Abstract
β-catenin, a multifunctional protein expressed in all tissues including the heart stimulates the expression of several genes important for cell proliferation. Signaling involving ß-catenin participates in directing cardiac development and in the pathophysiology of cardiac hypertrophy. Nothing is known, however, on the role of β-catenin in the regulation of cardiac ion channels. The present study explored the functional interaction of β-catenin and KCNE1/KCNQ1, the K+ channel complex underlying the slowly activating outwardly rectifying K+ current. To this end, KCNE1/KCNQ1 was expressed in Xenopus oocytes with and without β-catenin and the depolarization (up to + 80 mV) induced current (IKs) was determined using the two-electrode voltage clamp. As a result, β-catenin enhanced IKs by 30%. The effect of β-catenin on IKs was not affected by actinomycin D (10 μM), an inhibitor of transcription, indicating that β-catenin was not effective as transcription factor. Confocal microscopy revealed that β-catenin enhanced the KCNE1/KCNQ1 protein abundance in the cell membrane. Exposure of the oocytes to brefeldin A (5 μM), an inhibitor of vesicle insertion, was followed by a decline of IKs, which was then similar in oocytes expressing KCNE1/KCNQ1 together with β-catenin and in oocytes expressing KCNE1/KCNQ1 alone. In conclusion, β-catenin enhances IKs by increasing the KCNE1/KCNQ1 protein abundance in the cell membrane, an effect requiring vesicle insertion into the cell membrane.
Introduction
The multifunctional protein β-catenin is expressed in most tissues including cardiac tissue (Blankesteijn et al. Citation2008). It may enter the nucleus and stimulate the expression of genes important for cell proliferation (He et al. Citation1998, Tetsu and McCormick Citation1999). ß-catenin may further interact with the actin cytoskeleton (Pokutta et al. Citation2008). Phosphorylation of β-catenin by glycogen synthase kinase GSK3 triggers the proteasomal degradation of ß-catenin (Zumbrunn et al. Citation2001, van Noort et al. Citation2002, Cadigan and Liu Citation2006). GSK3-dependent phosphorylation is mediated by binding of both GSK3ß and β-catenin to the adenomatous polyposis coli (APC) protein (Korinek et al. Citation1997, Morin et al. Citation1997, Polakis Citation2000, Giles et al. Citation2003). Impaired degradation of β-catenin by defective APC fosters cell proliferation leading to the development of multiple intestinal tumors in humans (Takayama et al. Citation2006). Similarly, intestinal tumor formation is observed in mice carrying a truncating mutation in the apc gene (Moser et al. Citation1990).
In the heart, ß-catenin is critically important for organ development (Tzahor Citation2007, Grigoryan et al. Citation2008). Moreover, enhanced β-catenin activity participates in the pathophysiology of cardiac hypertrophy (Blankesteijn et al. Citation2008, Sugden et al. Citation2008). As a transcription factor, β-catenin stimulates the expression of the serum- and glucocorticoid-inducible kinase SGK1 (Naishiro et al. Citation2005, Dehner et al. Citation2008). In line with this, SGK1 expression is enhanced in apcMin/+ mice (Rotte et al. Citation2008). SGK1 phosphorylates and thus inactivates GSK3 (Sakoda et al. Citation2003), which should thus counteract β-catenin degradation. Moreover, SGK1 stimulates several channels including KCNQ1/KCNE1 (Lang et al. Citation2006) as well as the Na+/K+ ATPase (Setiawan et al. Citation2002, Verrey et al. Citation2003, Zecevic et al. Citation2004). Along those lines, a gain of function variant of the SGK1 gene is associated with a shortened QT interval (Busjahn et al. Citation2004, Seebohm et al. Citation2008).
The present study explored the role of β-catenin in the regulation of KCNE1/KCNQ1. To this end, KCNE1/KCNQ1 was expressed in Xenopus oocytes with or without β-catenin and channel activity determined by two-electrode voltage clamp.
