Abstract
The antimicrobial property of stabilized silver nanoparticles (AgNPs) with phospholipid membrane was investigated on both Gram-negative (Escherichia coli) and Gram-positive (Staphylococcus aureus) bacterial strains. The influence of phospholipid concentrations on antibacterial kinetics actions of AgNPs was studied with two different methodologies in order to understand the bactericidal and bacteriostatic effects. The bacterial inactivation of synthesized AgNPs fitted well to the Chick-Watson model with a high regression coefficient, R2 > 0.91. The antibacterial properties of AgNPs depend on the particle size, stabilizer and lecithin concentrations. Only the stabilized AgNPs that have the Klec/Ag values of 1 and 2 presented the inhabitation zone, while unstabilized AgNPs agglomerated quickly, settled on the wells and did not diffuse in agar. In addition, the specific coefficient of lethality depends on the lecithin concentration. An increase in lecithin concentration caused multilayer creation on the AgNPs' surface and reduced the release of AgNPs which led to low bacterial killing rate.
Introduction
As physical, chemical and biological properties of metal nanocomposites can be improved in nanoscale, many researchers have paid great attention on their synthesis and application for specific purposes. Nano-sized metals have special characteristics that can be used for several advanced applications (Carotenuto and Nicolais Citation2004, Majewski and Thierry Citation2007, Dong et al. Citation2009).
Recent studies have shown that metal nanocomposites are effective biocides against bacteria (Chen and Chiang Citation2008, Falletta et al. Citation2008, Hernández-Sierra et al. Citation2008, Dastjerdi et al. Citation2010, Marambio-Jones and Hoek, Citation2010), fungi (Kim et al. Citation2007, Citation2009), and viruses (Elechiguerra et al. Citation2005, Zodrow et al. Citation2009). The antibacterial properties of silver nanocomposites (Bakumov et al. Citation2007, Dastjerdi et al. Citation2009), silver ion (Woo et al. Citation2008, Barani et al. Citation2010), and silver compounds (Fisher et al. Citation2003, Silver et al. Citation2006) have been thoroughly investigated.
Silver is an ‘oligodynamic’ agent because of its capacity to have a bactericidal effect at very low concentrations (Collart et al. Citation2006). Results of studies on antibacterial properties of silver nanoparticles proposed that silver nanoparticles may attach to the cell membrane surface, which causes change in cell permeability and finally cell death (Rai et al. Citation2009). Moreover, there is a possibility of their penetration into the bacteria, resulting in cell death (Morones et al. Citation2005). Thus, the smaller silver nanoparticles have the larger available surface area for binding to the cell membrane surface of bacteria.
The presented models for disinfection are empirically (Hassen et al. Citation2000) and many of them have been proposed for disinfection kinetics (Hom Citation1972, Selleck et al. Citation1978, Severin et al. Citation1984, Haas and Joffe Citation1994). The simplest disinfection model was proposed by Chick and Watson. In the Chick-Watson model, the rate of inactivation of a microorganism is dependent on the silver nanoparticles concentration and contact time (Cho et al. Citation2003).
Several methods have been employed to synthesize silver nanoparticles among which, the chemical-reduction method has been extensively investigated because of its simplicity of performance and mild conditions (Zhang et al. Citation2007). Usually, the chemical reduction is composed of silver nitrate as a precursor, reducing agent, and stabilizer. The stabilizing agents are used to separate the particles to prevent aggregation (Chou and Lai Citation2004). Moreover, phospholipids as a stabilizer are used to improve the biocompatibility of synthesized metal nanoparticles (Chung et al. Citation2008, Barani et al. Citation2010). Park et al reported the membrane fluidity of loaded nanoparticles to the liposome (Park et al. Citation2005, Citation2006), while the synthesis of nanoparticles in phospholipid membrane had been reported already. In this study, the effect of phospholipid concentrations on the antibacterial and killing kinetic of two Gram-positive and Gram-negative bacteria was studied and the Chick-Watson model was adopted for evaluation of AgNPs disinfection kinetics.
