Abstract
Listeria monocytogenes, the causative agent of listeriosis, is a virulent foodborne Gram-positive bacterial pathogen, with 20–30% mortality. It has a broad ability to transport iron, either in the form of ferric siderophores, or by extracting it from mammalian iron binding proteins. In this review we focus on the mechanisms of ferric siderophore and haem transport into the listerial cell. Despite the fact that it does not synthesize siderophores, L. monocytogenes transports ferric siderophores in the wild environment by the actions of cytoplasmic membrane ABC-transporter systems. The bacterium acquires haem, on the other hand, by two mechanisms. At low (nanomolar) concentrations, sortase B-dependent, peptidoglycan-anchored proteins scavenge the iron porphyrin in human or animal tissues, and transfer it to the underlying ABC-transporters in the cytoplasmic membrane for uptake. At concentrations at or above 50 nM, however, haem transport becomes sortase-independent, and occurs by direct interactions of the iron porphyrin with the same ABC-transporter complexes. The architecture of the Gram-positive cell envelope plays a fundamental role in these mechanisms, and the haem acquisition abilities of L. monocytogenes are an element of its ability to cause infectious disease.
Introduction
This review article considers the mechanistic biochemistry of membrane iron transport by Gram-positive bacteria, among which Listeria monocytogenes is prototypic. Unlike Gram-negative bacterial cell envelope iron uptake systems, which became understood from extensive in vivo and in vitro biochemical analyses over many years, Gram-positive iron transporters were primarily identified only over the past decade from bioinformatic interpretation of genomic data. The in silico approaches created a catalog of potential transport systems, that were subsequently subject to molecular biological and biochemical characterizations. This different chronology of experimental approaches, and in some cases inaccurate genomic annotations, led to misunderstandings of the underlying transport mechanisms. We endeavor to summarize and explain listerial iron acquisition systems, including currently proposed theories of how iron complexes traverse the Gram-positive cell wall. The functions of the transporters relate to the structural features of their membrane systems, and we devote attention to this aspect. In this sense it is germane that our understanding of the Gram-positive cell surface remains rudimentary. Whereas Gram-negative cells have an outer membrane bilayer in which solute transporters reside and interact with the environment, a matrix of peptigoglycan (PG) envelops Gram-positive bacteria, embedded with covalently bound proteins. With regard to the transport of haem (Hn), a focal point of our paper, these surface proteins constitute its initial binding site and are proposed to transfer the porphyrin to ABC-transporters in the underlying cytoplasmic membrane. This postulated process of sequential exchange between or among iron-binding proteins downward through the PG layer distinguishes Gram-positive bacterial transport systems; at the cytoplasmic membrane stage the membrane transporters are comparable to those of Gram-negative cells. We consider the underpinnings and consequences of such proposed mechanisms with regard to their thermodynamic, kinetic and structural features.
A second point of interest in this topic is that bacterial membrane iron transport relates to the widespread pathogenesis of Gram-positive organisms, among which L. monocytogenes is prototypic of a group that includes Staphylococcus, Streptococcus, Bacillus and Corynebacteria. The extent of mortality from these microbes is already high and it is increasing: Deaths from staphylococcal infections alone are about 10,000 and 4,000 per year in the US and UK, respectively. The known relationship between membrane iron transport and the pathogenesis of these organisms is of interest to human health; we will consider this topic more fully elsewhere (Klebba et al. Submitted).
Bacterial mechanisms of iron uptake
The ability to acquire iron plays a role in the outcome of bacterial infections (Griffiths Citation1993, Tai et al. Citation1993, Furman et al. Citation1994, Rouquette et al. Citation1995, Conte et al. Citation1996, Tsolis et al. Citation1996, Ward et al. Citation1996, Cornelissen et al. Citation1998, Rozalska et al. Citation1998). Yet, besides this long-standing (Bullen Citation1974, Citation1981, Citation1985, Bullen et al. Citation2000) correlation, many fundamental mechanistic questions about bacterial iron uptake remain unanswered. At the cell surface, Gram-negative bacteria contain numerous receptors for uptake of ferric complexes that provide the recognition function of the complicated multicomponent active transport systems of which they are a part. These transporters exist for molecules such as ferric siderophores, as typified by the outer membrane (OM) ferric enterobactin-FepA system (Klebba Citation2004). Gram-positive cells employ fundamentally different iron transport processes, as a result of their different cell envelope architecture. For both Gram-negative and Gram-positive bacteria, the stepwise biochemical reactions of Fe+++ transit through the cell envelope are incompletely resolved. L. monocytogenes may acquire iron in at least four ways: Uptake of elemental iron, utilization of exogenous ferric siderophores, extraction of iron from host binding proteins like Tf/Lf/Ftn, and removal of Hn from host proteins like hemoglobin (Hb), hemopexin (Hpx) or haptoglobin-Hb (Hp-Hb) complexes.
Listerial iron acquisition
The fact that L. monocytogenes actively proliferates within cells and spreads from cell to cell suggests that it adeptly transports iron in the intracellular environment. Often microbial siderophores perform this function, because they rapidly extract iron from Tf (Konopka et al. Citation1982, Konopka and Neilands Citation1984) and Ftn (Tidmarsh et al. Citation1983), availing the metal for transport by invading cells. However, L. monocytogenes does not elaborate siderophores; neither does it encode siderophore biosynthetic systems in its genome (Glaser et al. Citation2001), nor do experimental approaches (chrome azurol S/iron(III)/hexadecyltrimethylammonium bromide (Schwyn and Neilands Citation1987)) reveal siderophore production during iron-deficient growth (X. Jiang, unpublished data). On the other hand, proteins bound to the listerial cell surface function in the uptake of Hn, either free or incorporated into Hb (Jin et al. Citation2005, Xiao et al. Citation2011). Staphylococcus aureus (Mazmanian et al. Citation2003, Torres et al. Citation2006, Zhu et al. Citation2008b), Streptococcus pyogenes (Bates et al. Citation2003, Fisher et al. Citation2008, Liu and Lei Citation2005, Zhu et al. Citation2008a) and Bacillus anthracis (Maresso et al. Citation2008), encode comparable Hn/Hb acquisition systems, reiterating their overall importance to Gram-positive pathogens. The ability to acquire iron from mammalian proteins is a common attribute of pathogenic bacteria (Cornelissen and Sparling Citation1994b), as evidenced by the TonB-dependent Tf/Lf receptors of Neisseria (Cornelissen and Sparling Citation1994a, Gray-Owen and Schryvers Citation1996, Bonnah and Schryvers Citation1998, Schryvers and Stojiljkovic Citation1999), the Hn/Hb-Hp/hHpx uptake systems of Haemophilus (Sanders et al. Citation1994, CitationElkins et al. 1995,1998, Jarosik et al. Citation1995, Stevens et al. Citation1996, Ren et al. Citation1998, Morton et al. Citation1999, Thomas et al. Citation2001, Jin et al. Citation2005) and Neisseriae (Stojiljkovic et al. Citation1997, Richardson and Stojiljkovic Citation1999, Zhu et al. Citation2000), and the broad ability of L. monocytogenes to utilize all known iron mammalian iron sources (). So for Listeria monocytogenes, a dichotomy exists: In the wild, the bacterium relies on siderophores produced by competing microbes and utilizes them as an external iron supply. In the host gut, the bacterium may still transport siderophores produced by other competing microbes, but in blood, tissues and the nervous system a different iron transport process occurs: Recognition, adsorption and uptake of Hn. Ultimately, both ferric siderophores and Hn iron complexes use cytoplasmic membrane ABC-transporters as their means of internalization into the bacterial cell.
Figure 1. Iron nutrition tests with L. monocytogenes EGD-e. Bacteria were grown in BHI broth, rendered iron-deficient with 1 mM bipyridyl, and plated on BHI agar containing bipyridyl (Newton et al. Citation2005). Iron-complexes were applied to the paper discs. EGD-e used siderophores Fc, FcA and FxB [250 uM (discs 1, 4, 7, respectively) 50 uM (discs 2, 5, 8) and 5 uM (discs 3, 6, 9), holoTf and holoLf (discs 10, 11; 6 uM), Hb (disc 13; 15 uM) and Hn (disc 14; 100 uM). Utilization of iron from Ftn (disc 12; 2 uM) and ferric enterobactin (discs 16–18) was not observed here, but seen in other tests (Jin et al. Citation2005, Newton et al. Citation2005). This Figure is reproduced in color in the online version of Molecular Membrane Biology.
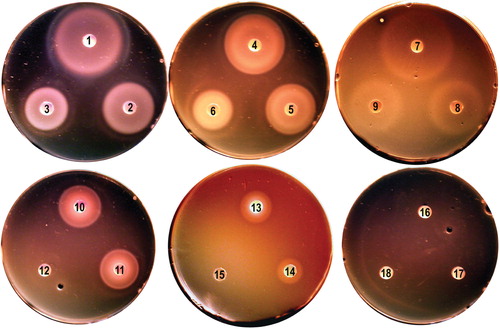
Despite its noted lack of siderophore biosynthesis, L. monocytogenes transports exogenously available ferric siderophores from other organisms (Coulanges et al. Citation1996, Jin et al. Citation2005), and various other iron acquisition systems were also reported. (i) An inducible ferric citrate transport system was found in the prototypic strain EGD-e (Adams et al. Citation1990) as well as (ii) a cell-surface localized reductase that acted on iron-containing catecholamines and siderophores (Deneer et al. Citation1995). The reductase had general specificity for iron containing molecules (Adams et al. Citation1990, Deneer et al. Citation1995, Barchini and Cowart Citation1996). In addition, L. monocytogenes was proposed to recognize catecholamine-iron complexes as siderophore-like compounds, and then reduce the ferric-catecholamines to release ferrous iron intracellularly (Coulanges et al. Citation1997, Citation1998). This ability to utilize neurotransmitter catecholate iron complexes presumably fit with the predilection of the pathogen for nervous system tissues, but no specific surface receptors for such iron forms were ever identified. Instead, the surface reductase was proposed to broadly recognize and reduce different ferric compounds, including ferric catecholamine complexes. These reports on the surface reductase remain indeterminate because it was neither identified by bioinformatic analyses, nor cloned, nor deleted, nor purified and biochemically characterized. On the other hand, more recent bioinformatic and biochemical characterizations of proteins encoded by Fur-regulated chromosomal loci in EGD-e identified other transport systems. (iii) ABC-transporters exist for ferric hydroxamate siderophores and for Hn (Jin et al. Citation2005, Xiao et al. Citation2011). Extensive sequence identity exists between the components of these systems and constituents of other known ABC-transporters (Jin et al. Citation2005). L. monocytogenes acquires from Tf, Lf and Ftn (Hartford et al. Citation1993, Jin et al. Citation2005; ), but by an undetermined process: No orthologs of Gram-negative bacterial Tf receptors were found in listerial genomes. Lastly, L. monocytogenes produces (iv) high affinity, sortase-anchored binding proteins that facilitate uptake of Hn/Hb at low concentrations (Jin et al. Citation2005, Newton et al. Citation2005, Xiao et al. Citation2011; see below).
