellular transport. Eur J Cell Biol 43:253–259.
- Hansen GH, Wetterberg LL, Sjostrom H, Noren O. 1992. Immunogold labelling is a quantitative method as demonstrated by studies on aminopeptidase N in microvillar membrane vesicles. Histochem J 24:132–136.
- Iqbal J, Hussain MM. 2009. Intestinal lipid absorption. Am J Physiol Endocrinol Metab 296:E1183–1194.
- Kasurinen J. 1992. A novel fluorescent fatty acid, 5-methyl-BDY-3-dodecanoic acid, is a potential probe in lipid transport studies by incorporating selectively to lipid classes of BHK cells. Biochem Biophys Res Commun 187:1594–1601.
- Keiding NR. 1966. Intestinal alkaline phosphatase in human lymph and serum. Scand J Clin Lab Invest 18:134–140.
- Klausner RD, Kleinfeld AM, Hoover RL, Karnovsky MJ. 1980. Lipid domains in membranes. Evidence derived from structural perturbations induced by free fatty acids and lifetime heterogeneity analysis. J Biol Chem 255:1286–1295.
- Kunding AH, Christensen SM, Danielsen EM, Hansen GH. 2010. Domains of increased thickness in microvillar membranes of the small intestinal enterocyte. Mol Membr Biol 4–6:170–177.
- Laemmli UK. 1970. Cleavage of structural proteins during the assembly of the head of bacteriophage T4. Nature 227:680–685.
- Lalles JP. 2010. Intestinal alkaline phosphatase: Multiple biological roles in maintenance of intestinal homeostasis and modulation by diet. Nutr Rev 68:323–332.
- Mansbach CM, Siddiqi SA. 2010. The biogenesis of chylomicrons. Annu Rev Physiol 72:315–333.
- Maroux S, Coudrier E, Feracci H, Gorvel JP, Louvard D. 1988. Molecular organization of the intestinal brush border. Biochimie 70:1297–1306.
- Meshulam T, Simard JR, Wharton J, Hamilton JA, Pilch PF. 2006. Role of caveolin-1 and cholesterol in transmembrane fatty acid movement. Biochemistry 45:2882–2893.
- Nakano T, Inoue I, Koyama I, Kanazawa K, Nakamura K, Narisawa S, Tanaka K, Akita M, Masuyama T, Seo M, Hokari S, Katayama S, Alpers DH, Millan JL, Komoda T. 2007. Disruption of the murine intestinal alkaline phosphatase gene Akp3 impairs lipid transcytosis and induces visceral fat accumulation and hepatic steatosis. Am J Physiol Gastrointest Liver Physiol 292:G1439–1449.
- Nakano T, Shimanuki T, Matsushita M, Koyama I, Inoue I, Katayama S, Alpers DH, Komoda T. 2006. Involvement of intestinal alkaline phosphatase in serum apolipoprotein B-48 level and its association with ABO and secretor blood group types. Biochem Biophys Res Commun 341:33–38.
- Proulx P. 1991. Structure-function relationships in intestinal brush border membranes. Biochim Biophys Acta 1071:255–271.
- Stahl A, Hirsch DJ, Gimeno RE, Punreddy S, Ge P, Watson N, Patel S, Kotler M, Raimondi A, Tartaglia LA, Lodish HF. 1999. Identification of the major intestinal fatty acid transport protein. Mol Cell 4:299–308.
- Young GP, Friedman S, Yedlin ST, Allers DH. 1981. Effect of fat feeding on intestinal alkaline phosphatase activity in tissue and serum. Am J Physiol 241:G461–468.