Materials
All the chemicals and reagents used were of analytical grade. Distilled water was used throughout the work. Silver nitrate (AgNO3 extra pure, >99.8%), sodium borohydride (NaBH4, 99.9%), sodium chloride (99.99%), CASO agar, Mueller-Hinton agar, and CASO broth were purchased from Merck Company (Germany). Lecithin was used as a biocompatible agent for stabilizing silver nanoparticles and was received as a gift from Lipoid Company (Lipoid S 75; Germany).
Bacteria and culture condition
Staphylococcus aureus ATCC 6538 as a Gram-positive bacterium and Escherichia coli ATCC 8739 as a Gram-negative bacterium were used in this study. The test strains were maintained as frozen stocks at −70°C in PBS (phosphate buffered saline) containing 20% (v/v) glycerol. Fresh cultures were prepared by inoculating 100 μl aliquots of the thawed microbial stock suspensions into CASO agar (Merck, Germany) plates followed by overnight incubation at 35°C. The bacteria inocula were prepared by suspending overnight colonies from CASO agar medium in 0.9% saline. The inocula were adjusted photometrically at 600 nm to a cell density equivalent to 0.5 McFarland standard (1.5 × 108 CFU/ml) before use.
Synthesis of AgNPs
Preparation of AgNPs was carried out according to our previous reported method (Barani et al. Citation2010). Nano liposome was prepared by heating method. The prepared lecithin solution (200 ppm, 50 ml) was stirred vigorously (approximately 1500 rpm) in a special designed bottle (Colas et al. Citation2007) for 30 min at 70°C and then cooled to room temperature. Then, the reducing agent with an amount of twice the concentration of silver nitrate solution was added, while stirring on a hotplate stirrer for 15 min. The mixture of reducing agent and liposome colloidal solution was cooled to 0°C, because the reducing agent is only activated by the reaction of silver salt according to Equation (1), and not by heating (Zhang et al. Citation2006).
The complete hydrolysis of the sodium borohydride can be rapidly achieved by the rise of temperature or by adding acids in aqueous solution (Schlesinger et al. Citation1953). Finally, the 10 ml of prepared silver nitrate (100 pm) was added to colloidal solution dropwise at a rate of 1 ml/min while stirring at 0°C. Adding silver nitrate to the reducing agent solution leads to the reaction in Equation (2) and changes the colour of the solution from clear transparent to dark yellow indicating synthesis of silver nanoparticles.
The KLec/Ag ([Lecithin]/[AgNO3]), the lecithin to silver nitrate molar concentration ratio, is the influencing factor in synthesis of silver nanoparticles. The KLec/Ag values were 2 × 10-3, 0.2, 1 and 2 while the micelles concentration (CMC) of lecithin is 5 × 10-9 molar (Barenholz and Lasic Citation1996). To obtain particles with small size (Petit et al. Citation1993), concentration of the reducing agent was chosen twice as the precursor (KNaBH4/Ag = 2). The remaining reducing agent in the solution of synthesized silver nanoparticles was decomposed by heating the colloidal nanoparticles solution to about 50°C (Wang et al. Citation1999).
Characterization of AgNPs
The UV absorption spectra of all AgNPs solutions were determined on an Optizen 2120UV spectrophotometer with 2 nm resolution at room temperature. The spectrum wavelengths were recorded between 200 and 700 nm and a glass cuvette with 1 cm optical path was used. The studies of size and morphology of the nanoparticles were performed by transmission electron microscopy (TEM) using Philips CM120 microscope operating at up to 120 KV. Samples for TEM studies were prepared by placing drops of the silver nanoparticles solutions on carbon-coated TEM grids and allowing the solvent to slowly evaporate under vacuum for 30 min at room temperature.