Competition for iron among siderophores and eukaryotic binding proteins
For several reasons, the ability of neurologically important catecholamines to supply iron to invading microbes is uncertain. Catecholamines like dopamine, epinephrine and nor-epinephrine contain effective (catecholate) groups for iron chelation. They are structurally comparable to DHBA and DHBS, the biosynthetic precursor and degradation product, respectively, of bacterial catecholates [the Gram-negative siderophore enterobactin and its glucosylated form (also called salmochelin) and the Gram-positive siderophore corynebactin (also called bacillibactin)]. Nevertheless, the affinity of monocatecholates for iron (III) is much, much lower than that of bacterial siderophores, for several reasons. The cell cytoplasm is a reducing environment, while extracellular sites like blood, lymph, the bacterial periplasm and exterior are oxidizing environments. In the latter places, free iron (II) relatively rapidly oxidizes to iron (III); the two oxidation states have different complexation preferences. Iron (III) is a hard Lewis acid with a preference for hard Lewis base ligands, like oxygen. The oxygen ligands almost always distribute around ferric iron in a hexadentate complex with octahedral geometry. Accordingly, molecules that inhabit the extracellular environment, including Tf, Lf and siderophores, chelate Fe+++ in this fashion. Iron (II), on the other hand, prefers soft ligands like nitrogen and sulfur, and may form tetracoordinate tetrahedral (e.g., iron-sulfur clusters) or hexacoordinate octahedral (e.g., Hn) biological complexes. In the competition for iron among microbial pathogens and eukaryotic iron sequestration systems, the relative affinity of the various iron complexation agents () mainly determines their ability to capture it in the host environment (although noteworthy exceptions exist (Konopka et al. Citation1982, Konopka and Neilands Citation1984)). Catecholamines may penetrate the complexation sphere of Fe+++ (Arreguin et al. Citation2009, Porter et al. Citation2010), but this activity is not equivalent to siderophore-mediated iron-scavenging in the human body. The reason is that the multiple chelation ligands in a single siderophore molecule dramatically increase its overall affinity for iron. The preference of iron (III) for hexacoordinate geometry mandates three catecholamine molecules in its coordination sphere. But, complexation by three independent catechol moieties does not increase the overall affinity of the hexacoordinate complex beyond that of the monomeric ligands, because any of these individual catechols may independently dissociate from the metal center and diffuse off in solution. The usually trimeric nature of siderophores, on the other hand, prevents a single ligand from leaving the iron center if it dissociates from the metal: The two other complexation groups remain attached and the third ligand may re-associate, preserving the overall integrity of the chelate. Furthermore, once an individual siderophore ligand penetrates the complexation sphere of iron in Tf, Lf or Ftn, its other two ligands have a high probability of displacing the other protein side chains, thereby capturing the metal. Lastly, the often huge affinity of siderophores for Fe+++ iron () acts as a thermodynamic sink that rapidly shifts the ferrous-ferric equilibrium to the right. The upshot of these considerations is that siderophores have much higher affinity and better ability to complex iron than monomeric species like dopamine, epinephrine or norepinephrine. Thus, the trimeric structure of most siderophores confers two advantages relative to their monomeric components or degradation products or catecholamines: It greatly increases the affinity for iron, and it enhances the ability to extract iron from other chelation spheres, like those of eukaryotic binding proteins. Despite these considerations, siderophore activity was observed for both monomeric catechols (2,3-dihydroxy benzoic acid) in Brucella abortus (Lopez-Goni et al. Citation1992) and catecholamines in Bordetella bronchiseptica (Anderson and Armstrong Citation2008, Armstrong et al. Citation2012).
Table I. Affinities of eukaryotic binding proteins and siderophores for iron (III).
The cell envelopes of bacteria
The mechanisms of prokaryotic membrane transport derive from the structures of their respective bacterial cell envelopes. Gram-negative and Gram-positive cell walls were biochemically, immunologically and microscopically characterized over the past half century, especially in the former case, but the past decade of research produced surprises about their overall architecture. The Enterobacteriaceae, like Escherichia coli, Salmonella, Yersinia, Klebsiella, Serratia, Citrobacter and Proteus, as well as related species Vibrio, Neisseria and Haemophilus, possess a trilaminar cell envelope, composed of an inner membrane (IM), outer membrane (OM), and an aqueous space between them, the periplasm. This macromolecular assembly contains the proteinaceous components needed to explain the uptake of metabolic solutes, including sugars, amino acids, nucleotides, vitamins and metals. The majority of Gram-positive bacteria, on the other hand, apparently only have an inner (or cytoplasmic) membrane (CM), surrounded by an assembly of the macromolecular polymer peptidoglycan (PG). Nevertheless, exceptions may exist, like the Corynebacteria (Hoffmann et al. Citation2008, Zuber et al. Citation2008, Marchand et al. Citation2012) and Mycobacteria (Niederweis et al. Citation2010), that contain some sort of an OM that includes porins (Trias et al. Citation1992, Schiffler et al. Citation2007). The cell surface of L. monocytogenes and related Gram-positive species consists of thick sheaths of the PG biopolymer (Dmitriev et al. Citation1999, Navarre and Schneewind Citation1999), to which proteins and other molecules, like techoic acid, covalently or non-covalently associate. However, as we will consider below, in several ways this perceived structure of the Gram-positive cell wall fails to fully explain its physiological transport properties.
Implications of PG structure in the cell wall
Besides its contributions to the physical integrity of the bacterial cells (Yem and Wu Citation1978, Tomasz, Citation1979, Scheurwater and Burrows Citation2011), the structural features of PG are suddenly germane to both Gram-negative and Gram-positive bacterial iron transport processes. Listerial uptake of Hn from animal proteins like Hb involves binding proteins that are covalently bound to PG. For over 40 years the murein polymer was envisioned to surround prokaryotic cells with its glycan [(N-acetyl glucosamine-N-acetyl muramic acid)n; (NAG-NAM)n] chains parallel to the cell surface, and perpendicular to the long axis of the bacterial rod. Microscopically observed PG in the periplasm of Gram-negative bacteria or on the surface Gram-positive bacteria was surmised to contain either a few, or as many as 50 or more of these parallel layers, respectively. PG with glycan chains arranged parallel to the membrane surface was envisioned to assemble and polymerize with a layered architecture. However, an alternative model exists in which the glycan chains organize perpendicular to the plane of the cell membranes (Dmitriev et al. Citation1999, Citation2003, Citation2005). According to this postulate, called the vertical scaffold model, the pentapeptide of PG extends from (NAG-NAM)n backbone in a triskelion pattern that crosslinks into hexagonal cells, creating a honeycomb-like polymer around the bacterial cell ().
Figure 2. Models of PG structure. Left: In (A) the layered model (Holtje Citation1998) predicts oligosaccharide chains perpendicular to the long axis of the bacterial cell and (B) parallel to the cell surface (reprinted with permission from Holtje Citation1998). (C) Cryotomography of purified, frozen-hydrated Gram-negative bacterial (Caulobacter crescentus) sacculi (reprinted with permission from Gan et al. Citation2008). The image represents a 10-nm thick Z-slice through the 3-D reconstruction of a purified sacculus. The inset shows enhanded magnification to show glycan moieties (gl). Right (images reprinted with permission from Meroueh et al. Citation2006): the vertical scaffold model (48, 100), showing the NMR-determined structure of a glycan chain and attached oligopeptide side chains (D), that lead to a hexagonal polymerization (E), creating cells of defined size through the cell wall (F) when the polymer assembles with the NAGNAM chains perpendicular to the cell surface (G). This Figure is reproduced in color in the online version of Molecular Membrane Biology.
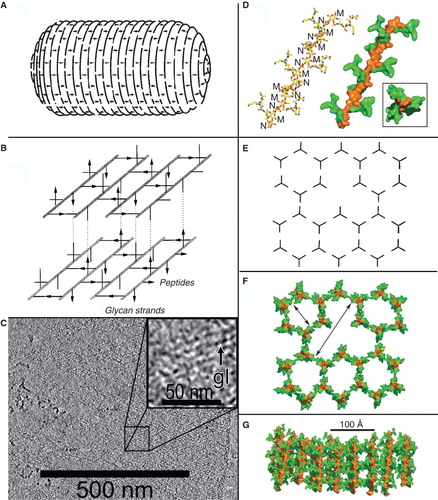
PG contains a repeating disaccharide of β-1,4-linked N-acetylglucosamine (NAG) and N-acetylmuramic acid (NAM), to which oligopeptide chains attach (by amide linkage to the lactyl carboxylic acid group of NAM) and crosslink the glycan strands. Variation exists among different species in the composition of the oligopeptide moiety (in L. monocytogenes, it is the tetrapeptide L-Ala-D-Glu-m-Dap-D-Ala; (Kamisango et al. Citation1982)), the length of glycan polymers, the nature and extent of their crosslinking, and the overall thickness of the PG component. In E. coli, the periplasmic PG layer between the IM and OM is very thin (Labischinski et al. Citation1991, Matias et al. Citation2003; for review see Vollmer et al. Citation2008, Vollmer and Seligman Citation2010), whereas in Gram-positive cells PG forms a dense outer wall, 2- to 10-fold thicker (Matias and Beveridge Citation2007). Unexpectedly, the orientation of glycan chains in PG architecture has come in question, in that the layered model (also called the planar network model (Holtje Citation1998, Koch Citation2000) predicts them parallel to, whereas the vertical scaffold model (Dmitriev et al. Citation2003, Meroueh et al. Citation2006)) predicts them perpendicular to, the cell surface. One ramification of the vertical scaffold model is the formation of a matrix of PG cells with defined, repeating dimensions enveloping the bacterial cells (Meroueh et al. Citation2006). In this new context, the PG layer may create a previously unappreciated physical architecture that facilitates both Gram-negative and Gram-positive iron acquisition (Kaserer et al. Citation2008, Xiao et al. Citation2011; ). Especially because cell envelope proteins of both prokaryotic families covalently or non-covalently associate with PG, its architecture has implications about iron transport mechanisms.