Materials and methods
Voltage clamp experiments
For the generation of cRNA, constructs were used encoding wild type β-catenin (Sopjani et al. Citation2010a) and KCNE1/KCNQ1 (Heitzmann et al. Citation2007, Seebohm et al. Citation2008, Henrion et al. Citation2009). The cRNA was generated as described previously (Bohmer et al. Citation2010, Eckey et al. Citation2010). For voltage clamp analysis, Xenopus oocytes were prepared as previously described (Bohmer et al. Citation2010, Eckey et al. Citation2010). Where indicated 7.5 ng of β-catenin cRNA were injected with or without 1.5 ng KCNE1 and 1.5–5 ng KCNQ1 cRNA on the first day after preparation of the Xenopus oocytes. All experiments were performed at room temperature 3 days after cRNA injection. In two-electrode voltage-clamp experiments KCNQ1/KCNE1 channel currents were elicited every 20 s with 5 s depolarizing pulses from −120 mV to + 80 mV. Pulses were applied in 20 mV increments. The data were filtered at 1 kHz and recorded with a GeneClamp 500 amplifier, a DigiData 1322A A/D-D/A converter and the pClamp 9.0 software package for data acquisition and analysis (Axon Instruments, USA). The control bath solution (superfusate/ND96) contained 96 mM NaCl, 2 mM KCl, 1.8 mM CaCl2, 1 mM MgCl2 and 5 mM HEPES, pH 7.4. The final solutions were titrated to pH 7.4 using NaOH. The flow rate of the superfusion was 20 ml/min and a complete exchange of the bath solution was reached within about 10 s.
Immunhistochemistry
Approximately 5 oocytes were fixed in 4% paraformaldehyde/PBS for 24 h. Then, the oocytes were cryoprotected with 30% sucrose in PBS for another day and then frozen in mounting medium (Tissue-Tek, O.C.T Compound Containing – SAKURA). Cryostat Sections were made at a thickness of 6–8 μm and stored at −20°C. For immunostainings, sections were dehydrated for 30 min at room temperature, fixed for 15 min in acetone/methanol (1:1), washed 3 times for 5 min in PBS and incubated in 5% bovine-serum-albumin for 1 h. The sections were washed again in PBS and incubated with the first antibody (rabbit anti-KCNQ1 1:500 antibody; Abcam, Cambridge, UK) in a moist chamber overnight at 4°C. Binding of the first antibody was visualized with a fluorescent dye-labeled 633 Alexa Fluor antibody (Invitrogen, Karlsruhe, Germany). The staining was then analyzed on a confocal laser-scanning microscope (Zeiss LSM 510 Exciter) using an Ar laser at 633 nm excitation wavelength and appropriate filter set.
Chemiluminescence
Oocytes were injected with H20 or with cRNA encoding FLAG-tagged KCNQ1 (the FLAG tag was inserted at the N-terminal end (Kanki et al. Citation2004)) with or without additional injection of β-catenin cRNA. After incubation, the oocytes were shaken on ice with 1% bovine-serum-albumin in ND96 medium for 30 min to block non-specific antibody binding. Oocytes were subsequently incubated with rabbit anti-FLAG antibody (Sigma, Schnelldorf, Germany; 1:200) for 60 min on ice, washed 3 times for 5 min with 1% bovine-serum-albumin in ND96 and incubated on ice with secondary anti-rabbit IgG antibody (Invitrogen, Karlsruhe, Germany; 1:1000) for 60 min. The oocytes were then washed 3 times for 20 min with 1% bovine-serum-albumin in ND96 and additional 3 times for 5 min with ND96 medium. Individual oocytes were transferred into the wells of a 96-well plate with 100 μl ND96 per well. Twenty μl of SuperSignal ELISA Femto Maximum Sensitivity Substrate (Pierce, Schwerte, Germany) was added to each well and chemiluminescence was quantified by a multilabel counter (Wallac Victor, Perkin Elmer, Rodgau, Germany).
Site-directed mutagenesis
The human truncated β-catenin (β-catenin 1-530) (Fagotto et al. Citation1996) was generated by replacing Gly531 with a STOP codon by site-directed mutagenesis using the Quick Change II XL Site-Directed Mutagenesis Kit (Stratagene, Heidelberg, Germany) according to the manufacturer's instructions. The following primers were used:
sense: 5′-ACCTTTGCGTGAGCAGTGAGCCATTCCACGACTAG-3′ and
antisense: 5′-CTAGTCGTGGAATGGCTCACTGCTCACGCAAAGGT-3′.
The mutant was sequenced to verify the desired mutation.