Antibacterial studies
Minimum inhibitory concentration (MIC)
MIC was determined by both agar well diffusion and broth dilution methods. In the well diffusion method, the surface of Petri dishes containing 25 ml of Mueller-Hinton agar was seeded individually with bacterial suspensions (108 CFU/ml) with a sterile cotton swab. Wells with 7 mm diameter were created by punching a stainless steel cylinder on to the agar plates and removing the agar to form a well. Finally, 80 μl aliquots of each prepared sample (100, 50, 25, 12.5, and 6.25 ppm) were placed individually in two wells. The prepared Petri dishes were incubated at 35°C for 20 h, and diameters of inhibition zones (i.e., areas surrounding the test samples where bacteria growth is inhibited or not) were determined.
In the broth dilution method, the bacterial suspension was added to the tubes containing various concentrations of silver nano composites solutions (100, 50, 25, 12.5, and 6.25 ppm) in Mueller-Hinton broth to reach the final concentration of about 106 CFU/ml. Tubes were incubated at 35°C for 20 h. Then, 1 ml of each tube was added to the 9 ml of neutralizing solution (0.6% sodium thioglycolate and 1% sodium thiosulphate) and the total bacterial count was determined by pour plate method using CASO agar medium. The minimum inhibitory concentration (MIC) was defined as the minimum concentration of the antibacterial agent that inhibits the growth of tested microorganism. The minimum bactericidal concentration (MBC) was defined as the minimum antibacterial concentration that kills more than 99.9% of the first bacterial inocula.
Bacterial killing kinetic
It was assessed without nutrient medium. For this purpose, 40 ml of AgNPs solutions (100 ppm) were inoculated with bacterial suspension at a final concentration of about 105 CFU/ml. Then 1 ml aliquots were removed at different intervals of 0, 5, 10, 20, 30, 45, 60, 90, and 120 min which were added to 9 ml of neutralizing solution. Total bacterial count was determined by pour plate method using CASO agar medium.
Results and discussion
UV-visible spectrum analysis
Localized surface plasmon resonances of AgNPs depend on the size, shape, as well as the surrounding dielectric medium. Usually the spherical nanoparticles in the range of 10–50 nm show an extinction peak at ∼400 nm, leading to a characteristic yellow colour (Cobley et al. Citation2009) and changing the size of spherical particles can induce small shifts in the peak position. shows the UV absorption of synthesized AgNPs, which is capped with phospholipids membrane. The fresh synthesized silver nanoparticles have a yellow colour which has higher absorption than the capped AgNPs. But they have a short lifetime and their colour changes to dark yellow, and then black suspension and slowly turn to macro particle and settle. AgNPs have a localized peak at 405 nm and their colour shift to darker by adding lecithin which causes their absorption to reduce (Barani et al. Citation2010). This is because of the lack of absorption for lecithin suspension at this position.
Transmission electron microscopy studies
The morphology of AgNPs was further proved by recording TEM of prepared silver colloidal suspension. The TEM images of synthesized AgNPs are presented in . The silver nanoparticles which were not stabilized by phospholipids membrane agglomerated and created large particles, which linked to one another and created sediments in range of micrometer (). By adding the lecithin stabilizer (KLec/Ag = 0.2) spherical AgNPs () with a size of 5–19 nm and mean value of 12.06 nm were synthesized.
Figure 2. TEM images of prepared AgNPs with various lecithin concentrations: (a) Klec/Ag = 0; synthesized AgNPs have a short life without stabilizer and linked one to another and appear agglomerated silver particles; (b) Klec/Ag = 0.2, and (c) Klec/Ag = 2; lecithin molecules capped AgNPs and prevent them to link to one another.