Figure 3. Space-filling depiction of the PG matrix in the vertical scaffold model. The image in was rotated to show the honeycombed PG polymer (brown) from the cell exterior (100). The pores (20–50 Å) of the polymer allow permeation of small molecules like ferric siderophores and Hn, which the lipoprotein binding proteins of ABC transporters adsorb for uptake. Note the similarity to the arrangement of glycans in the cryotomographic section shown in . This Figure is reproduced in color in the online version of Molecular Membrane Biology.
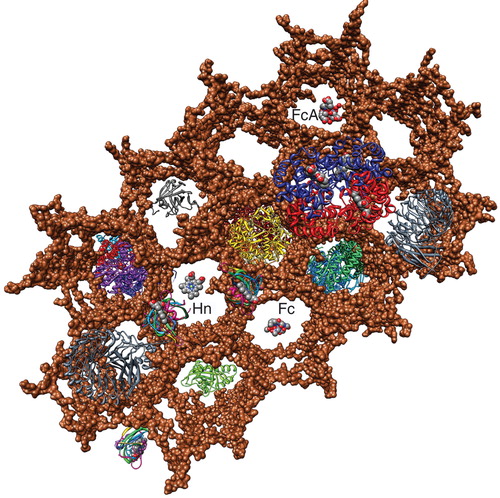
Figure 4. High resolution AFM image of the staphylococcal cell wall. The image shows a network of fibers with large empty spaces. Bar = 50 nm. Reprinted with permission from Touhami et al. (Citation2004).
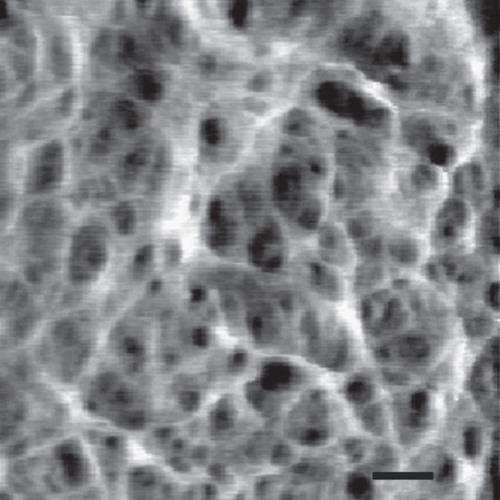
The demonstration of three-fold symmetry in the side-chains attached to NAM was a key discovery that lent support to the vertical scaffold model of PG (Meroueh et al. Citation2006). The solution structure of synthetic NAG-NAM oligomers, determined by NMR, showed three- fold symmetry in the lactyl-oligopeptide component of NAM, intimating the formation of hexagonal cells within the polymer. This finding refuted previous suggestions of two-fold symmetry, or 90 degree angles, etc. (Holtje Citation1998, Koch Citation2000) in the disposition of the oligopeptide component: the NMR results did not concur with such structures. Furthermore, findings from several crystallographic studies confirmed the three-fold symmetry of peptides attached to the glycan chains of PG (Cho et al. Citation2007, Perez-Dorado et al. Citation2007, Citation2010): In these cases, the same framework of the tetrasaccharide occurred in PG bound to cell envelope proteins, vindicating its triskelion structure and its right-handed helical arrangement (Meroueh et al. Citation2006). Therefore, convincing evidence supports the scaffold model. From a conceptual standpoint, a vertical orientation of the sugar oligomers offers physiological and biochemical advantages. L. monocytogenes encodes over 100 anchored cell envelope proteins (Popowska Citation2004), and the organization of PG associated proteins may physically promote a biochemical pathway, or a particular mechanism of solute transfer among such PG-bound components. Finally, the cases of Gram-negative and Gram-positive cell walls may be different. Young summarized the situation (Young Citation2006), and argued that opportunities exist for several orientations of the PG polymer in the cell wall.
Active iron transporters in the OM and IM of Gram-negative cells
As ferric siderophores traverse the Gram-negative cell envelope they first bind to high affinity OM receptor proteins. Because environmental concentrations of ferric siderophores are rather low, usually not exceeding micromolar levels, the avidity of OM proteins for their ferric siderophore ligands determines the overall efficacy of the iron uptake process. The crystal structures of E. coli FepA and FhuA (Ferguson et al. Citation1998, Locher et al. Citation1998, Buchanan et al. Citation1999) revealed a unique structural fold shared among OM siderophore receptors. Biochemical determinations of their properties (Newton et al. Citation1999, Cao et al. Citation2000, Scott et al. Citation2001, Annamalai et al. Citation2004) showed that a subnanomolar affinity for ferric enterobactin and ferrichrome, respectively, confers both efficiency and specificity to iron acquisition. It's noteworthy that this high affinity usually confers monospecificity of OM transporters for particular ferric siderophores, and as a result different Gram-negative bacterial species contain multiple surface receptors for the multitude of microbial iron chelators that may exist in their microenvironment. After passage through the OM, ferric siderophores enter the periplasm, where binding proteins (with slightly lesser affinity) adsorb (Sprencel et al. Citation2000) and deliver them to other transporters in the IM. Several types of IM uptake systems exist (Kuan et al. Citation1995) but ferric siderophores presumably all enter the cytoplasm through ABC-type permeases (Braun and Cole Citation1983, Braun et al. Citation1998). This three-stage transport process describes the uptake of most solutes by Gram-negative bacteria, although many variations on this theme are known (Nikaido and Vaara Citation1985, Saier et al. Citation1999, Saier Citation2000, Dassa and Bouige Citation2001, Aboulwafa et al. Citation2003, Zhang et al. Citation2003, Dassa Citation2011). For ferric siderophores the periplasmic binding protein is essential to the efficiency of the overall solute transport process. Without it, accumulating iron complexes leak back, or are excreted out of the periplasm, through TolC (Newton et al. Citation2010).
Sortase-independent and dependent uptake pathways in Gram-positive cells
Without an OM, and hence, in the absence of high affinity ferric siderophore receptor proteins, how do Gram-positive bacteria acquire sufficient quantities of iron to meet their metabolic needs? Either the cytoplasmic membrane ABC transporters of Gram positive bacteria act as the initial binding site for iron-containing molecules in the environment, or the iron acquisition process of Gram positive organisms entails some type of high affinity iron binding proteins within the PG surface layer. Recent findings demonstrate that L. monocytogenes and its relatives employ both transport mechanisms.
Sortase-independent iron transport.
In L. monocytogenes an iron uptake process was described that utilizes a putative non-specific surface reductase (Deneer et al. Citation1995, Coulanges et al. Citation1997). The challenge to this pathway is that the relevant membrane protein(s) remain(s) unidentified. The listerial genome contains 25 or more potential membrane oxidoreductases (Glaser et al. Citation2001), making the identification of the postulated enzyme a daunting task. For instance, site-directed deletion of the annotated reductase lmo1961 in the fhu operon had no effect on ferric siderophore or Hn uptake (Jin et al. Citation2005, Xiao et al. Citation2011). So, at present an iron reductase remains undiscovered. Conversely, ABC-transporters were identified and experimentally demonstrated in the listerial CM for uptake of ferric siderophores (Sebulsky et al. Citation2000, Jin et al. Citation2005). That is to say, that the fhuBCDG locus, located adjacent to fur at 2.031 Mb in the EGD-e genome, is responsible for the uptake of the hydroxamate iron complexes ferrichrome (Fc), ferrichrome A (FcA) and ferrioxamine B (FxB). Site-directed deletion of genes within this Fur-regulated region completely eliminated the ability to acquire iron from hydroxamate ferric siderophores, and the locus does not encode additional potentially PG-bound proteins. These data confirm that L. monocytogenes and related species directly acquire ferric siderophores from their environments by the activity of cytoplasmic membrane permeases alone. The binding protein, FhuD, of the Fhu membrane permease system manifests a roughly 45 nM affinity for its preferred substrate, FxB, and lower affinities for Fc and FcA (Xiao et al. Citation2011). The transport KM values for these hydroxamate iron complexes are about 10 nM; rates for these iron complexes are approximately 25 pMol/109 cells/min (Jin et al. Citation2005; ).
Table II. Thermodynamic and kinetic parameters of ferric siderophore and Hn transport in L. monocytogenes. Binding KD (nM) and capacity (Cap; pMol/109 cells), and transport KM (nM) and Vmax were determined from studies with [59Fe]-Hn.
These results have some unexpected ramifications. First, the ability to transport FcA () is novel. Gram-negative bacteria readily transport the neutral compound Fc, but they are unable to transport its anionic (3-) relative FcA (Wayne and Neilands Citation1975). Thus, the listerial transporter does not discriminate the two compounds, and recognizes and internalizes hydroxamate ferric siderophores by different biochemical attributes than the Gram-negative bacterial receptor protein. A practical implication of this finding is that apoFcA is inappropriate for iron limitation of L. monocytogenes in nutrition tests (Newton et al. Citation2005). Secondly, the selectivity of the ferric hydroxamate transport system for FxB concurs with the fact that L. monocytogenes is a saprophytic organism that is widespread in nature: FxB originates from the genus Streptomycetes, which contains Gram-positive bacteria predominantly found in soil and decaying vegetation. The ability to transport this iron chelate is therefore of great utility to L. monocytogenes in wild environments.
