Abstract
Previous studies have shown that certain saturated lipids protect red blood cells (RBCs) during hypothermic storage but provide little protection during freezing or freeze-drying, whereas various unsaturated lipids destabilize RBCs during hypothermic storage but protect during freezing and freeze-drying. The protective effect of liposomes has been attributed to membrane modifications. We have previously shown that cholesterol exchange and lipid transfer between liposomes composed of saturated lipids and RBCs critically depends on the length of the lipid acyl chains. In this study the effect of unsaturated lipids with differences in their number of unsaturated bonds (18:0/18:1, 18:1/18:1, 18:2/18:2) on RBC membrane properties has been studied. RBCs were incubated in the presence of liposomes and both the liposomal and RBC fraction were analyzed by Fourier transform infrared spectroscopy (FTIR) after incubation. The liposomes caused an increase in RBC membrane conformational disorder at suprazero temperatures. The fluidizing effect of the liposomes on the RBC membranes, however, was found to be similar for the different lipids irrespective of their unsaturation level. The gel to liquid crystalline phase transition temperature of the liposomes increased after incubation with RBCs. RBC membrane fluidity increased linearly during the first 8 hours of incubation in the presence of liposomes. The increase in RBC membrane fluidity was found to be temperature dependent and displayed Arrhenius behaviour between 20 and 40°C, with an activation energy of 88 kJ mol-1. Taken together, liposomes composed of unsaturated lipids increase RBC membrane conformational disorder, which could explain their cryoprotective action.
Abbreviations: | ||
DLPC, | = | 1,2-dilinoleoyl-sn-glycero-3-phosphocholine |
DOPC, | = | dioleoyl-sn-glycero-3-phosphocholine |
Egg-PC, | = | L-α-phosphatidylcholine |
FTIR, | = | Fourier transform infrared spectroscopy |
LUV, | = | large unilamellar vesicles |
Tm , | = | fluid-to-gel membrane phase transition temperature |
SOPC, | = | 1-stearoyl-2-oleoyl-sn-glycero-3-phosphocholine |
Introduction
Blood transfusions are used for trauma victims, surgery, organ transplants, and patients receiving treatment for leukemia, cancer or other diseases, such as sickle cell disease and thalassemia. Due to a steadily increasing demand of blood products, there is a need to improve currently applied storage methods to avoid shortages and to ensure permanent availability for clinical use. Storage methods for blood cells include liquid storage and cryopreservation. The majority of RBC units are hypothermically stored at temperatures in the range of 2–6°C. Cell quality, however, decreases during storage which is referred to as the hypothermic storage lesion. Hypothermic storage results in translocation of phosphatidyl serine from the inner to the outer leaflet of the cell membrane and the shedding of microvesicles (Hess and Greenwalt Citation2002, Hess Citation2006). The transfusion of ‘older’ RBC units has been associated with adverse clinical outcomes and increased morbidity and mortality in critically ill patients (Tinmouth et al. Citation2006). Cryopreservation procedures for RBCs using glycerol as cryoprotective agent either need a high glycerol content (40%) combined with a low cooling rate, or a low glycerol content (17–20%) in combination with a high cooling rate is required (Smith Citation1950, Mollison and Sloviter Citation1958, Rowe et al. Citation1968, Meryman and Hornblower Citation1977). One of the main disadvantages of these procedures is the deglycerolization step, which has to be implemented prior to transfusion into patients.