Statistical analysis
Data are provided as means ± SEM; n represents the number of oocytes studied. All data were tested for significance using ANOVA and only results with p < 0.05 were considered statistically significant. Since the magnitude of the voltage-gated current varies markedly among different oocyte batches, the current was normalized for statistical analysis as detailed in the Figure legends.
Results
The heteromeric KCNE1/KCNQ1 channel mediates an outwardly-directed K+ current activated by depolarization of the cell membrane. In KCNE1/KCNQ1-expressing Xenopus oocytes, but not in water injected oocytes, depolarization of the cell membrane from −120 mV to + 80 mV in 20 mV steps led to the typical delayed outward current IKs (). The coexpression of β-catenin was followed by a significant increase in the outward current. After substracting the voltage-gated current at + 80 mV determined in water-injected oocytes the delayed outward current at + 80 mV was 1637 ± 367 nA (n = 8 oocyte batches) in oocytes expressing only KCNE1/KCNQ1 and 2186 ± 490 nA (n = 8 oocyte batches) in oocytes expressing both KCNE1/KCNQ1 and ß-catenin, values significantly different (p < 0.05). To further verify a specific effect of β-catenin on KCNE1/KCNQ1, the delayed outward current was determined with or without the KCNQ1 inhibitor chromanol 293b. As a result, coexpression of β-catenin significantly increased the voltage-gated current at + 80 mV in KCNE1/KCNQ1-expressing oocytes in the absence of chromanol 293 b from 1.00 ± 0.05 rel. units (n = 14 oocytes) to 1.34 ± 0.04 rel. units (n = 14). In the presence of 100 μM chromanol in the bath, however, the determined current at + 80 mV was not significantly different between oocytes expressing ß-catenin (0.50 ± 0.09; n = 14) and those not expressing ß-catenin (0.39 ± 0.07; n = 14). Thus, β-catenin expression leads to an upregulation of KCNE1/KCNQ1-mediated current. In order to study, whether association of ß-catenin with cadherin is required for the ß-catenin effect on KCNE1/KCNQ1, a mutant form of ß-catenin (ß-catenin 1-530; (Fagotto et al. Citation1996)) with low binding affinity for cadherin was generated and expressed in Xenopus oocytes. As shown in , ß-catenin 1-530 was still capable of stimulating KCNE1/KCNQ1 activity. However, its effect was significantly smaller than the effect of wild type ß-catenin.
Figure 1. Increase in KCNE1/KCNQ1 activity in Xenopus oocytes by β-catenin. (A) Original tracings demonstrating outward currents activated by depolarizations from −120 to + 80 mV in 20 mV steps in Xenopus oocytes injected with water (upper panel), in Xenopus oocytes injected with cRNA encoding KCNE1/KCNQ1 (middle panel) and in Xenopus oocytes injected with cRNA encoding KCNE1/KCNQ1 and β-catenin (lower panel). (B) Arithmetic means ± SEM (n = 7–-24) of the normalized depolarization-induced K + current at + 80 mV in Xenopus oocytes injected with water (left bar) or expressing KCNE1/KCNQ1 without (middle bar) and with (right bar) by β-catenin. ***indicates significant difference from water-injected oocytes (p < 0.001). ### indicates significant difference from the absence of β-catenin (p < 0.001). For normalization, every current at + 80 mV was divided by the average current of only KCNE1/KCNQ1-injected oocytes of the same oocyte batch. (C) Arithmetic means ± SEM of the non-normalized depolarization-induced current as a function of the potential in Xenopus oocytes injected as in B.
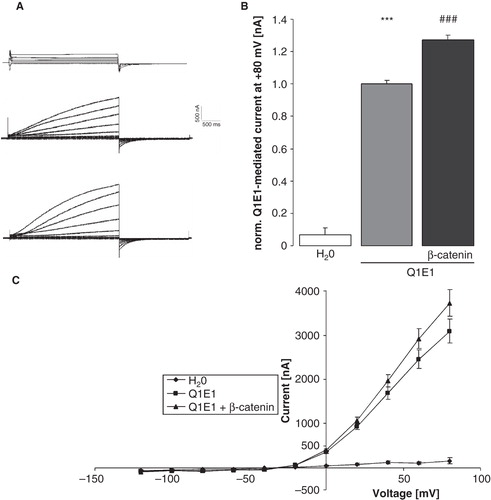
Figure 2. Stimulation of KCNE1/KCNQ1 activity in Xenopus oocytes by β-catenin depends in part on its capability to associate with cadherin. (A) Arithmetic means ± SEM (n = 12–36) of the normalized depolarization-induced K + current at + 80 mV in Xenopus oocytes injected with water (1st bar) or expressing KCNE1/KCNQ1 without (2nd bar) and with (3rd bar) wild-type β-catenin or with (4th bar) β-catenin 1-530 with decrased binding affinity for cadherin. *p < 0.05; ***p < 0.001. For normalization, every current at + 80 mV was divided by the average current of only KCNE1/KCNQ1-expressing oocytes from the same oocyte batch. (B) Arithmetic means ± SEM of the non-normalized depolarization-induced current as a function of the potential in Xenopus oocytes injected as in (A).