Just as for ferric siderophores, L. monocytogenes encodes a CM ABC-transporter system for Hn (HupDGC) that functions during its pathogenesis of animal hosts (Jin et al. Citation2005, Xiao et al. Citation2011). Hn directly binds to this Fur-regulated membrane transport system, that resides at 2.499 Mb on the EGD-e chromosome, with KD = 10 nM. The transport KM = 15 nM and VMAX = 23 pMol/min/109 cells for this system (). It is important to note that no obvious barriers exist to Hn diffusion through the PG layer of the Gram-positive cell wall, especially in the vertical scaffold model (), and that microscopic (Matias and Beveridge Citation2007) and biochemical (Demchick and Koch Citation1996) evidence confirms this inference (). The Hn ABC-transporter also illustrates a failing of the genomic approach to identification of membrane transport systems. The original annotation of the EGD-e genome (Glaser et al. Citation2001) identified three Fur-regulated, putative Fc transporters, at 2.274, 2.031 and 2.499 Mb. However, experimental analyses of these systems by site-directed deletions, cloning of their structural genes and purification of the proteins they encode revealed that only one, at 2.031 Mb, primarily functions in ferric hydroxamate uptake. The ABC-transporter at 2.499 Mb (lmo2431–2429) preferentially transports Hn (it was subsequently designated hupDGC), and the function of the putative ABC-transporter at 2.274 (lmo2182–4), closely linked to srtB, remains unknown (Jin et al. Citation2005). Deletion of this locus did not affect ferric hydroxamate, ferric catecholate or Hn acquisition. So, the original annotations of the biochemical functions of two of the three loci were in error, and despite the passage of 7 years, remain uncorrected in both ListiList (http://genolist.pasteur.fr/ListiList/) and NCBI (http://www.ncbi.nlm.nih.gov/gene).
Figure 5. Model of the listerial PG, based on the vertical scaffold model. A schematic drawing of the listerial cell wall, from the crystallographic coordinates of homologous constituents, shows the direct permeation of ferric siderophores (Fc; ferrichrome) and Hn to CM ABC-transporters (red arrows), and sortase-dependent uptake of Hn from Hb through PG-anchored binding proteins (blue arrows). This Figure is reproduced in color in the online version of Molecular Membrane Biology.
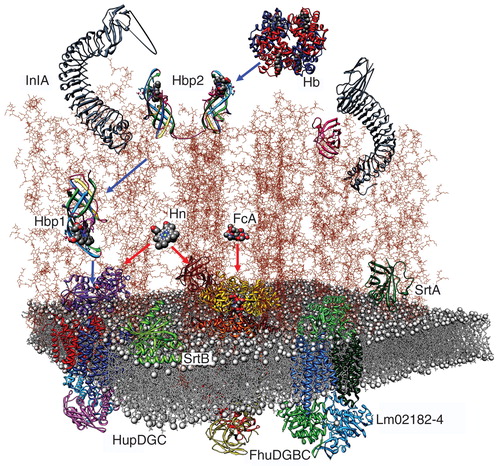
Sortase-dependent Hn acquisition.
The listerial Hn uptake system is novel relative to the listerial ferric siderophore transport process, in that at low substrate concentrations additional proteins, anchored to external PG, bind Hn with higher affinity to begin uptake. A class of novel enzymes called sortases (Schneewind et al. Citation1993, Navarre and Schneewind Citation1994, Citation1999) process secreted proteins in Gram-positive bacteria by concomitantly cleaving off their C-termini and anchoring their N-termini to PG. Sortases cut their target proteins after T in the generic sequences LPxTG (sortase A) or NPxTN (sortase B), and subsequently form a peptide bond between the α-carboxyl of the Thr or Ser in the cleavage site of the secreted protein and the δ- or ε-amino groups of diaminopimelic acid or Lys side chains, respectively, in the pentapeptide of PG (Cossart and Jonquieres Citation2000, Dhar et al. Citation2000, Lebrun et al. Citation1996). Consequently, the extracellular layers of the Gram-positive bacterial cell envelope become permanently decorated with secreted proteins, including Hn-binding proteins (ranging in size from 25–100 kDa). These PG-associated binding proteins initiate the transport of Hn/Hb in the host environment (Jin et al. Citation2005, Torres et al. Citation2006, Hammer and Skaar Citation2011, Xiao et al. Citation2011). Yet, it remains unclear how binding proteins anchored within the PG facilitate the Hn acquisition process. Once bound to such proteins, no obvious driving force exists to transfer Hn/Hb to CM permeases. We will consider this question below.
Surface proteins anchored within PG create previously unknown physiological functions of the cell envelope. Up to six different classes of sortases exist; L. monocytogenes encodes sortases in classes A and B (Spirig et al. Citation2011), that catalyze the linkage of proteins to PG. For example, sortase A (SrtA) recognizes the LPxTG in internalin (Lebrun et al. Citation1996, Dramsi et al. Citation1997) and attaches it to the PG matrix. This widespread mechanism (Ton-That et al. Citation1999, Citation2000) relates to the function of PG-associated proteins in bacterial virulence, as evidenced by the attenuation of srtA mutants in L. monocytogenes (Bierne et al. Citation2002), S. aureus and S. pyogenes (Mazmanian et al. Citation2000, Kharat and Tomasz Citation2003, Lee and Boran Citation2003). A second, iron acquisition-related sorting mechanism in the same species involves the srtB gene product (Autret et al. Citation2001, Bierne et al. Citation2004). In L. monocytogenes SrtB anchors Hbp1 (the product of lmo2186) and Hbp2 (the product of lmo2185) to PG, probably through the motifs NPKSS and NAKTN, respectively (Bierne et al. Citation2004, Mariscotti et al. Citation2009). However, it is mechanistically relevant (see below) that a substantial quantity of these Hn-binding proteins escapes to the extracellular milieu, where they presumably act as hemophores. In S. aureus, sortase B targets another protein implicated in iron uptake, IsdC, through the anchoring domain NPQTN (Mazmanian et al. Citation2002).
As discussed above, at micromolar concentrations Hn traverses the cytoplasmic membrane by the direct action of ABC-transporters, but at lower (nanomolar) external concentrations a cell surface receptor protein facilitates its uptake by initially binding the iron porphyrin. Such binding proteins anchored to the external cell wall were predicted (Mazmanian et al. Citation2002), and later shown to extract the iron porphyrin from Hb (Jin et al. Citation2005, Newton et al. Citation2005, Nobles and Maresso Citation2011, Xiao et al. Citation2011). At low concentrations the sortase-anchored, PG-attached binding proteins are required for efficient Hn transport. So, in a broad sense Gram-positive bacterial Hn/Hb uptake systems encompass both surface receptors and CM transport proteins. Furthermore, besides their sortase-mediated attachment to the PG polymer, the escape or secretion of the same Hn/Hb binding proteins to the external environment (Newton et al. Citation2005) allows them to act as ‘hemophores', that scavenge free Hn (Newton et al. Citation2005, Maresso et al. Citation2008, Xiao et al. Citation2011). Nevertheless, listerial Hn binding proteins only facilitate Hn uptake when when the bacteria face extreme iron deprivation. At such low (nanomolar) concentrations Hbp2 initiates acquisition by recognizing and adsorbing Hn (Xiao et al. Citation2011). On the basis of its structural similarity (see below) Hbp1 may similarly participate, but this possibility was not experimentally demonstrated. The presence of PG-anchored Hbp2 in the cell wall greatly enhances the utilization of externally-supplied Hn-Hbp2 (Xiao et al. Citation2011). Once bound to Hbp1/2, Hn presumably passes from the exterior surface to the CM along a sequential network of the binding proteins, that deliver the iron complex to an ABC transporter complex in the CM bilayer (Liu and Lei Citation2005, Nygaard et al. Citation2006, Pilpa et al. Citation2009). The Hn-binding proteins and the underlying CM ABC transporters have nanomolar affinities for the iron porphyrin (). So, at high external concentrations, the CM permeases may directly adsorb Hn, and at low external concentrations they receive it by unknown mechanisms from the external network of binding proteins, in both cases ultimately internalizing Hn as a result of ATP hydrolysis (Jin et al. Citation2005, Nygaard et al. Citation2006; ).
Hn-binding NEAT-domain proteins
The listerial cell envelope proteins of interest to the Hn/Hb binding reaction are Hbp1 and Hbp2. Homologous proteins exist in Staphylococcus aureus (IsdA, B, C and H), Streptococcus pyogenes (Shr, Shp) and in Bacillus anthracis (IsdX1 and X2; Maresso and Schneewind Citation2006). This family of Gram-positive bacterial antigens contains diverse overall architectures (), but all its constituents have structurally conserved, approximately 125-residue regions (NEAT domains; Andrade et al. Citation2002) that tightly complex Hn. NEAT domains were so designated from bioinformatic analyses of Gram-positive bacterial genomes, that identified their structural genes and localized them near Fur-regulated loci encoding iron transporters (Andrade et al. Citation2002). As noted, NEAT-domain-containing proteins either anchor to the PG layer by sortases A and B; (Mazmanian et al. Citation1999, Ton-That et al. Citation1999, Bierne et al. Citation2004), or pass to the external environment (Newton et al. Citation2005, Maresso et al. Citation2008, Xiao et al. Citation2011). Besides this dual localization, different members of the group were reported to target Hn, Hb or Hp as their ligands, so their exact disposition and specificity remains the subject of conjecture.