Alternative and more efficient biopreservation strategies for red blood cells using non-toxic protective agents are urgently needed. Liposomes are a new promising class of protectants for hypothermic storage, cryopreservation as well al freeze-drying of RBCs (Kheirolomoom et al. Citation2005, Holovati et al. Citation2008, CitationStadnick et al. 2011 unpublished). The stabilizing effect of liposomes during cold storage and freezing has first been discovered in spermatozoa (Graham and Foote Citation1987). Recently liposomes have been implicated for preservation of RBCs during freezing (Holovati et al. Citation2008) and freeze-drying (Kheirolomoom et al. Citation2005). The stabilizing mechanisms of liposomes in cell preservation strategies, however, are still poorly understood. The protective properties may be attributed to a transfer of lipids and/or cholesterol between liposomal and RBC membranes. Cholesterol can exchange rapidly between different membrane bilayers (Bruckdorfer et al. Citation1969, Steck et al. Citation1988), whereas lipid transfer is a relatively slow process (Phillips et al. Citation1987). Saturated lipids hold promise for hypothermic storage of RBCs but provide little protection during freezing or freeze-drying, whereas various unsaturated lipids destabilize RBCs during hypothermic storage but protect during freezing and freeze-drying (Kheirolomoom et al. Citation2005, Stadnick et al. 2011). For saturated lipids the length of the acyl chains comes critical whether they stabilize or destabilize RBCs (Stoll et al. Citation2011).
We have previously shown that cholesterol transfers from RBCs to liposomes, whereas lipids in liposomes transfer to RBC membranes (Stoll et al. Citation2011). The rate of this lipid and cholesterol transfer is dependent on the type of lipid. Liposomes composed of lipids with short acyl chain lengths (i.e., DLPC) display rapid lipid and cholesterol transfer, whereas lipids with longer acyl chain length such as DPPC display relatively slow transfer. The depletion of cholesterol by liposomes can be inhibited or even reversed by adding cholesterol to the liposomes. Liposomes composed of lipids with short acyl chain lengths increase lipid disorder of RBC membranes at suprazero temperatures, whereas long acyl chain length lipids have little effects. Cholesterol has a temperature-dependent effect on the membrane phase state: It increases membrane conformational disorder at low temperatures when membranes are in the gel state and reduces the disorder at high temperatures when membranes are in the fluid state (Gousset et al. Citation2002, Wolkers et al. Citation2002).
Fourier transform infrared spectroscopy (FTIR) can be used to study the effect of liposomes on RBCs and vice versa the effect of RBCs on liposomes based on changes in thermotropic membrane phase behaviour of the liposomes and RBCs after incubation (Stoll et al. Citation2011). With this technique, changes in membrane conformational disorder (fluidity) during heating or cooling can be studied by following the peak position of the symmetric CH2 stretching vibration absorption band that arises from the lipid acyl chains as a function of temperature. FTIR has proven to be a powerful method for studying physical properties of membranes both in model systems from isolated lipids (Popova and Hincha Citation2007, Fidorra et al. Citation2009, Mannock et al. 2010b), as well as in situ in whole cells (Crowe et al. Citation1989, Citation1999, Moore et al. Citation1996, Wolkers et al. Citation2007).
This work follows up on previous studies where we investigated how liposomes composed of linear saturated acyl chains affect RBC membrane properties (Stoll et al. Citation2011) and affect RBC stability during hypothermic storage (Stadnick et al. 2011). In this study the effects of liposomes composed of unsaturated lipids on RBC membranes have been investigated. The effect of unsaturated lipids with differences in number of unsaturated bonds (18:0/18:1, 18:1/18:1, 18:2/18:2) on RBC membrane fluidity has been studied by FTIR. RBCs were incubated in the presence of liposomes and both the liposomal and the RBC fraction were analyzed by FTIR after incubation. The temperature dependence of the liposomal effects on RBC membrane properties have been investigated to reveal the activation energy of RBC liposome interactions.
Materials and methods
Preparation of liposomes
Preparation of liposomes was performed as previously described in detail (Holovati et al. Citation2008, Stoll et al. Citation2011). Lipids were obtained from Avanti Polar Lipids (Alabaster, AL). Large unilamellar vesicles (LUVs) were prepared from L-α-phosphatidylcholine (Egg-PC), 1,2-dioleoyl-sn-glycero-3-phosphocholine (DOPC, 18:1), 1-stearoyl-2-oleoyl-sn-glycero-3-phosphocholine (18:0–18:1-PC) and 1,2-dilinoleoyl-sn-glycero-3-phosphocholine (18:2-PC). The lipids were dissolved in chloroform, and the chloroform was evaporated overnight and subsequently lyophilized (Christ® Epsilon 2-10 D) to create a thin lipid film. Dry lipids were hydrated in HBSI buffer. Unilamellar vesicles were obtained by extrusion using an Avestin mini extruder with 200 nm pore size filters. The lipid concentration after extrusion was 25 mM.