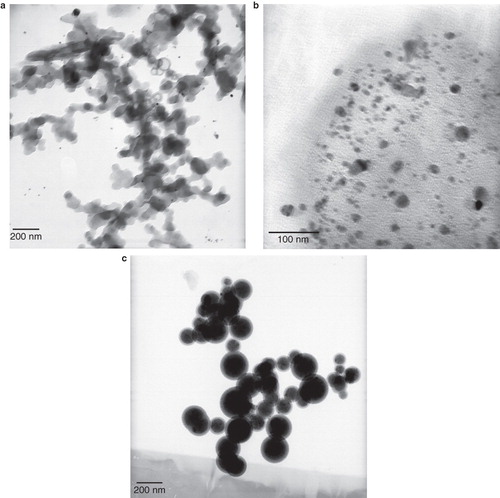
presents the size distribution of these AgNPs, which have a narrow size distribution. The spherical and narrow size distribution of AgNPs were also confirmed by the narrow and sharp UV absorption peak at 405 nm. Higher amount of stabilizer led to synthesis of AgNPs in a range of 75–110 nm and mean value of 83.84 nm (). With a high lecithin concentration, liposome nanoparticles were created in the mean size of 84 nm, and AgNPs were entrapped in them ().
Antibacterial of AgNPs
As well as the synthesis of AgNPs, the antibacterial property of AgNPs against two Gram-positive and Gram-negative bacteria was also analyzed. shows MIC and MBC of synthesized AgNPs with KLec/Ag = 2. The ratio of MBC/MIC is a feature which can show the bactericidal ability of antibacterial agents (Ayala-Núñez et al. Citation2009). The synthesized AgNPs in the phospholipids membranes with KLec/Ag = 2 had a ratio of 1.6 and 2 for E. coli and S. aureus, respectively. The smaller ratio suggests that a little amount of antibacterial agent is needed to reach the bactericidal effect. Therefore, the synthesized AgNPs with a ratio lower than 2 could be considered as a bactericidal agent (Ayala-Núñez et al. Citation2009).
Table I. Mean value of MIC and MBC for synthesized AgNPs at phospholipid membrane with the ratio of KLec/Ag = 2 and determined by broth dilution method. Experiments were performed in duplicates.
shows the bacterial inhibition zones at various silver contents for two samples with Klec/Ag values of 1 and 2, while the other ratios did not show any bacterial inhibition zones. Results indicated that AgNPs demonstrate higher inhibition zone diameter against S. aureus than E. coli which was confirmed by other researchers (Cho et al. Citation2005, Prema and Raju Citation2009, Barani et al. Citation2010). So, it can be suggested that the antibacterial effects of AgNPs can be associated with types of bacterial species. It is hypothesized that the AgNPs with smaller particles size can easily penetrate to the agar medium and can absorb to the cell surface of bacteria which result in higher antibacterial activity (Morones et al. Citation2005). However, the large AgNPs at ratio lower than 1 did not show the antibacterial properties. The size of nanoparticles ensures that a significantly large surface area of the particles is in contact with the bacterial cell membrane. Considering a spherical particles of uniform size on a theoretical case, a decrease in the particle size from 10 μm to 10 nm will increase the contact surface area by 106 times. Also, inhibition depends on the AgNPs concentrations, which the stabilized AgNPs at lower 6.25 ppm did not prove the bacterial inhibition zones (Prema and Raju Citation2009).
Table II. Mean value of inhibition zone diameter of AgNPs with various amounts of lecithin. Experiments were performed in duplicates.
When the KLec/Ag value was lower than 1, the inhibition zone was not remarkable in both agar plates containing E. coli and S. aureus (). The results suggested that the AgNPs were not stable at lower concentrations of lecithin which leads to agglomeration and sedimentation of AgNPs and not to diffuse to the agar plates (Lee et al. Citation2010). shows that silver nanoparticles were settled in the wells and cannot diffuse to the agar medium. According to the results, it can be assumed that the antibacterial property of AgNPs depends on silver concentration, lecithin concentration, and bacterial species.
Bacterial killing kinetics:
The antibacterial kinetics of AgNPs were determined in the aqueous media and nutrition broth by counting the number of colony units. The kinetics reduction of bacterial colonies was calculated according to Equation (3):
where N is the number of CFU per milliliter of solution at any defined time, and N0 is the initial number of CFU per milliliter of solution at the beginning of test. shows the percentage of E. coli and S. aureus reduction at various KLec/Ag values at 100 ppm concentrations during 120 min. It shows that increasing of KLec/Ag value led to lower bacterial killing rate. This means that higher value of lecithin causes slow release of silver nanoparticles to the medium which leads to longer bacterial inactivation time.