Figure 6. Hn/Hb binding proteins of Gram (+) bacteria. (A) The srtB region of the L. monocytogenes chromosome contains proteins with either sortaseB (purple loci) and sortaseA (light purple loci) recognition sites. (B) The full-length sequences of IsdA (354 aa), B (645 aa), C (227 aa) and H (895 aa) of S. aureus show homology to Hbp1 (207aa) and Hbp2 (569 aa) of L. monocytogenes. In a CLUSTALW comparison, the ∼ 125 residue NEAT domains (boxed regions) of IsdA, C, Hbp1 and the two NEAT-domains of IsdB or Hbp2 are aligned (once or more) to the three predicted NEAT-domains of IsdH (highlighted green). (C) Homology in NEAT3 of IsdH. Identical amino acids are highlighted blue; black stars mark identity between IsdB and H; red stars mark identity between IsdA or C and Hbp1 or 2; white stars mark identity between IsdB or H and IsdA or C or Hbp1 or 2. NEAT domains of IsdA, C and NEAT3 of IsdH were crystallographically solved, allowing denotation of their secondary structures (8 β-strands and 1 short α-helix; boxed in colors); Hn-contact residues (and identical a.a in other proteins) are highlighted yellow. IsdB (NEAT2) and H (NEAT3) are 58% identical; IsdA, C, Hbp1, 2 and IsdH (NEAT3) are 49% identical. This Figure is reproduced in color in the online version of Molecular Membrane Biology.
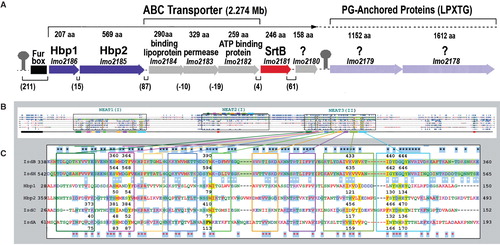
From their polypeptide sequences NEAT-domains fall into in four groups (Andrade et al. Citation2002). These are speculative categories, in that the majority of their proteins are not yet structurally solved; in some cases the original classifications may require revision. But, the bioinformatic analysis identified a family of paralogous or orthologous protein receptors () containing nearly identical structural domains () that complex Hn (not ferric siderophores, as originally assumed; Andrade et al. Citation2002), and interact with Hn-containing eukaryotic proteins. X-ray crystallographic or NMR structural determinations confirm complexation of Hn by IsdA (Grigg et al. Citation2007), IsdC (Sharp et al. Citation2007), and IsdH (NEAT1 and 3; Pilpa et al. Citation2009). The extent of sequence identity in Hn-contact residues among 6 such NEAT1-containing proteins is ∼60% (), which assures conservation of their basic structural motifs (Ginalski Citation2006). Other Hn/Hb binding proteins exist in Gram-negative bacteria, typified by the hemophore HasA (Letoffe et al. Citation2001), but aside from a similar molecular mass (∼20 kDa), they are completely distinct in structural organization from the Isd/Hbp proteins.
Figure 7. Structure of NEAT-domains. Three protein domains (IsdA, isdC, IsdH are blue, yellow and green, respectively), aligned by the MATCH algorithm of CHIMERA (UCSF), are illustrated in ribbon format with ligand contact residues (noted in ) displayed in stick form, in the absence (A) and presence (B) of Hn. This Figure is reproduced in color in the online version of Molecular Membrane Biology.
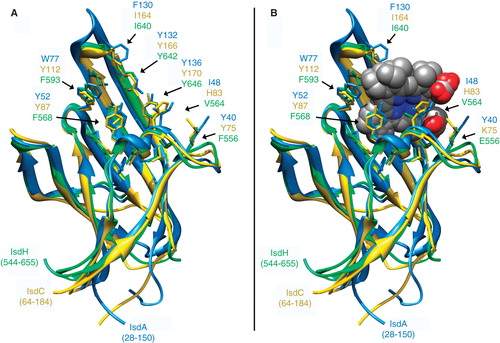
In light of the participation of PG-anchored Hn-binding proteins in Hn uptake the question arises: How do external binding proteins achieve an influx of Hn to the underlying ABC transporters in the CM? The Hn/Hb binding proteins are passively suspended in the extracellular matrix, at fixed distances from the bacterial CM, collecting low concentrations of Hn in the environment as a result of their high affinity (KD = 10 nM) interactions with the iron porphyrin. Once associated with the network of extracellular Hn/Hb binding proteins, that ostensibly decorate the PG matrix like leaves on a tree, no obvious rationale for Hn to progress deeper into the cell wall to the CM permease (). Two explanations may provide insight into the question. First, transport rate is a relevant consideration. In environments with only low concentrations of Hn the rate of its direct binding and uptake by CM ABC-transporters may be insufficient to sustain a significant bacterial growth. High affinity Hn binding to a network of abundant, nearby proteins will enhance its concentration near the cell surface, accelerating the rate of its transport by CM permeases. For L. monocytogenes the high affinity binding equilibrium created by PG-associated Hbp1 and Hbp2 may locally increase the amount of Hn in the PG layer, raising its concentration from nanomolar external levels to a more effective range for the Hup permease (KM = 2 nM, so at 20 nM uptake velocity = Vmax). Secondly, attachment to the PG matrix may organize Hn-binding proteins into a sequential pathway that leads to the Hup transporters in the CM (). The PG polymer is porous to molecules up to 50 kDa (Demchick and Koch Citation1996), so it permits the diffusion of small molecules like Hn. High resolution microscopic images show the loosely linked PG matrix around Gram-positive bacteria (Touhami et al. Citation2004), leaving no doubt about its permeability to molecules as large as 50 kD (). With regard to the different proposed configurations of PG constituents, either orientation of glycan chains explains this property: The vertical scaffold model creates regularly arrayed gaps and aligned cells through the cell wall; the layered model predicts similar, but less organized permeability venues. The important point is that PG creates a framework within which functionally related macromolecules may assemble to form a spatially, temporally and thermodynamically viable pathway from the exterior to the CM. This proposed organization has kinetic advantages, similar to those created by the association of different enzymes together to create a multifunctional complex (e.g., the pyruvate dehydrogenase complex; Roche and Hiromasa Citation2007). Furthermore, the notion facilitates the proposed sequence of protein-mediated acquisition of Hn from Hb by S. aureus, in which sortase A-anchored proteins IsdA, IsdB and IsdH extract the porphyrin from the globin and convey/transfer it to sortase B-anchored IsdC, which delivers the Hn to the HtsABC permease in the CM (Torres et al. Citation2006, Grigg et al. Citation2010).
Perhaps the most important ramification of both PG structural models is that neither poses a barrier to the diffusion of small molecules like ferric siderophores and Hn. The particulars of the two PG schemes, for example layer stratification in the planar network model, and regularly arrayed tunnels or portals to the cytoplasmic membrane in the vertical scaffold model, may provide frameworks for the localization of Hn/Hb binding proteins, leading to vectorial Hn transfer mechanisms. But, aside from conceptual postulates, little or no data currently exists to support these ideas.
The multiplicity of Hn/Hb binding proteins in the staphylococcal cell wall, including sortase A-dependent IsdA, B, and H, and sortase B-dependent IsdC, raises the question of whether a comparable multi-protein uptake system exists in L. monocytogenes? Besides Hbp1 and 2, the presence of two genes that encode LPXTG-containing, and hence potentially SrtA-anchored proteins, in its srtB region () underscores this possibility. Lmo2178 and Lmo2179 contain the sortase A recognition sequences LPSTG and LPHTG, respectively, but they do not manifest convincing sequence homology in the structurally relevant, haem-binding site of the NEAT (II) domains of Hbp1 and Hbp2. In particular, they lack relatedness seen in the ligand contact-residues of other known haem-binding NEAT-domain proteins (): Among 11 such residues, only one is conserved in Lmo2178, and 3 in Lmo2179 (data not shown). Furthermore, neither structural gene is Fur-regulated, and biochemical characterizations of a ΔsrtA derivative of EGD-e revealed no deficiencies in Hn uptake, nor any obvious defects in Hb utilization (Xiao et al. Citation2011). At present Hbp2 is the only sortase-anchored cell envelope protein that was directly implicated in listerial Hn acquisition. The activity of other NEAT-domain or sortase A-dependent proteins in this process remains hypothetical.
Hn homeostasis
Despite the demonstrated function of NEAT-domain proteins in listerial iron acquisition, an alternative or additional explanation exists for the presence of Hn-binding proteins in the PG layer enveloping Gram-positive bacterial cells: Detoxification. Hn is harmful at high concentrations. Its extreme hydrophobicity confers membrane permeability, and high external concentrations may translate into lethal cellular levels, from damage to lipids, proteins and DNA as a result of the generation of reactive oxygen species (Ascenzi et al. Citation2005). Many bacteria express specific mechanisms for detoxification of Hn. Bacterial cell envelope Hn binding proteins (Nhien et al. Citation2010, Roden et al. Citation2012), as well as intracellular Hn-binding proteins (Kaur and Wilks Citation2007) and superoxide dismutases (Negari et al. Citation2008) provide protection against Hn toxicity in insect and animal host environments, where tissue damage from pathogens may include RBC lysis, Hb degradation and consequent high Hn levels. Regulation of intracellular Hn is essential to bacterial metabolism, and besides systems for its uptake, a variety of Gram-positive bacteria, including L. monocytogenes (McLaughlin et al. Citation2012), Corynebacterium diptheria (Bibb and Schmitt Citation2010), S. aureus (Stauff et al. Citation2008, Anzaldi and Skaar Citation2010) and Lactococcus lactis (Lechardeur et al. Citation2012), also encode membrane transport systems for its export. The listerial Fur-regulated locus frvA, that likely also encodes a Hn-exporter (Mclaughlin et al. Citation2012), is essential for virulence and for resistance to Hn-mediated toxicity. Therefore, the possibility exists that secreted and externally located, PG-anchored Hbp1/2 may prevent excessive intracellular accumulation of Hn, by binding the porphyrin and thereby preventing its unregulated diffusion through the CM into the cell.