Pretreatment of blood and incubation of blood in the presence of liposomes
Human blood was obtained from the Institute for Transfusion medicine (Hannover Medical School). Venous blood was collected from healthy adults, with informed consent, according to institutional protocols. Blood was anticoagulated with citrate phosphate dextrose adenine (CPDA) and centrifuged to remove buffy coat and plasma. Anticoagulated RBCs were stored in 15 ml polypropylene Falcon tubes at 1–6°C and exhausted within a week.
For incubation with liposomes the hematocrit of the RBC concentrate was adjusted to 5% by adding HBSI buffer. The liposome concentration was 6.4 mM. RBCs were incubated for 4 h at 37°C. Two experiments with different incubation parameters were performed. In both of them the incubation time was extended to 8 h. In addition, the incubation temperature was varied at 22°C, 30°C, 34°C, 37°C and 40°C. The suspension was gently rotated during incubation.
After incubation RBCs and liposomes were separated using a Ficoll density gradient separation procedure. RBCs and supernatant (liposomes) were collected and stored at 4°C. Control samples were processed under the same conditions, but with HBSI-buffer instead of liposomes.
Red blood cells contain haemoglobin, which strongly absorbs light in the IR-range. In intact RBCs, the lipid bands are relatively weak because their signal is disturbed by the strong absorbance of the haemoglobin. Haemoglobin removal has advantages for thermal analyses of the lipid band (Stoll et al. Citation2011). After haemoglobin removal, the relative contribution of the lipid signal is stronger. Therefore, FTIR analysis studies were done on RBC ghosts instead of intact cells. Erythrocyte ghosts were prepared according to Dodge et al. (Citation1963). The erythrocyte concentrate was incubated in a 100-fold amount of lysis buffer in 50 ml falcon tubes under constant stirring overnight on ice. The RBC ghosts were concentrated by centrifugation (9,112 g, 4°C, 1 h) and the supernatant was subsequently removed. This procedure was repeated in a 1.5 ml Eppendorf tube (30 min, 14,000 g), to form a more resistant pellet, which was used for FTIR-spectroscopic analysis.
FTIR spectroscopy and data processing
Infrared spectra were recorded on a Perkin-Elmer (Spectrum 100) Fourier transform IR-spectrometer as previously described (Oldenhof et al. Citation2010). Approximately 10 μl sample (RBC pellet or liposomes) was sandwiched between two CaF2 infrared windows in a temperature-controlled cell. IR spectra were recorded at 30-sec intervals during warming of the sample at 2 K/min. Data processing consisted of taking the second derivative of the IR-absorbance spectra using a nine-point smoothing factor. Inverted second derivative spectra were normalized on the lipid band around 2850 cm-1. Band positions were determined as previously described (Wolkers and Hoekstra Citation1995, Wolkers et al. Citation2002). The wavenumber (cm-1) of the CH2 symmetric stretching vibration was plotted as a function of temperature. The first derivative of the wavenumber versus temperature plots was obtained using Peakfit (Jandel Scientific, San Rafael, CA, USA) to show inflections more clearly and to obtain a measure for the co-operativity of membrane phase transitions. Phase transition temperatures and co-operativity values were determined from the maxima in the first derivatives of the wavenumber vs. temperature plots. Ghosts prepared from liposome-treated RBCs were analyzed as described above. In addition, the different liposomes were analyzed before and after incubation with RBCs. Different sample groups were measured at least three times, and a control sample that was not treated with liposomes was analyzed for every donated RBC unit.