Figure 5. Bacterial inactivation Kinetics of (a) S. aureus and (b) E. coli which is calculated according to R% = (N0−N)/N0 × 100 during 120 min, in which N, and N0 are the number of CFU per milliliter of solution at the defined and initial time, respectively.
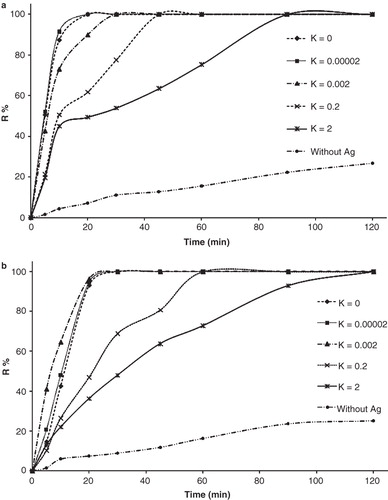
The buffer testing media does not support the bacterial growth. The bacterial suspension at all conditions has a decline trend in the number of bacteria during 120 min. Furthermore, the bacterial suspension without AgNPs also showed a decline trend, which was not significant.
Lecithin molecule is a safe biological agent which capped the AgNPs with a bilayer structure. , shows the formation of phospholipids membrane on the surface of silver nanoparticles. The presence of lecithin molecule bilayer leads to reduce the direct contact of AgNPs to the bacterial cell membrane which results in lower killing rate of AgNPs. The silver nanoparticles should diffuse through the bilayer and contact to the bacterial cell membrane and cause the cell death. The killing rate is more decreased by an increase in lecithin concentration which leads to formation of multilayer structure on the AgNPs surface and prolong reaching time to the bacterial cell membrane.
Figure 6. Schematic molecular of lecithin which is formed as a bilayer membrane on the AgNPs surface.
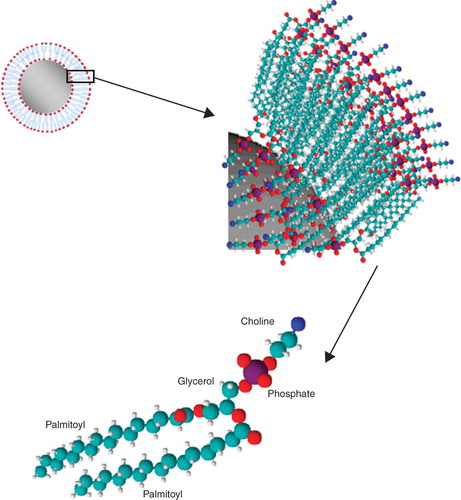
The higher amount of Klec/Ag resulted in the lowest killing rate because of slower release rate of AgNPs. The slow release of AgNPs from phospholipid membranes can be achieved by high amount of lecithin which is due to larger nanocomposites (Barani et al. Citation2010) and leads to slow release. In comparison, the silver nanoparticles without stabilizer killed all bacteria within 20 min at KLec/Ag = 2; this time was prolonged to 90 and 120 min for S. aureus () and E. coli (), respectively, because the AgNPs had direct contact to the bacterial cell membrane and could easily disturb its permeability.
The killing kinetic of bacteria can be shown according to the Chick-Watson equation (Kwok-Keung and LeChevallier Citation2004):
where N is the number of CFU per milliliter of solution at the time t, N0 is the initial number of CFU per milliliter of solution at the beginning of the test, C is the concentration of applied AgNPs colloidal solution and K is the specific coefficient of lethality. The killing rate at specific concentrations can be shown as Equation (4). The specific coefficient of lethality was calculated according to Equation (4) for 100 ppm concentration of AgNPs colloidal solution and presented in . The linear regression was applied for fitting a mathematical model to the data. The existed models have a good correlation to the experimental data with a high regression coefficient (R2 > 0.91) for all samples.