Overview of iron transport through the listerial cell envelope
Combined with insight into PG architecture discussed above, a model of the Gram-positive cell wall emerges with proteins attached at various locations and distances from the CM (). In the wild environment ferric siderophores simply diffuse through the outer cell wall PG until they encounter appropriate ABC-transporters in the CM, which serve as high-affinity uptake systems (e.g., FhuDCBG, KM ≈ 10 nM). The details of the host environment that successful pathogens must overcome are sketchy. What are the pertinent iron- or Hn-containing macromolecules in these sites? The elaboration of Hn binding proteins by L. monocytogenes into the extracellular environment and anchored to the PG matrix surrounding the cell argues for their central role in iron acquisition in vivo. The concentrations of free Hn in tissues, whole blood, serum, and at sites of bacterial infection or abscess are not well defined, which complicates our understanding of the relationship between Hn uptake and microbial infection. L. monocytogenes is beta-hemolytic, so at its sites of infection or abscess at least Hb is present, potentially Hn as well, and therefore Hp and Hpx too. Hn may enter listerial cells by direct permeation through PG to its CM ABC-transporter (HupDGC; KD ≈ 10 nM), or at lower concentrations follow a binding-protein mediated pathway that begins on the cell surface and passes the porphyrin along a sequential network of binding proteins, secreted or anchored by SrtB. The PG polymer may create a barrier to diffusion of the α2β2 Hb tetramer (mw = 64 kD), and sortase B-anchored proteins Hbp1 and Hbp2 (as well as the orthologous NEAT proteins IsdA, B, C and H of S. aureus (Torres et al. Citation2006, Stauff and Skaar Citation2009) and Shr and Shp of S. pyogenes (Lei et al. Citation2002, Liu and Lei Citation2005, Nygaard et al. Citation2006, Zhu et al. Citation2008a, Citation2008b) are proposed to bind the protein in the media or on the cell surface and extract its Hn. Therefore, in the host environment listerial Hn/Hb uptake may occur by two pathways: A sortase-independent route that predominates at Hn concentrations above 50 nM, and a high-affinity, sortase-dependent system that functions in the nanomolar concentration range. The latter system may entail a particular architecture of participant proteins that enables direct, physical interactions among PG-anchored Hn-binding proteins. These associations result in extraction of the porphyrin from Hb, its subsequent directional transfer to the HtsABC CM transporter, and final internalization into the cytoplasm, where the porphyrin either enters metabolic/biosynthetic pools or is degraded to free its iron for other metabolic or regulatory purposes.
In the listerial CM the HupDGC permease internalizes Hn into the cytoplasm (Jin et al. Citation2005, Xiao et al. Citation2011), directly or indirectly as described above. It is noteworthy that while individual site- directed deletions in the hupDGC locus and the complete operon deletion drastically decreased Hn utilization by EGD-e, the effect was not absolute. Nutrition tests revealed a small amount of residual Hn-promoted growth of L. monocytogenes, indicating the presence of an alternate, secondary Hn transporter. The same deletions increased LD50 of strain EGD-e in the mouse infection model system (100-fold for ΔhupDGC), whereas Δhbp1, Δhbp2 (Jin et al. Citation2005, Newton et al. Citation2005, Xiao et al. Citation2011) and ΔsrtB (Weiss et al. Citation2004, Jin et al. Citation2005, Newton et al. Citation2005, Xiao et al. Citation2011) had no effect on bacterial virulence. Together the results suggest that direct uptake by HupDGC constitutes both the primary listerial Hn transport pathway and the primary virulence determinant related to iron acquisition. The biochemical activities of PG-anchored binding proteins play a secondary role, in the sense that they only function at low concentrations. In light of their ability to scavenge miniscule concentrations of Hn in the host, the contributions of these proteins to listerial pathogenesis bears further investigation.
Acknowledgements
The research was supported by National Institutes of Health (NIH) grant GM53836 and National Science Foundation (NSF) grant MCB0417694 to PEK and SMN, and funds from Trellis Biosciences, S. San Francisco, CA, USA. The funders had no role in experimental design, data collection and analysis, decision to publish, or preparation of the manuscript.
Declaration of interest: The authors report no conflicts of interest. The authors alone are responsible for the content and writing of the paper.
References
- Aboulwafa M, Chung YJ, Wai HH, Saier MH Jr. 2003. Studies on the Escherichia coli glucose-specific permease, PtsG, with a point mutation in its N-terminal amphipathic leader sequence. Microbiology 149:763–771.
- Adams TJ, Vartivarian S, Cowart RE. 1990. Iron acquisition systems of Listeria monocytogenes. Infect Immun 58:2715–2718.
- Aisen P, Leibman A, Zweier J. 1978. Stoichiometric and site characteristics of the binding of iron to human transferrin. J Biol Chem 253:1930–1937.
- Anderson MT, Armstrong SK. 2008. Norepinephrine mediates acquisition of transferrin-iron in Bordetella bronchiseptica. J Bacteriol 190:3940–3947.
- Andrade MA, Ciccarelli FD, Perez-Iratxeta C, Bork P. 2002. NEAT: A domain duplicated in genes near the components of a putative Fe3+ siderophore transporter from Gram-positive pathogenic bacteria. Genome Biol 3:Research 0047.
- Annamalai R, Jin B, Cao Z, Newton SM, Klebba PE. 2004. Recognition of ferric catecholates by FepA. J Bacteriol 186:3578–3589.
- Anzaldi LL, Skaar EP. 2010. Overcoming the heme paradox: Heme toxicity and tolerance in bacterial pathogens. Infect Immun 78:4977–4989.
- Armstrong SK, Brickman TJ, Suhadolc RJ. 2012. Involvement of multiple distinct Bordetella receptor proteins in the utilization of iron liberated from transferrin by host catecholamine stress hormones. Mol Microbiol 84:446–462.
- Arreguin S, Nelson P, Padway S, Shirazi M, Pierpont C. 2009. Dopamine complexes of iron in the etiology and pathogenesis of Parkinson's disease. J Inorg Biochem 103:87–93.
- Ascenzi P, Bocedi A, Visca P, Altruda F, Tolosano E, Beringhelli T, 2005. Hemoglobin and heme scavenging. IUBMB Life 57:749–759.
- Autret N, Dubail I, Trieu-Cuot P, Berche P, Charbit A. 2001. Identification of new genes involved in the virulence of Listeria monocytogenes by signature-tagged transposon mutagenesis. Infect Immun 69:2054–2065.
- Barchini E, Cowart RE. 1996. Extracellular iron reductase activity produced by Listeria monocytogenes. Arch Microbiol 166:51–57.
- Bates CS, Montanez GE, Woods CR, Vincent RM, Eichenbaum Z. 2003. Identification and characterization of a Streptococcus pyogenes operon involved in binding of hemoproteins and acquisition of iron. Infect Immun 71:1042–1055.
- Bibb LA, Schmitt MP. 2010. The ABC transporter HrtAB confers resistance to hemin toxicity and is regulated in a hemin-dependent manner by the ChrAS two-component system in Corynebacterium diphtheriae. J Bacteriol 192:4606–4617.
- Bierne H, Garandeau C, Pucciarelli MG, Sabet C, Newton SM, Del Portillo FG, 2004. Sortase B, a new class of sortase in Listeria monocytogenes. J Bacteriol 186:1972–1982.
- Bierne H, Mazmanian SK, Trost M, Pucciarelli MG, Liu G, Dehoux P, 2002. Inactivation of the srtA gene in Listeria monocytogenes inhibits anchoring of surface proteins and affects virulence. Mol Microbiol 43:869–881.
- Bluhm ME, Hay BP, Kim SS, Dertz EA, Raymond KN. 2002a. Corynebactin and a serine trilactone based analogue: Chirality and molecular modeling of ferric complexes. Inorg Chem 41:5475–5478.
- Bluhm ME, Kim SS, Dertz EA, Raymond KN. 2002b. Corynebactin and enterobactin: Related siderophores of opposite chirality. J Am Chem Soc 124:2436–2437.
- Bonnah RA, Schryvers AB. 1998. Preparation and characterization of Neisseria meningitidis mutants deficient in production of the human lactoferrin-binding proteins LbpA and LbpB. J Bacteriol 180:3080–3090.
- Braun G, Cole ST. 1983. Molecular characterization of the gene coding for major outer membrane protein OmpA from Enterobacter aerogenes. Eur J Biochem 137:495–500.
- Braun V, Hantke K, Koster W. 1998. Bacterial iron transport: Mechanisms, genetics, and regulation. Met Ions Biol Syst 35:67–145.
- Buchanan SK, Smith BS, Venkatramani L, Xia D, Esser L, Palnitkar M, 1999. Crystal structure of the outer membrane active transporter FepA from Escherichia coli [see comments]. Nat Struct Biol 6:56–63.
- Bullen J, Griffiths E, Rogers H, Ward G. 2000. Sepsis: The critical role of iron. Microbes Infect 2:409–415.
- Bullen JJ. 1974. Proceedings: Iron and infection. Br J Haematol 28:139–140.
- Bullen JJ. 1981. The significance of iron in infection. Rev Infect Dis 3:1127–1138.
- Bullen JJ. 1985. Iron and infection. Eur J Clin Microbiol 4:537–539.
- Cao Z, Qi Z, Sprencel C, Newton SM, Klebba PE. 2000. Aromatic components of two ferric enterobactin binding sites in Escherichia coli fepA. Mol Microbiol 37:1306–1317.
- Cho S, Wang Q, Swaminathan CP, Hesek D, Lee M, Boons GJ, 2007. Structural insights into the bactericidal mechanism of human peptidoglycan recognition proteins. Proc Natl Acad Sci USA 104:8761–8766.
- Conte MP, Longhi C, Polidoro M, Petrone G, Buonfiglio V, Di Santo S, 1996. Iron availability affects entry of Listeria monocytogenes into the enterocytelike cell line Caco-2. Infect Immun 64:3925–3929.
- Cornelissen CN, Kelley M, Hobbs MM, Anderson JE, Cannon JG, Cohen MS, 1998. The transferrin receptor expressed by gonococcal strain FA1090 is required for the experimental infection of human male volunteers. Mol Microbiol 27:611–616.
- Cornelissen CN, Sparling PF. 1994a. Identification of receptor-mediated transferrin-iron uptake mechanism in Neisseria gonorrhoeae. Methods Enzymol 235:356–363.