Results
In this study the effects of various unsaturated lipids on RBC membrane properties have been investigated. Both the effects of liposome treatment on RBC membrane phase behaviour and the effects of RBCs on phase behaviour of the liposomes have been studied by FTIR-spectroscopy.
Effect of egg-PC liposomes on membrane phase behaviour of RBCs
depicts the membrane phase behaviour of RBC ghosts prepared from RBCs that have been incubated with liposomes consisting of egg-PC liposomes. Egg-PC consists of a natural mixture of various saturated and unsaturated lipids derived from egg yolk. It mainly consists of 16:0-PC, 18:0-PC, 18:1-PC and 18:2-PC. Liposome treated RBCs (filled squares) are compared with untreated control RBCs (open circles). The wavenumber of the lipid band monotonously increases with increasing temperature, indicating that RBCs do not display cooperative phase transitions. Liposome treatment causes an increase in membrane fluidity, which is particularly visible at higher temperatures. shows the effect of RBC treatment on the membrane phase behaviour of the liposomes. Egg-PC liposomes display a gel-to-liquid crystalline phase transition at −10°C. After incubation with RBCs, the tm of the liposomes increased with approximately 4°C. The increased membrane fluidity of the RBCs and the increase in tm of the liposomes likely indicate cholesterol transfer from RBCs to liposomes (Stoll et al. Citation2011).
Figure 1. Wavenumber vs. temperature plot of RBC ghosts after incubation with egg-PC liposomes. Egg-PC treated RBCs (filled squares) have a higher cooperativity than non liposome treated RBCs (open circles).
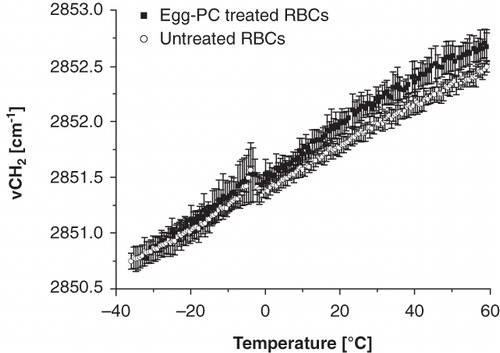
Figure 2. Wavenumber vs. temperature plot of egg-PC liposomes before (open circles) and after (filled squares) incubation with RBCs. The phase transition is visible as an abrupt increase in wavenumber and indicates a transition from gel-to-fluid phase. The tm is shifted 4.2°C after incubation with RBCs.
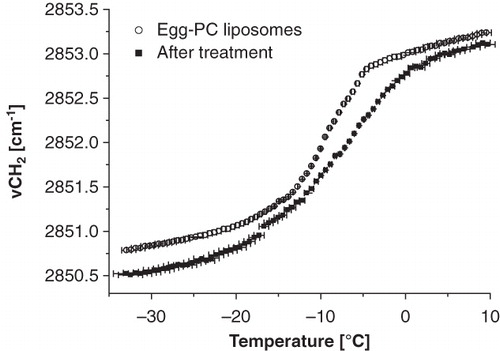
Effect of unsaturated liposomes on membrane phase behaviour of RBCs
shows the wavenumber versus temperature plots of RBCs after treatment with liposomes consisting of different unsaturated PCs. The acyl chain length of the lipids is the same, but the lipids vary in saturation level (one, two or four unsaturated bonds). Panel A and D depict the RBC and liposomal fraction of RBCs incubated with 18:0/18:1-PC (SOPC) liposomes, panel B and E depict 18:1/18:1-PC (DOPC)-treated RBCs, and panel C and F depicts 18:2/18:2-PC (DLPC)-treated RBCs. The shape of the wavenumber versus temperature plots of the RBCs after liposome treatment is similar among the different lipids (, panel ) and similar to that of the RBCs after incubation with egg-PC liposomes. The wavenumber vs. temperature plots of liposomes before (open circles) and after (filled squares) incubation with RBCs (, panel ) show that the tm of the liposomes increased after incubation. The tm of the lipids is visible as an abrupt increase in vCH2 associated with the gel-to liquid-crystalline-phase transition. In liposomes consisting of lipids with one unsaturated bond (18:0–18:1-PC) the shift in tm after incubation is approximately 2°C. With increasing number of double bonds in the acyl chains, the shift in tm after incubation increases. The tm of DOPC increases by 7°C after incubation. The strongest shift was found in 18:2-PC, which contains four unsaturated bonds (Δtm = 9°C) (). shows a correlation plot of the shift in tm after incubation with RBCs, Δtm, versus the original tm of the lipids. The saturated DSPC, 18:0, which is also included in the plot, shows a decrease in tm after incubation.