Figure 7. The specific coefficient of lethality of colloidal AgNPs solution (100 ppm) on S. aureus and E. coli is obtained by adopting the bacterial inactivation data to the Chick-Watson model by linear regression. The inset numbers on each bar show the linear regression coefficient.
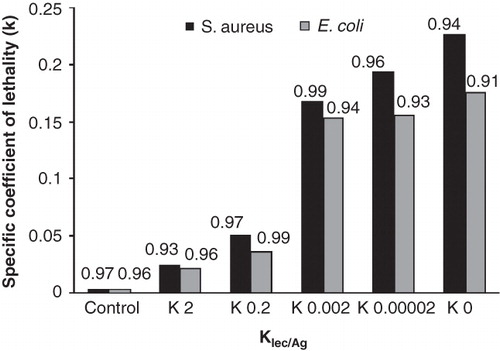
The specific coefficient of lethality of AgNPs increases with a decrease in lecithin concentration which increases their direct contact to the bacterial cell membrane. The AgNPs without lecithin had the highest specific coefficient of lethality because of high direct contact of AgNPs to bacterial cell membrane. In addition, this coefficient on S. aureus is higher than E. coli, which shows that these bacteria are inactivated quicker than E. coli in presence of AgNPs.
Conclusion
The synthesized AgNPs colloidal solution had a localized peak at 405 nm indicating the presence of spherical structure. Moreover, this was also confirmed by the TEM micrographs. The synthesized AgNPs without stabilizer had a short shelf life and agglomerated quickly, linked to each other and created large particles. Lecithin molecules as a stabilizer separated the AgNPs and prevented their agglomeration. The stabilized AgNPs only presented antibacterial properties in agar method due to their small size. The MBC/MIC ratio of stabilized AgNPs was low which indicated the capacity of bactericidal effects of these nanoparticles. The sample with lower KLec/Ag did not show antibacterial properties at various concentrations of silver in the well diffusion test because of less stability of colloidal silver nanoparticles. The bacteria reduction by AgNPs in the buffer solution was well described by the Chick-Watson model. The specific coefficient of lethality was calculated from this model with a high regression coefficient. The specific coefficient of lethality was dependant on the lecithin concentration. An increase in lecithin concentration, as a phospholipids membrane stabilizer, caused slower release of silver and prolonged the time for total bacterial inactivation.
Declaration of interest: The authors report no conflicts of interest. The authors alone are responsible for the content and writing of the paper.
References
- Ayala-Núñez N, Lara Villegas H, del Carmen Ixtepan Turrent L, Rodríguez Padilla C. 2009. Silver nanoparticles toxicity and bactericidal effect against methicillin-resistant staphylococcus aureus: Nanoscale does matter. Nanobiotechnology 5(1):2–9.
- Bakumov V, Gueinzius K, Hermann C, Schwarz M, Kroke E. 2007. Polysilazane-derived antibacterial silver-ceramic nanocomposites. J Eur Ceramic Soc 27(10):3287–3292.
- Barani H, Montazer M, Toliyat T, Samadi N. 2010. Synthesis of Ag-liposome nano composites. J Liposome Res 20(4):323–329.
- Barenholz Y, Lasic DD. 1996. From design to microreactors. Boca Raton, FL: CRC Press.
- Carotenuto G, Nicolais L. 2004. Synthesis and characterization of gold-based nanoscopic additives for polymers. Composites Part B: Engineering 35(5):385–391.
- Chen C-Y, Chiang C-L. 2008. Preparation of cotton fibers with antibacterial silver nanoparticles. Materials Lett 62(21–22):3607–3609.
- Cho K-H, Park J-E, Osaka T, Park S-G. 2005. The study of antimicrobial activity and preservative effects of nanosilver ingredient. Electrochim Acta 51(5):956–960.
- Cho M, Chung H, Yoon J. 2003. Disinfection of water containing natural organic matter by using ozone-initiated radical reactions. Appl Environ Microbiol 69(4):2284–2291.