- Cornelissen CN, Sparling PF. 1994b. Iron piracy: Acquisition of transferrin-bound iron by bacterial pathogens. Mol Microbiol 14:843–850.
- Cossart P, Jonquieres R. 2000. Sortase, a universal target for therapeutic agents against gram-positive bacteria? Proc Natl Acad Sci USA 97:5013–5015.
- Coulanges V, Andre P, Vidon DJ. 1996. Esculetin antagonizes iron-chelating agents and increases the virulence of Listeria monocytogenes. Res Microbiol 147:677–685.
- Coulanges V, Andre P, Vidon DJ. 1998. Effect of siderophores, catecholamines, and catechol compounds on Listeria spp. Growth in iron-complexed medium. Biochem Biophys Res Commun 249:526–530.
- Coulanges V, Andre P, Ziegler O, Buchheit L, Vidon DJ. 1997. Utilization of iron-catecholamine complexes involving ferric reductase activity in Listeria monocytogenes. Infect Immun 65:2778–2785.
- Dassa E. 2011. Natural history of ABC systems: Not only transporters. Essays Biochem 50:19–42.
- Dassa E, Bouige P. 2001. The ABC of ABCS: A phylogenetic and functional classification of ABC systems in living organisms. Res Microbiol 152:211–229.
- Demchick P, Koch AL. 1996. The permeability of the wall fabric of Escherichia coli and Bacillus subtilis. J Bacteriol 178:768–773.
- Deneer HG, Healey V, Boychuk I. 1995. Reduction of exogenous ferric iron by a surface-associated ferric reductase of Listeria spp. Microbiology 141:1985–1992.
- Dhar G, Faull KF, Schneewind O. 2000. Anchor structure of cell wall surface proteins in Listeria monocytogenes. Biochemistry 39:3725–3733.
- Dmitriev B, Toukach F, Ehlers S. 2005. Towards a comprehensive view of the bacterial cell wall. Trends Microbiol 13:569–574.
- Dmitriev BA, Ehlers S, Rietschel ET. 1999. Layered murein revisited: A fundamentally new concept of bacterial cell wall structure, biogenesis and function. Med Microbiol Immunol (Berl) 187:173–181.
- Dmitriev BA, Toukach FV, Schaper KJ, Holst O, Rietschel ET, Ehlers S. 2003. Tertiary structure of bacterial murein: The scaffold model. J Bacteriol 185:3458–3468.
- Dramsi S, Dehoux P, Lebrun M, Goossens PL, Cossart P. 1997. Identification of four new members of the internalin multigene family of Listeria monocytogenes EGD. Infect Immun 65:1615–1625.
- Elkins C, Chen CJ, Thomas CE. 1995. Characterization of the hgbA locus encoding a hemoglobin receptor from Haemophilus ducreyi. Infect Immun 63:2194–2200.
- Elkins C, Totten PA, Olsen B, Thomas CE. 1998. Role of the Haemophilus ducreyi Ton system in internalization of heme from hemoglobin. Infect Immun 66:151–160.
- Ferguson AD, Hofmann E, Coulton JW, Diederichs K, Welte W. 1998. Siderophore-mediated iron transport: Crystal structure of FhuA with bound lipopolysaccharide [see comments]. Science 282:2215–2220.
- Fisher M, Huang YS, Li X, Mciver KS, Toukoki C, Eichenbaum Z. 2008. Shr is a broad-spectrum surface receptor that contributes to adherence and virulence in group A streptococcus. Infect Immun 76:5006–5015.
- Furman M, Fica A, Saxena M, Di Fabio JL, Cabello FC. 1994. Salmonella typhi iron uptake mutants are attenuated in mice. Infect Immun 62:4091–4094.
- Gan L, Chen S, Jensen GJ. 2008. Molecular organization of Gram-negative peptidoglycan. Proc Nat Acad Sci USA 105:18953–18957.
- Ginalski K. 2006. Comparative modeling for protein structure prediction. Curr Opin Struct Biol 16:172–177.
- Glaser P, Frangeul L, Buchrieser C, Rusniok C, Amend A, Baquero F, 2001. Comparative genomics of Listeria species. Science 294:849–852.
- Gray-Owen SD, Schryvers AB. 1996. Bacterial transferrin and lactoferrin receptors. Trends Microbiol 4:185–191.
- Griffiths E. 1993. Iron and infection: Better understanding at the molecular level but little progress on the clinical front [editorial]. J Med Microbiol 38:389–390.
- Grigg JC, Ukpabi G, Gaudin CF, Murphy ME. 2010. Structural biology of heme binding in the Staphylococcus aureus Isd system. J Inorg Biochem 104:341–348.
- Grigg JC, Vermeiren CL, Heinrichs DE, Murphy ME. 2007. Haem recognition by a Staphylococcus aureus NEAT domain. Mol Microbiol 63:139–149.
- Hammer ND, Skaar EP. 2011. Molecular mechanisms of Staphylococcus aureus iron acquisition. Annu Rev Microbiol 65:129–147.
- Hartford T, O'Brien S, Andrew PW, Jones D, Roberts IS. 1993. Utilization of transferrin-bound iron by Listeria monocytogenes. FEMS Microbiol Lett 108:311–318.
- Hoffmann C, Leis A, Niederweis M, Plitzko JM, Engelhardt H. 2008. Disclosure of the mycobacterial outer membrane: Cryo-electron tomography and vitreous sections reveal the lipid bilayer structure. Proc Natl Acad Sci USA 105:3963–3967.
- Holtje JV. 1998. Growth of the stress-bearing and shape-maintaining murein sacculus of Escherichia coli. Microbiol Mol Biol Rev 62:181–203.
- Jarosik GP, Maciver I, Hansen EJ. 1995. Utilization of transferrin-bound iron by Haemophilus influenzae requires an intact tonB gene. Infect Immun 63:710–713.
- Jin B, Newton SM, Shao Y, Jiang X, Charbit A, Klebba PE. 2005. Iron acquisition systems for ferric hydroxamates, haemin and haemoglobin in Listeria monocytogenes. Mol Microbiol 59:1185–1198.
- Kamisango K, Saiki I, Tanio Y, Okumura H, Araki Y, Sekikawa I, 1982. Structures and biological activities of peptidoglycans of Listeria monocytogenes and Propionibacterium acnes. J Biochem 92:23–33.
- Kaserer WA, Jiang X, Xiao Q, Scott DC, Bauler M, Copeland D, 2008. Insight from TonB hybrid proteins into the mechanism of iron transport through the outer membrane. J Bacteriol 190:4001–4016.
- Kaur AP, Wilks A. 2007. Heme inhibits the DNA binding properties of the cytoplasmic heme binding protein of Shigella dysenteriae (ShuS). Biochemistry 46:2994–3000.
- Kharat AS, Tomasz A. 2003. Inactivation of the srtA gene affects localization of surface proteins and decreases adhesion of Streptococcus pneumoniae to human pharyngeal cells in vitro. Infect Immun 71:2758–2765.
- Klebba PE. 2004. Transport biochemistry of FepA. Washington: DC: ASM Press.
- Koch AL. 2000. Length distribution of the peptidoglycan chains in the sacculus of Escherichia coli. J Theor Biol 204:533–541.
- Konopka K, Bindereif A, Neilands JB. 1982. Aerobactin-mediated utilization of transferrin iron. Biochemistry 21:6503–6508.
- Konopka K, Neilands JB. 1984. Effect of serum albumin on siderophore-mediated utilization of transferrin iron. Biochemistry 23:2122–2127.
- Kuan G, Dassa E, Saurin W, Hofnung M, Saier MH Jr. 1995. Phylogenetic analyses of the ATP-binding constituents of bacterial extracytoplasmic receptor-dependent ABC-type nutrient uptake permeases. Res Microbiol 146:271–278.
- Labischinski H, Goodell EW, Goodell A, Hochberg ML. 1991. Direct proof of a ‘more-than-single-layered' peptidoglycan architecture of Escherichia coli W7: A neutron small-angle scattering study. J Bacteriol 173:751–756.
- Lebrun M, Mengaud J, Ohayon H, Nato F, Cossart P. 1996. Internalin must be on the bacterial surface to mediate entry of Listeria monocytogenes into epithelial cells. Mol Microbiol 21:579–592.
- Lechardeur D, Cesselin B, Liebl U, Vos MH, Fernandez A, Brun C, 2012. Discovery of intracellular heme-binding protein HrtR, which controls heme efflux by the conserved HrtB-HrtA transporter in Lactococcus lactis. J Biol Chem 287:4752–4758.
- Lee SF, Boran TL. 2003. Roles of sortase in surface expression of the major protein adhesin P1, saliva-induced aggregation and adherence, and cariogenicity of Streptococcus mutans. Infect Immun 71:676–681.
- Lei B, Smoot LM, Menning HM, Voyich JM, Kala SV, Deleo FR, 2002. Identification and characterization of a novel heme-associated cell surface protein made by Streptococcus pyogenes. Infect Immun 70:4494–4500.
- Letoffe S, Deniau C, Wolff N, Dassa E, Delepelaire P, Lecroisey A, 2001. Haemophore-mediated bacterial haem transport: Evidence for a common or overlapping site for haem-free and haem-loaded haemophore on its specific outer membrane receptor. Mol Microbiol 41:439–450.
- Liu M, Lei B. 2005. Heme transfer from streptococcal cell surface protein Shp to HtsA of transporter HtsABC. Infect Immun 73:5086–5092.
- Locher KP, Rees B, Koebnik R, Mitschler A, Moulinier L, Rosenbusch JP, 1998. Transmembrane signaling across the ligand-gated FhuA receptor: Crystal structures of free and ferrichrome-bound states reveal allosteric changes. Cell 95:771–778.
- Lopez-Goni I, Moriyon I, Neilands JB. 1992. Identification of 2,3-dihydroxybenzoic acid as a Brucella abortus siderophore. Infect Immun 60:4496–4503.