Figure 3. Membrane phase behaviour of RBCs (panel A–C) and liposomes from unsaturated lipids (panel D–F). The data points of panel A–C reflect νCH2 vs. temperature plots of non-liposome-treated RBCs (open circles) and liposome-treated RBCs (filled squares). Panel D–F show wavenumber vs. temperature plots of the liposomes before (open circles) and after incubation (filled circles) with red blood cells.
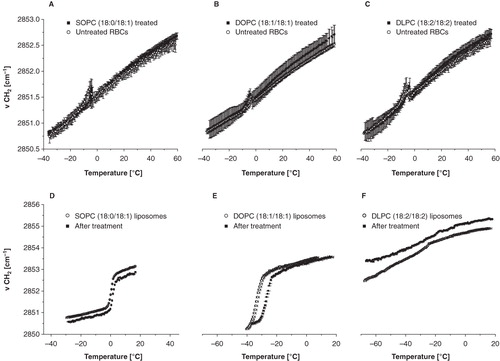
Incubation time and temperature
The effect of liposomes on RBC membrane phase behaviour is dependent on incubation time and temperature. In order to quantify the effects of liposomes on RBC membrane phase behaviour, the incubation time and incubation temperature were varied. The slope in the wavenumber versus temperature plots of the RBCs, which was determined via linear fitting from 0–50°C, increased over time. shows that the slope increases in a linear fashion as a function of the incubation time in the presence of liposomes, whereas the slope does not change in the control cells. This effect is likely due to cholesterol or lipid transfer between RBCs and liposomes. The increase in slope in the presence of liposomes is also dependent on the incubation temperature and displays Arrhenius behaviour. shows an Arrhenius plot of the increase in slope (taken as D) after 8-h incubation with liposomes. The process has an activation energy of 87.5 kJ mol-1, and likely reflects cholesterol transfer from RBCs to liposomes.
Discussion
We have previously shown that liposomes composed of saturated lipids can be used to modify RBC membranes. The length of the lipid acyl chains determines the rate of cholesterol exchange and lipid transfer between RBCs and liposomes (Stoll et al. Citation2011), which in turn affects RBC stability (Stadnick et al. 2011). In this study the effects of liposomes made from unsaturated lipids on RBC membrane properties have been investigated. Membrane phase behaviour of both liposomes and RBCs is altered after incubation, indicating cholesterol and/or lipid transfer. The ultimate aim of these studies is to develop liposome formulations for biopreservation strategies of mammalian cells. Liposomes composed of unsaturated lipids have been used as stabilizing agents for freeze drying of red blood cells (Kheirolomoom et al. Citation2005) and cryopreservation of spermatozoa (Graham and Foote Citation1987).