- Chou K-S, Lai Y-S. 2004. Effect of polyvinyl pyrrolidone molecular weights on the formation of nanosized silver colloids. Materials Chem Phys 83(1):82–88.
- Chung Y-C, Chen IH, Chen C-J. 2008. The surface modification of silver nanoparticles by phosphoryl disulfides for improved biocompatibility and intracellular uptake. Biomaterials 29(12):1807–1816.
- Cobley C, Skrabalak S, Campbell D, Xia Y. 2009. Shape-controlled synthesis of silver nanoparticles for plasmonic and sensing applications. Plasmonics 4(2):171–179.
- Colas J-C, Shi W, Rao VSNM, Omri A, Mozafari MR, Singh H. 2007. Microscopical investigations of nisin-loaded nanoliposomes prepared by Mozafari method and their bacterial targeting. Micron 38(8):841–847.
- Collart D, Kepner B, Mehrabi S, Robinson L, Mintz EA. 2006. Efficacy of oligodynamic metals in the control of bacteria growth in humidifier water tanks and mist droplets. J Water Health 4(2):149–156.
- Dastjerdi R, Montazer M, Shahsavan S. 2009. A new method to stabilize nanoparticles on textile surfaces. Colloids Surfaces A: Physicochem Engineer Asp 345(1–3):202–10.
- Dastjerdi R, Montazer M, Shahsavan S. 2010. A novel technique for producing durable multifunctional textiles using nanocomposite coating. Colloids Surfaces B: Biointerfaces 81(1):32–41.
- Dong J, Xu Z, Kuznicki SM. 2009. Magnetic multi-functional nano composites for environmental applications. Adv Function Materials 19(8):1268–1275.
- Elechiguerra J, Burt J, Morones J, Camacho-Bragado A, Gao X, Lara H, Yacaman M. 2005. Interaction of silver nanoparticles with HIV-1. J Nanobiotechnol 3(1):6.
- Falletta E, Bonini M, Fratini E, Lo Nostro A, Pesavento G, Becheri A, Lo Nostro P, Canton P, Baglioni P. 2008. Clusters of poly(acrylates) and silver nanoparticles: Structure and applications for antimicrobial fabrics. J Phys Chem C 112(31):11758–11766.
- Fisher NM, Marsh E, Lazova R. 2003. Scar-localized argyria secondary to silver sulfadiazine cream. J Am Acad Dermatol 49(4):730–732.
- Haas CN, Joffe J. 1994. Disinfection under dynamic conditions: Modification of Hom's model for decay. Environ Sci Technol 28(7):1367–1369.
- Hassen A, Mahrouk M, Ouzari H, Cherif M, Boudabous A, Damelincourt JJ. 2000. UV disinfection of treated wastewater in a large-scale pilot plant and inactivation of selected bacteria in a laboratory UV device. Bioresource Technol 74(2):141–150.
- Hernández-Sierra JF, Ruiz F, Cruz Pena DC, Martínez-Gutiérrez F, Martínez AE, de Jesús Pozos Guillén A, Tapia-Pérez H, Martínez Castañón G. 2008. The antimicrobial sensitivity of Streptococcus mutans to nanoparticles of silver, zinc oxide, and gold. Nanomed: Nanotechnol, Biol Med 4(3):237–240.
- Hom LW. 1972. Kinetics of chlorine disinfection in an ecosystem. ASCE J Sanit Eng Div 98(SA1):183–194.
- Kim JS, Kuk E, Yu KN, Kim J-H, Park SJ, Lee HJ, Kim SH, Park YK, Park YH, Hwang C-Y, Kim Y-K, Lee Y-S, Jeong DH, Cho M-H. 2007. Antimicrobial effects of silver nanoparticles. Nanomed: Nanotechnol, Biol Med 3(1):95–101.
- Kim K-J, Sung W, Suh B, Moon S-K, Choi J-S, Kim J, Lee D. 2009. Antifungal activity and mode of action of silver nano-particles on Candida albicans. BioMetals 22(2):235–242.