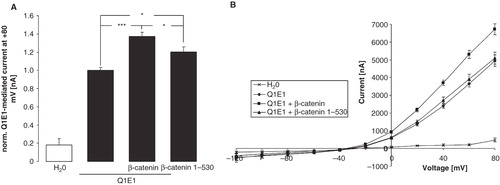
As ß-catenin is a transcription factor, its effect could have been due to stimulation of gene transcription leading to the expression of an endogeneous protein, such as SGK1, which may in turn upregulate KCNE1/KCNQ1 activity. To test that possiblility, experiments were repeated in the presence of the transcription inhibitor actinomycin D (10 μM). As illustrated in , the coexpression of β-catenin upregulated the KCNE1/KCNQ1 activity even in Xenopus oocytes treated with actinomycin D.
Figure 3. Stimulation of KCNE1/KCNQ1 activity in Xenopus oocytes by β-catenin does not depend on transcription. (A) Arithmetic means ± SEM (n = 22–50) of the normalized depolarization-induced K + current at + 60 mV in Xenopus oocytes injected with water (left bars) or expressing KCNE1/KCNQ1 without (middle bars) and with (right bars) β-catenin in the absence (white bars) and presence (black bars) of 10 μM actinomycin D. ***indicates significant difference from water-injected oocytes (p < 0.001). ### indicates significant difference from the absence of β-catenin (p < 0.001). For normalization, every current at + 60 mV was divided by the average current of only KCNE1/KCNQ1-injected oocytes of the same oocyte batch. (B) Arithmetic means ± SEM of the non-normalized depolarization-induced current as a function of the potential in Xenopus oocytes injected as in A.
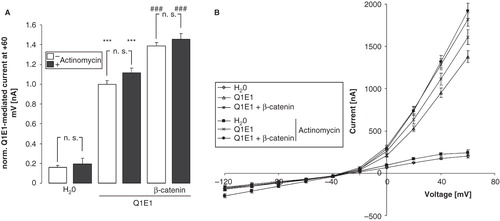
To test, whether actin polymerization is required for the ß-catenin effect on KCNE1/KCNQ1, the voltage-gated current was determined in oocytes incubated in the presence or absence of the actin polymerization inhibitor cytochalasin D for 2 h. As a result, coexpression of ß-catenin significantly increased the normalized current in KCNE1/KCNQ1-expressing oocytes from 1.00 ± 0.04 rel. units (n = 15) to 1.30 ± 0.06 rel. units (n = 15) in the absence of cytochalasin D. The addition of cytochalasin D to the culture medium did not affect the voltage-gated current in oocytes expressing KCNE1/KCNQ1 only (0.99 ± 0.04 rel. units, n = 15). However, coexpression of ß-catenin failed to stimulate the voltage-gated current in oocytes incubated with cytochalasin D for 2 h (1.02 ± 0.05 rel. units; n = 15).
In theory, ß-catenin could stimulate KCNE1/KCNQ1-mediated currents by activating the channel protein or by enhancing the KCNE1/KCNQ1 protein abundance in the cell membrane. Thus, immunofluorescence and confocal microscopy were employed to define the protein abundance of FLAG-tagged KCNQ1 in the plasma membrane. As illustrated in , KCNQ1 protein abundance in the cell membrane was indeed enhanced by coexpression of β-catenin. The results were confirmed by specific chemiluminescence-based antibody binding to surface KCNQ1 in Xenopus oocytes (). The functionality of FLAG-tagged KCNQ1 was verified with two-electrode voltage clamp. As a result, the current at + 80 mV was 2423 ± 260 nA (n = 7) in oocytes expressing KCNQ1/E1 and 2008 ± 155 nA (n = 7) in oocytes expressing FLAG-tagged KCNQ1 together with KCNE1, values not significantly different. Thus, FLAG-tagged KCNQ1 was functional.