Liposomes cause several alterations in the cell membranes structure, which range from an increased conformational disorder (Levine and Wilkins Citation1971, Moore et al. Citation2005) at low temperatures, an increased bilayer thickness (McIntosh Citation1978) and an increased permeability for hydrophilic solutes (Kimbelberg, Citation1978) affecting the stability of RBCs at reduced temperatures. In our previous study we have investigated how saturated lipids affect red blood cell membranes and found that liposomes deplete cholesterol from RBC membranes (Stoll et al. Citation2011). This was particularly observed with short acyl chain length lipids, such as DLPC, which cause a strong reduction in cholesterol/PL ratio coinciding with strong haemolysis. In addition, DLPC incorporates in RBC membranes. DMPC also shows cholesterol exchange and lipid transfer with RBCs. Lipids with larger acyl chain length, such as DPPC or DSPC, however, display much slower cholesterol and lipid exchange. The unsaturated lipids that were studied here all have a fluidizing effect on RBC membranes irrespective of the saturation level. This is most likely due to transfer of cholesterol from RBCs to liposomes. MALDI-TOF Mass Spectrometry measurements did not show evidence lipid incorporation in RBC membranes (data not shown). Not only RBC membranes are affected after incubation, also the membrane phase behaviour of the liposomes is changed after incubation. This is evident from a shift in the main gel-to-fluid phase transition temperature. The higher the number of unsaturated bonds of the liposomal lipids, the greater the shift in the phase transition temperature. This effect can be explained by incorporation of cholesterol in the liposomes. McMullen et al. (Citation1993) studied the effect of cholesterol in a homologous series of saturated phosphatidylcholine model membranes. At chain lengths <17 CH2 groups the tm shifts to higher temperatures, whereas above 17 CH2 groups, tm shifts to lower temperatures. A cholesterol concentration of 15 mol% shifts the tm of 1-stearoyl-2-oleoylphosphatidylcholine by 3°C (Vilchèze et al. Citation1996). The temperature shift was attributed to the hydrophobic mismatch between the effective lengths of the cholesterol molecule compared to that of the PC hydrocarbon chains normal to the bilayer plane. The higher the mismatch between the phospholipid and the cholesterol, the more profound is the effect on the phase transition. The presence of unsaturation determines the effective length of the PC bilayer, the crankshaft-kink conformation decreases the hydrophobic length only slightly compared to saturated analogues and appears to permit more favorable sterol/PC contacts in the gel state than a cis-kink (Vilchèze et al. Citation1996 Mannock et al. 2010a, Mouritsen and Bloom Citation1984). This indicates that the shift in tm of the liposomal membrane is not proportional to the amount of incorporated cholesterol, but is determined by the number of unsaturated bonds of the lipid acyl chains.
The transfer of cholesterol from membranes likely occurs through an activation-collision mechanism (Phillips et al. Citation1987). The rate of transfer is dependent on the number of donor and acceptor molecules and the total amount of fluid respectively the hematocrit during incubation. Time and temperature were mentioned as important parameters for the cholesterol transfer rate. The transfer of lipids is a relatively slow process, whereas cholesterol transfer is relatively fast reaching equilibrium in the order of hours (Steck et al. Citation1988). The rate of cholesterol transfer between cells and liposomes can be decreased by incorporating cholesterol in the liposomes. Phillips et al. (Citation1987) carried out comparable studies on model membranes consisting of different lipids, and showed that cholesterol transfer can be described by first order kinetics and displays Arrhenius behaviour. These studies showed that the activation energy for cholesterol exchange is close to 88 kJ/mol, which is similar to the activation energy we report here. This further supports the idea that the observed effects of liposomes on RBC membranes are due to transfer of cholesterol. We suggest that the temperature dependency may arise from structural alterations within the RBC membrane with increasing temperature. Reinl et al. (Citation1992) postulated a movement of cholesterol molecules in DPPC/cholesterol vesicles in the liquid ordered phase from the centre towards the polar head group of the region of the membrane. As cholesterol is placed near to the surface of the RBC membrane, it could easily transfer to liposomes through collision.
Conclusion
Liposomes composed of unsaturated lipids increase RBC membrane fluidity. The level of unsaturation of the lipids in liposomes, however, does not affect the fluidizing effect on RBC membranes. Liposomes composed of unsaturated lipids, such as the ones that were studied here, have been shown to increase cryostability of RBCs and spermatozoa (Graham and Foote Citation1987, Kheirolomoom et al. Citation2005). We suggest that the improved cryosurvival of RBCs in the presence of liposomes is related to the fluidizing effect of liposomes on RBC membranes.