- Kwok-Keung AU, LeChevallier MW. 2004. Water treatment and pathogen control: Process efficiency in achieving safe drinking-water (WHO Drinking-Water Quality). London: IWA Publishing.
- Lee S, Song K, Lee B. 2010. Antibacterial activity of silver nanoparticles prepared by a chemical reduction method. Korean J Chem Engineer 27(2):688–692.
- Majewski P, Thierry B. 2007. Functionalized magnetite nanoparticles – synthesis, properties, and bio-applications. Crit Rev Solid State Materials Sci 32(3):203–215.
- Marambio-Jones C, Hoek E. 2010. A review of the antibacterial effects of silver nanomaterials and potential implications for human health and the environment. J Nanoparticle Res 12(5):1531–1551.
- Morones JR, Elechiguerra JL, Camacho A, Holt K, Kouri JB, Ramírez JT, Yacaman MJ. 2005. The bactericidal effect of silver nanoparticles. Nanotechnology 16(10):2346–2353.
- Park S-H, Oh S-G, Mun J-Y, Han S-S. 2005. Effects of silver nanoparticles on the fluidity of bilayer in phospholipid liposome. Colloids Surfaces B: Biointerfaces 44(2–3):117–122.
- Park S-H, Oh S-G, Mun J-Y, Han S-S. 2006. Loading of gold nanoparticles inside the DPPC bilayers of liposome and their effects on membrane fluidities. Colloids Surfaces B: Biointerfaces 48(2):112–118.
- Petit C, Lixon P, Pileni MP. 1993. In situ synthesis of silver nanocluster in AOT reverse micelles. J Phys Chem 97(49):12974–12983.
- Prema P, Raju R. 2009. Fabrication and characterization of silver nanoparticle and its potential antibacterial activity. Biotechnol Bioprocess Engineer 14(6):842–847.
- Rai M, Yadav A, Gade A. 2009. Silver nanoparticles as a new generation of antimicrobials. Biotechnol Adv 27(1):76–83.
- Schlesinger HI, Brown HC, Finholt AE, Gilbreath JR, Hoekstra HR, Hyde EK. 1953. Sodium borohydride, its hydrolysis and its use as a reducing agent and in the generation of hydrogen1. J Am Chem Soc 75(1):215–219.
- Selleck RE, Saunier BM, Collins HF. 1978. Kinetics of bacterial deactivation with chlorine. J Environ Eng Div Am Soc Civ Eng 104(6 Ee6):1197–1212.
- Severin BF, Suidan MT, Engelbrecht RS. 1984. Series-event kinetic model for chemical disinfection. J Environ Engineer 110(2):430–439.
- Silver S, Phung L, Silver G. 2006. Silver as biocides in burn and wound dressings and bacterial resistance to silver compounds. J Industr Microbiol Biotechnol 33(7):627–634.
- Wang W, Chen X, Efrima S. 1999. Silver nanoparticles capped by long-chain unsaturated carboxylates. J Phys Chem B 103(34):7238–7246.
- Woo KJ, Hye CK, Ki WK, Shin S, So HK, Yong HP. 2008. Antibacterial activity and mechanism of action of the silver ion in Staphylococcus aureus and Escherichia coli. Appl Environ Microbiol 74(7):2171–2178.
- Zhang Q, Smith G, Wu Y, Mohring R. 2006. Catalytic hydrolysis of sodium borohydride in an auto-thermal fixed-bed reactor. Int J Hydrogen Energy 31(7):961–965.
- Zhang W, Qiao X, Chen J. 2007. Synthesis of silver nanoparticles – effects of concerned parameters in water/oil microemulsion. Mater Sci Engineer B 142(1):1–15.
- Zodrow K, Brunet L, Mahendra S, Li D, Zhang A, Li Q, Alvarez PJJ. 2009. Polysulfone ultrafiltration membranes impregnated with silver nanoparticles show improved biofouling resistance and virus removal. Water Res 43(3):715–723.