Figure 4. β-catenin enhances the KCNQ1 protein abundance within the plasma membrane of oocytes. (A) Confocal microphotographs of KCNQ1 protein abundance at the cell surface of Xenopus oocytes injected with water (left panel), expressing KCNE1/KCNQ1 alone (middle panel) or expressing ß-catenin together with KCNE1/KCNQ1 (right panel). (B) Arithmetic means ± SEM (n = 51–53) of the normalized KCNE1/KCNQ1-dependent chemiluminescence intensity of Xenopus oocytes injected with water (left bar), expressing KCNE1/KCNQ1 alone (middle bar) or expressing ß-catenin together with KCNE1/KCNQ1 (right bar). ***indicates significant difference from water-injected oocytes (p < 0.001). #indicates significant difference from the absence of β-catenin (p < 0.05). This Figure is reproduced in color in the online version of Molecular Membrane Biology.
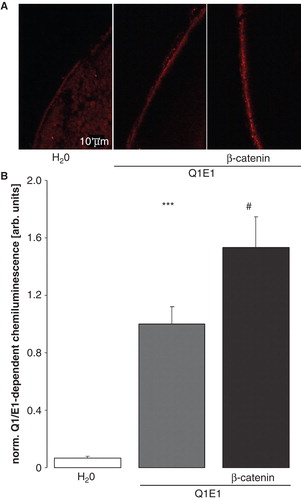
β-catenin could enhance the protein abundance in the cell membrane either by insertion of KCNE1/KCNQ1 into the cell membrane or by delaying the retrieval of the channel protein from the membrane. To discriminate between these two mechanisms, further experiments were performed with brefeldin A (5 μM), which inhibits the Golgi apparatus-dependent protein trafficking and thus the insertion of newly synthesized proteins into the cell membrane. As shown in , in the presence of brefeldin A, the decline of IKs was similar in oocytes expressing β-catenin and KCNE1/KCNQ1 and in oocytes expressing KCNE1/KCNQ1 alone. Thus, the stimulating effect of β-catenin on IKs was lost in the presence of brefeldin A.
Figure 5. β-catenin enhances the insertion of KCNE1/KCNQ1 into the membrane. (A) Arithmetic means ± SEM (n = 15–29) of the normalized depolarization-induced K + current at + 80 mV in Xenopus oocytes injected with KCNE1/KCNQ1 without (white bars) or with (black bars) additional coexpression of β-catenin and incubated in the absence of brefeldin A (left bars) or incubated in the presence of 5 μM brefeldin A for the last one (middle bars) or two (right bars) days. ###indicates significant difference from the absence of brefeldin A (p < 0.001). ***indicates significant difference from the absence of β-catenin (p < 0.001). For normalization, every current at + 80 mV was divided by the average current of only KCNE1/KCNQ1-expressing oocytes from the same oocyte batch. (B) Arithmetic means ± SEM of the non-normalized depolarization-induced current as a function of the potential in Xenopus oocytes injected as in (A).
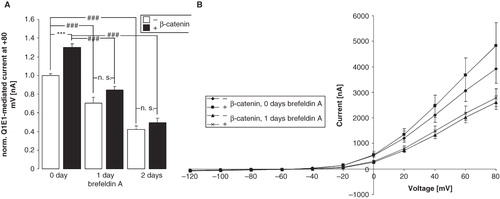
Discussion
The present observations disclose a novel function of the multifunctional protein β-catenin, i.e., the regulation of KCNE1/KCNQ1 channels. According to the present observations, β-catenin upregulates the outwardly directed current IKs in KCNE1/KCNQ1-expressing Xenopus oocytes. According to confocal microscopy, the effect of β-catenin can be explained by increased abundance of the KCNE1/KCNQ1 protein in the cell membrane. In the presence of brefeldin A the decline of IKs was not delayed by coexpression of β-catenin and the differences of IKs between Xenopus oocytes expressing both, KCNE1/KCNQ1 and β-catenin, and Xenopus oocytes expressing KCNE1/KCNQ1 alone were lost. This result suggests that β-catenin is effective by stimulating the insertion of KCNE1/KCNQ1 protein into rather than by inhibiting its retrieval from the cell membrane. It should be kept in mind, however, that prolonged incubation of the oocytes with brefeldin A might have affected trafficking and function of further proteins thus interacting with additional cellular mechanisms.