Acknowledgements
We kindly acknowledge Dr Heuft from the Hanover Medical school blood donation service for providing blood samples for the experiments. This work is supported by funding from the Deutsche Forschungsgemeinschaft (DFG, German Research Foundation) for the Cluster of Excellence REBIRTH (From Regenerative Biology to Reconstructive Therapy) and from a grant “Liposomes in Transfusion Medicine: An Approach for Reducing the Red Blood Cell Hypothermic Storage Lesion” to JA from the Canadian Blood Services/Canadian Institutes for Health Research.
Declaration of interest: The authors report no conflicts of interest. The authors alone are responsible for the content and writing of the paper.
References
- Bruckdorfer KR, Demel RA, de Gier J, van Deenen LLM. 1969. The effect of partial replacements of membrane cholesterol by other steroids on the osmotic fragility and glycerol permeability of other erythrocytes. Biochim Biophys Acta 183:334–345.
- Crowe JH, Hoekstra FA, Crowe LM, Anchordoguy TJ, Drobnis E. 1989. Lipid phase transitions measures in intact cells with Fourier transform infrared spectroscopy. Cryobiology 26:76–84.
- Crowe JH, Tablin F, Tsvetkova N, Oliver AE, Walker NJ, Crowe LM. 1999. Are lipid phase transitions responsible for chilling damage in human platelets? Cryobiology 38:180–191.
- Dodge JT, Mitchell GD, Hanahan DJ. 1963. The preparation and chemical characteristics of haemoglobin-free ghosts of human erythrocytes. Archs Biochem Biophys 100:119–130.
- Fidorra M, Heimburg T, Seeger HM. 2009. Melting of individual lipid components in binary lipid mixtures studied by FTIR spectroscopy, DSC and Monte Carlo simulations. Biochim Biophys Acta 1788:600–607.
- Gousset K, Wolkers WF, Tsvetkova NM, Oliver AE, Field CL, Walker NJ, Crowe JH, Tablin F. 2002. Evidence for a physiological role for membrane rafts in human platelets. J Cell Physiol 119:117–128.
- Graham JK, Foote RH. 1987. Effect of several lipids, fatty acyl chain length, and degree of unsaturation on the motility of bull spermatozoa after cold shock and freezing. Cryobiology 24:42–52.
- Hess JR. 2006. An update on solutions for red cell storage. Vox Sanguinis 91:13–19.
- Hess JR, Greenwalt TG. 2002. Storage of red blood cells: New approaches. Transfus Med Rev 16(4):283–295.
- Holovati JL, Gyongyossy-Issa M, Acker JP. 2008. Effects of trehalose-loaded liposomes on red blood cell response to freezing and post-thaw membrane quality. Cryobiology 58:75–83.
- Kimbelberg HK. 1978. Influence of lipid phase transitions and cholesterol on protein-lipid interactions. Cryobiology 15:222–226.
- Kheirolomoom A, Satpathy GR, Torok Z, Banerjee M, Bali R, Novaes RC, Little E, Manning DM, Dwyre DM, Tablin F, Crowe JH, Tsvetkova NM. 2005. Phospholipid vesicles increase the survival of freeze-dried human red blood cells. Cryobiology 51:290–305.
- Levine YK, Wilkins MHF. 1971. Structure of oriented lipid bilayers. Nature New Biol 230:69–72.
- Mannock DA, Lewis RNAH, McElhaney RN. 2010a. A calorimetric and spectroscopic comparison of the effects of ergosterol and cholesterol on the thermotropic phase behavior and organization of dipalmitoylphosphatidylcholine bilayer membranes. Biochim Biophys Acta 1798:376–388.
- Mannock DA, Lewis RNAH, McMullen TPW, McElhaney RN. 2010b. The effect of variations in phospholipid and sterol structure on the nature of lipid-sterol interactions in lipid bilayer model membranes. Chem Phys Lipids 163:403–448.
- McIntosh TJ. 1978. The effect of cholesterol on the structure of phosphatidylcholine bilayers. Biochim Biophys Acta 513:43–58.