Since β-catenin 1-530 stimulated KCNE1/KCNQ1 to a lesser extent than wild type β-catenin, binding of β-catenin to the cell adhesion molecule cadherin may be needed for a full upregulation of KCNE1/KCNQ1 by β-catenin. The effect of ß-catenin on KCNE1/KCNQ1 was disrupted by the actin polymerization inhibitor cytochalasin D, indicating involvement of actin filaments. As a matter of fact, catenins are known to interact with the actin cytoskeleton (Pokutta et al. Citation2008).
The mechanisms underlying ß-catenin-sensitive KCNE1/KCNQ1 protein abundance remained, however, ill-defined. The ß-catenin protein generated by translation from the injected mRNA could, in theory, enter the nucleus of the oocytes and modulate expression of endogenous genes as transcription factor. For instance, ß-catenin could stimulate IKs by upregulating the expression of SGK1 (Naishiro et al. Citation2005, Dehner et al. Citation2008) and subsequent stimulation of KCNE1/KCNQ1 by SGK1 (Busjahn et al. Citation2004, Seebohm et al. Citation2008, Strutz-Seebohm et al. Citation2009). Xenopus oocytes do express an endogenous SGK1 (Sopjani et al. Citation2010b). However, β-catenin coexpression is effective in the presence of actinomycin. Thus, at least in our experiments, enhanced endogenous expression of factors such as SGK, hardly accounts for the stimulating effect of ß-catenin on KCNE1/KCNQ1. Recently, β-catenin has been shown to similarly upregulate the Na+/K+ ATPase independently of transcripition (Sopjani et al. Citation2010a).
While in our experiments ß-catenin is not effective through upregulation of SGK1, the present observations reveal the possibility that the known stimulating effect of SGK1 on KCNE1/KCNQ1 (Lang et al. Citation2006) could result partially from regulation of β-catenin abundance. β-catenin degradation is fostered by glycogen synthase kinase (GSK3) (Cadigan and Liu Citation2006), which is in turn under the control of SGK1 (Lang et al. Citation2006). SGK1 phosphorylates and thus inhibits GSK3 and is thus expected to counteract the degradation of β-catenin (Lang et al. Citation2006). Enhanced SGK1 expression and/or activity are further expected to increase β-catenin protein abundance, which should result in increased KCNE1/KCNQ1 activity. Along those lines, a gain of function variant of the SGK1 gene is associated with shortened QT (Busjahn et al. Citation2004). Moreover, β-catenin may participate in the SGK1-dependent regulation of the Na+/K+ ATPase (Setiawan et al. Citation2002, Verrey et al. Citation2003, Zecevic et al. Citation2004).
β-catenin degradation is further regulated by the Wnt pathway (Jin et al. Citation2008), which participates in the pathogenesis of polycystic kidney disease, a disorder involving deranged cell proliferation, epithelial polarity and transport (Benzing et al. Citation2007). Polycystic kidney disease is paralleled by excessive formation of β-catenin (Simons and Walz Citation2006, Harris and Torres Citation2011), which is considered to participate in the stimulation of cell proliferation (Peruzzi and Bottaro Citation2006). It is tempting to speculate that β-catenin may similarly contribute to the derangement of transepithelial transport.
In conclusion, β-catenin stimulates the K+ channel complex KCNE1/KCNQ1, an effect independent of its ability to stimulate gene expression. At least in theory, the effect may participate in the pathophysiology of β-catenin-related diseases.
Acknowledgements
This work was supported by the DFG (GRK 1302/1 and SFB 773) and the IZKF of the University of Tübingen (Nachwuchsgruppe). The authors gratefully acknowledge the meticulous preparation of the manuscript by Tanja Loch and Sari Rübe.
Declaration of interest : The authors report no conflicts of interest. The authors alone are responsible for the content and writing of the paper.
References
- Benzing T, Simons M, Walz G. 2007. Wnt signaling in polycystic kidney disease. J Am Soc Nephrol 18:1389–1398.
- Blankesteijn WM, van dS V, ter Horst P, Smits JF. 2008. The Wnt/frizzled/GSK-3 beta pathway: A novel therapeutic target for cardiac hypertrophy. Trends Pharmacol Sci 29:175–180.
- Bohmer C, Sopjani M, Klaus F, Lindner R, Laufer J, Jeyaraj S, 2010. The serum and glucocorticoid inducible kinases SGK1-3 stimulate the neutral amino acid transporter SLC6A19. Cell Physiol Biochem 25:723–732.
- Busjahn A, Seebohm G, Maier G, Toliat MR, Nurnberg P, Aydin A, 2004. Association of the serum and glucocorticoid regulated kinase (sgk1) gene with QT interval. Cell Physiol Biochem 14:135–142.
- Cadigan KM, Liu YI. 2006. Wnt signaling: Complexity at the surface. J Cell Sci 119:395–402.
- Dehner M, Hadjihannas M, Weiske J, Huber O, Behrens J. 2008. Wnt signaling inhibits forkhead box O3a-induced transcription and apoptosis through upregulation of serum- and glucocorticoid-inducible kinase 1. J Biol Chem; 283:19201-19210.
- Eckey K, Strutz-Seebohm N, Katz G, Fuhrmann G, Henrion U, Pott L, 2010. Modulation of human ether a gogo related channels by CASQ2 contributes to etiology of catecholaminergic polymorphic ventricular tachycardia (CPVT). Cell Physiol Biochem 26:503–512.
- Fagotto F, Funayama N, Gluck U, Gumbiner BM. 1996. Binding to cadherins antagonizes the signaling activity of beta-catenin during axis formation in Xenopus. J Cell Biol 132:1105–1114.
- Giles RH, van Es JH, Clevers H. 2003. Caught up in a Wnt storm: Wnt signaling in cancer. Biochim Biophys Acta 1653:1–24.
- Grigoryan T, Wend P, Klaus A, Birchmeier W. 2008. Deciphering the function of canonical Wnt signals in development and disease: Conditional loss- and gain-of-function mutations of beta-catenin in mice. Genes Dev 22:2308–2341.
- Torres VE, Harris PC. 2011. Polycystic kidney disease in 2011: Connecting the dots toward a polycystic kidney disease therapy. Nat Rev Nephrol 8:66-68.
- He TC, Sparks AB, Rago C, Hermeking H, Zawel L, da Costa LT, 1998. Identification of c-MYC as a target of the APC pathway. Science 281:1509–1512.
- Heitzmann D, Koren V, Wagner M, Sterner C, Reichold M, Tegtmeier I, 2007. KCNE beta subunits determine pH sensitivity of KCNQ1 potassium channels. Cell Physiol Biochem 19:21–32.
- Henrion U, Strutz-Seebohm N, Duszenko M, Lang F, Seebohm G. 2009. Long QT syndrome-associated mutations in the voltage sensor of I(Ks) channels. Cell Physiol Biochem 24:11–16.
- Jin T, George Fantus I, Sun J. 2008. Wnt and beyond Wnt: Multiple mechanisms control the transcriptional property of beta-catenin. Cell Signal 20:1697–1704.
- Kanki H, Kupershmidt S, Yang T, Wells S, Roden DM. 2004. A structural requirement for processing the cardiac K+ channel KCNQ1. J Biol Chem 279:33976–33383.
- Korinek V, Barker N, Morin PJ, van Wichen D, de Weger R, Kinzler KW, 1997. Constitutive transcriptional activation by a beta-catenin-Tcf complex in APC-/- colon carcinoma. Science 275:1784–1787.
- Lang F, Bohmer C, Palmada M, Seebohm G, Strutz-Seebohm N, Vallon V. 2006. (Patho)physiological significance of the serum- and glucocorticoid-inducible kinase isoforms. Physiol Rev 86:1151–1178.
- Morin PJ, Sparks AB, Korinek V, Barker N, Clevers H, Vogelstein B, 1997. Activation of beta-catenin-Tcf signaling in colon cancer by mutations in beta-catenin or APC. Science 275:1787–1790.
- Moser AR, Pitot HC, Dove WF. 1990. A dominant mutation that predisposes to multiple intestinal neoplasia in the mouse. Science 247:322–324.
- Naishiro Y, Yamada T, Idogawa M, Honda K, Takada M, Kondo T, 2005. Morphological and transcriptional responses of untransformed intestinal epithelial cells to an oncogenic beta-catenin protein. Oncogene 24:3141–3153.