- McMullen T, Lewis RN, McElhaney R. 1993. Differential scanning calorimetric study of cholesterol on the thermotropic phase behavior of a homologous series of linear saturated phosphatidylcholines. Biochemistry – US 31:516–522.
- Meryman HT, Hornblower M. 1977. Simplified procedure for deglycerolizing red blood-cells frozen in a high glycerol concentration. Transfusion 17(5):438–442.
- Mollison PL, Sloviter HA. 1958. Successful transfusion of previously frozen red blood cells. Lancet (ii):862–864.
- Moore DJ, Sills RH, Patel N, Mendelsohn R. 1996. Conformational order of phospholipids incorporated into human erythrocytes: An FTIR spectroscopy study. Biochemistry – US 35:229–235.
- Moore AI, Squires EL, Graham JK. 2005. Adding cholesterol to the stallion sperm plasma membrane improves cryosurvival. Cryobiology 51(3):241–249.
- Mouritsen OG, Bloom M. 1984. Mattress Model of lipid-protein interactions in membranes. Biophys J 46:141–151.
- Oldenhof H, Friedel K, Sieme H, Glasmacher B, Wolkers WF. 2010. Membrane permeability parameters for freezing of stallion sperm as determined by Fourier transform infrared spectroscopy. Cryobiology 61:115–122.
- Phillips MC, Johnson WJ, Rothblat GH. 1987. Mechanisms and consequences of cellular cholesterol exchange and transfer. Biochim Biophys Acta 906:223–276.
- Popova M, Hincha DK. 2007. Effects of cholesterol on dry bilayers: Interactions between phosphatidylcholine unsaturation and glycolipid or free sugar. Biophys J 93:1204–1214.
- Reinl H, Brumm T, Bayerl TM. 1992. Changes of the physical properties of the liquid-ordered phase with temperature in binary mixtures of DPPC with cholesterol – A 2H-NMR, FT-IR, DSC, and neutron scattering study. Biophys J 61:1025–1035.
- Rowe AW, Eyster E, Kellner A. 1968. Liquid nitrogen preservation of red blood cells for transfusion: A low glycerol-Rapid freeze procedure. Cryobiology 5:119–128.
- Smith AU. 1950. Prevention of haemolysis during freezing and thawing of red blood-cells. Lancet 265:910–911.
- Stadnick H, Stoll C, Wolkers WF, Acker JP, Holovati JL. 2011. Application of liposomes to improve the hypothermic storage of red blood cells. Vox Sang 99(suppl, 1):67–68.
- Steck TL, Kezdy FJ, Lange Y. 1988. An activation-collision mechanism for cholesterol transfer between membranes. J Biol Chem 263(26):13023–13031.
- Stoll C, Stadnick H, Kollas O, Holovati JL, Glasmacher B, Acker JP, Wolkers WF. 2011. Liposomes alter thermal phase behavior and composition of red blood cell membranes. BBA – Biomembranes 1808:474–481.
- Tinmouth A, Fergusson D, Yee IC. 2006. Clinical consequences of red cell storage in the critically ill. Transfusion 46(11):2014–2027.
- Vilchèze C, McMullen TPW, McElhaney RN, Bittman R. 1996. The effect of side-chain analogues of cholesterol on the thermotropic phase behavior of 1-stearoyl-2-oleoylphosphatidylcholine bilayers: A differential scanning calorimetric study. Biochim Biophys Acta 1279:235–242.
- Wolkers WF, Balasubramanian SK, Ongstad EL, Bischof JC. 2007. Effects of freezing on membranes and proteins in LNCaP prostate tumor cells. BBA – Biomembranes 1768:728–736.
- Wolkers WF, Crowe LM, Tsvetkova NM, Tablin F, Crowe JH. 2002. In situ assessment of erythrocyte membrane properties during cold storage. Mol Mem Biol 19:59–65.
- Wolkers WF, Hoekstra FA. 1995. Aging of dry desiccation-tolerant pollen does not affect protein secondary structure. Plant Physiol 109:907–915.