- Peruzzi B, Bottaro DP. 2006. Beta-catenin signaling: Linking renal cell carcinoma and polycystic kidney disease. Cell Cycle 5:2839–2841.
- Pokutta S, Drees F, Yamada S, Nelson WJ, Weis WI. 2008. Biochemical and structural analysis of alpha-catenin in cell-cell contacts. Biochem Soc Trans 36:141–147.
- Polakis P. 2000. Wnt signaling and cancer. Genes Dev 14:1837–1851.
- Rotte A, Bhandaru M, Ackermann TF, Boini KM, Lang F. 2008. Role of PDK1 in regulation of gastric acid secretion. Cell Physiol Biochem 22:725–734.
- Sakoda H, Gotoh Y, Katagiri H, Kurokawa M, Ono H, Onishi Y, 2003. Differing roles of Akt and serum- and glucocorticoid-regulated kinase in glucose metabolism, DNA synthesis, and oncogenic activity. J Biol Chem 278:25802–25807.
- Seebohm G, Strutz-Seebohm N, Ureche ON, Henrion U, Baltaev R, Mack AF, 2008. Long QT syndrome-associated mutations in KCNQ1 and KCNE1 subunits disrupt normal endosomal recycling of IKs channels. Circ Res 103:1451–1457.
- Setiawan I, Henke G, Feng Y, Bohmer C, Vasilets LA, Schwarz W, 2002. Stimulation of Xenopus oocyte Na(+),K(+)ATPase by the serum and glucocorticoid-dependent kinase sgk1. Pflugers Arch 444:426–431.
- Simons M, Walz G. 2006. Polycystic kidney disease: cell division without a c(l)ue? Kidney Int 70:854–864.
- Sopjani M, Alesutan I, Wilmes J, Dermaku-Sopjani M, Lam RS, Koutsouki E, 2010a. Stimulation of Na+/K+ ATPase activity and Na+ coupled glucose transport by beta-catenin. Biochem Biophys Res Commun 402:467–470.
- Sopjani M, Kunert A, Czarkowski K, Klaus F, Laufer J, Foller M, 2010b. Regulation of the Ca(2+) channel TRPV6 by the kinases SGK1, PKB/Akt, and PIKfyve. J Membr Biol 233:35–41.
- Strutz-Seebohm N, Henrion U, Steinke K, Tapken D, Lang F, Seebohm G. 2009. Serum- and glucocorticoid-inducible kinases (SGK) regulate KCNQ1/KCNE potassium channels. Channels (Austin) 3:88–90.
- Sugden PH, Fuller SJ, Weiss SC, Clerk A. 2008. Glycogen synthase kinase 3 (GSK3) in the heart: A point of integration in hypertrophic signalling and a therapeutic target? A critical analysis. Br J Pharmacol 153(Suppl 1):S137–S153.
- Takayama T, Miyanishi K, Hayashi T, Sato Y, Niitsu Y. 2006. Colorectal cancer: Genetics of development and metastasis. J Gastroenterol 41:185–192.
- Tetsu O, McCormick F. 1999. Beta-catenin regulates expression of cyclin D1 in colon carcinoma cells. Nature 398:422–426.
- Tzahor E. 2007. Wnt/beta-catenin signaling and cardiogenesis: Timing does matter. Dev Cell 13:10–13.
- van Noort M, Meeldijk J, van der ZR, Destree O, Clevers H. 2002. Wnt signaling controls the phosphorylation status of beta-catenin. J Biol Chem 277:17901–17905.
- Verrey F, Summa V, Heitzmann D, Mordasini D, Vandewalle A, Feraille E, 2003. Short-term aldosterone action on Na,K-ATPase surface expression: Role of aldosterone-induced SGK1? Ann NY Acad Sci 986:554–561.
- Zecevic M, Heitzmann D, Camargo SM, Verrey F. 2004. SGK1 increases Na,K-ATP cell-surface expression and function in Xenopus laevis oocytes. Pflugers Arch 448:29–35.
- Zumbrunn J, Kinoshita K, Hyman AA, Nathke IS. 2001. Binding of the adenomatous polyposis coli protein to microtubules increases microtubule stability and is regulated by GSK3 beta phosphorylation. Curr Biol 11:44–49.