- Marchand CH, Salmeron C, Raad RB, Meniche X, Chami M, Masi M, 2012. Biochemical disclosure of the mycolate outer membrane of Corynebacterium glutamicum. J Bacteriol 194:587–597.
- Maresso AW, Garufi G, Schneewind O. 2008. Bacillus anthracis secretes proteins that mediate heme acquisition from hemoglobin. PLoS Pathog 4:e1000132.
- Maresso AW, Schneewind O. 2006. Iron acquisition and transport in Staphylococcus aureus. Biometals 19:193–203.
- Mariscotti JF, Garcia-Del Portillo F, Pucciarelli MG. 2009. The Listeria monocytogenes sortase-B recognizes varied amino acids at position 2 of the sorting motif. J Biol Chem 284:6140–6146.
- Matias VR, Al-Amoudi A, Dubochet J, Beveridge TJ. 2003. Cryo-transmission electron microscopy of frozen-hydrated sections of Escherichia coli and Pseudomonas aeruginosa. J Bacteriol 185:6112–6118.
- Matias VR, Beveridge TJ. 2007. Cryo-electron microscopy of cell division in Staphylococcus aureus reveals a mid-zone between nascent cross walls. Mol Microbiol 64:195–206.
- Mazmanian SK, Liu G, Jensen ER, Lenoy E, Schneewind O. 2000. Staphylococcus aureus sortase mutants defective in the display of surface proteins and in the pathogenesis of animal infections. Proc Natl Acad Sci USA 97:5510–5515.
- Mazmanian SK, Liu G, Ton-That H, Schneewind O. 1999. Staphylococcus aureus sortase, an enzyme that anchors surface proteins to the cell wall. Science 285:760–763.
- Mazmanian SK, Skaar EP, Gaspar AH, Humayun M, Gornicki P, Jelenska J, 2003. Passage of heme-iron across the envelope of Staphylococcus aureus.. Science 299:906–909.
- Mazmanian SK, Ton-That H, Su K, Schneewind O. 2002. An iron-regulated sortase anchors a class of surface protien during Staphylococcus aureus pathogenesis. Proc Natl Acad Sci USA 99:2293–2298.
- Mazurier J, Spik G. 1980. Comparative study of the iron-binding properties of human transferrins. I. Complete and sequential iron saturation and desaturation of the lactotransferrin. Biochim Biophys Acta 629:399–408.
- Mclaughlin H, Xiao Q, Rea RB, Pi H, Casey PG, Darby T, 2012. A putative P-type ATPase required for virulence and resistance to haem toxicity in Listeria monocytogenes. PLoS One 7:e30928.
- Meroueh SO, Bencze KZ, Hesek D, Lee M, Fisher JF, Stemmler TL, 2006. Three-dimensional structure of the bacterial cell wall peptidoglycan. Proc Natl Acad Sci USA 103:4404–4409.
- Morton DJ, Whitby PW, Jin H, Ren Z, Stull TL. 1999. Effect of multiple mutations in the hemoglobin- and hemoglobin-haptoglobin-binding proteins, HgpA, HgpB, and HgpC, of Haemophilus influenzae type b. Infect Immun 67:2729–2739.
- Navarre WW, Schneewind O. 1994. Proteolytic cleavage and cell wall anchoring at the LPXTG motif of surface proteins in gram-positive bacteria. Mol Microbiol 14:115–121.
- Navarre WW, Schneewind O. 1999. Surface proteins of gram-positive bacteria and mechanisms of their targeting to the cell wall envelope. Microbiol Mol Biol Rev 63:174–229.
- Negari S, Sulpher J, Pacello F, Ingrey K, Battistoni A, Lee BC. 2008. A role for Haemophilus ducreyi Cu, ZnSOD in resistance to heme toxicity. Biometals 21:249–258.
- Newton SM, Igo JD, Scott DC, Klebba PE. 1999. Effect of loop deletions on the binding and transport of ferric enterobactin by FepA. Mol Microbiol 32:1153–1165.
- Newton SM, Klebba PE, Raynaud C, Shao Y, Jiang X, Dubail I, 2005. The svpA-srtB locus of Listeria monocytogenes: Fur-mediated iron regulation and effect on virulence. Mol Microbiol 55:927–940.
- Newton SM, Trinh V, Pi H, Klebba PE. 2010. Direct measurements of the outer membrane stage of ferric enterobactin transport: Postuptake binding. J Biol Chem 285:17488–17497.
- Nhien NT, Huy NT, Naito M, Oida T, Uyen DT, Huang M, 2010. Neutralization of toxic haem by Porphyromonas gingivalis haemoglobin receptor. J Biochem 147:317–325.
- Niederweis M, Danilchanka O, Huff J, Hoffmann C, Engelhardt H. 2010. Mycobacterial outer membranes: In search of proteins. Trends Microbiol 18:109–116.
- Nikaido H, Vaara M. 1985. Molecular basis of bacterial outer membrane permeability. Microbiol Rev 49:1–32.
- Nobles CL, Maresso AW. 2011. The theft of host heme by Gram-positive pathogenic bacteria. Metallomics 3:788–796.
- Nygaard TK, Blouin GC, Liu M, Fukumura M, Olson JS, Fabian M, 2006. The mechanism of direct heme transfer from the streptococcal cell surface protein Shp to HtsA of the HtsABC transporter. J Biol Chem 281:20761–20771.
- Perez-Dorado I, Campillo NE, Monterroso B, Hesek D, Lee M, Paez JA, 2007. Elucidation of the molecular recognition of bacterial cell wall by modular pneumococcal phage endolysin CPL-1. J Biol Chem 282:24990–24999.
- Perez-Dorado I, Gonzalez A, Morales M, Sanles R, Striker W, Vollmer W, 2010. Insights into pneumococcal fratricide from the crystal structures of the modular killing factor LytC. Nat Struct Mol Biol 17:576–581.
- Pilpa RM, Robson SA, Villareal VA, Wong ML, Phillips M, Clubb RT. 2009. Functionally distinct NEAT (NEAr Transporter) domains within the Staphylococcus aureus IsdH/HarA protein extract heme from methemoglobin. J Biol Chem 284:1166–1176.
- Popowska M. 2004. Analysis of the peptidoglycan hydrolases of Listeria monocytogenes: Multiple enzymes with multiple functions. Pol J Microbiol 53(Suppl):29–34.
- Porter J, Arreguin S, Pierpont CG. 2010. Ferric iron complexes of dopamine and 5,6-dihydroxyindole with nta, edda, and edta as ancillary ligands. Inorg Chim Acta 363:2800–2803.
- Raymond KN, Carrano CJ. 1979. Coordination chemistry and microbial iron transport. Accts Chem Res 12:183–190.
- Ren Z, Jin H, Morton DJ, Stull TL. 1998. hgpB, a gene encoding a second Haemophilus influenzae hemoglobin- and hemoglobin-haptoglobin-binding protein. Infect Immun 66:4733–4741.
- Richardson AR, Stojiljkovic I. 1999. HmbR, a hemoglobin-binding outer membrane protein of Neisseria meningitidis, undergoes phase variation. J Bacteriol 181:2067–2074.
- Roche TE, Hiromasa Y. 2007. Pyruvate dehydrogenase kinase regulatory mechanisms and inhibition in treating diabetes, heart ischemia, and cancer. Cell Mol Life Sci 64:830–849.
- Roden JA, Wells DH, Chomel BB, Kasten RW, Koehler JE. 2012. Hemin binding protein C is found in outer membrane vesicles and protects Bartonella henselae against toxic concentrations of hemin. Infect Immun 80:929–942.
- Rouquette C, Bolla JM, Berche P. 1995. An iron-dependent mutant of Listeria monocytogenes of attenuated virulence. FEMS Microbiol Lett 133:77–83.
- Rozalska B, Lisiecki P, Sadowska B, Mikucki J, Rudnicka W. 1998. The virulence of Staphylococcus aureus isolates differing by siderophore production. Acta Microbiol Pol 47:185–194.
- Saier MH Jr. 2000. Families of proteins forming transmembrane channels. J Membr Biol 175:165–180.
- Saier MH Jr, Beatty JT, Goffeau A, Harley KT, Heijne WH, Huang SC, 1999. The major facilitator superfamily. J Mol Microbiol Biotechnol 1:257–279.
- Sanders JD, Cope LD, Hansen EJ. 1994. Identification of a locus involved in the utilization of iron by Haemophilus influenzae.. Infect Immun 62:4515–4525.
- Scheurwater EM, Burrows LL. 2011. Maintaining network security: How macromolecular structures cross the peptidoglycan layer. FEMS Microbiol Lett 318:1–9.
- Schiffler B, Barth E, Daffe M, Benz R. 2007. Corynebacterium diphtheriae: Identification and characterization of a channel-forming protein in the cell wall. J Bacteriol 189:7709–7719.
- Schneewind O, Mihaylova-Petkov D, Model P. 1993. Cell wall sorting signals in surface proteins of Gram-positive bacteria. EMBO J 12:4803–4811.
- Schryvers AB, Stojiljkovic I. 1999. Iron acquisition systems in the pathogenic Neisseria. Mol Microbiol 32:1117–1123.
- Schwarzenbach G, Schwarzenbach K. 1963. Hydroxamatkomplexe I. Die stabilitat der Eisen (III)-komplexe einfacher hydroxamsauren und des ferroxamins B. Helv Chim Acta 46:1390–1400.
- Schwyn B, Neilands JB. 1987. Universal chemical assay for the detection and determination of siderophores. Anal Biochem 160:47–56.
- Scott DC, Cao Z, Qi Z, Bauler M, Igo JD, Newton SM, 2001. Exchangeability of N termini in the ligand-gated porins of Escherichia coli.. J Biol Chem 276:13025–13033.
- Sebulsky MT, Hohnstein D, Hunter MD, Heinrichs DE. 2000. Identification and characterization of a membrane permease involved in iron-hydroxamate transport in Staphylococcus aureus.. J Bacteriol 182:4394–4400.
- Sharp KH, Schneider S, Cockayne A, Paoli M. 2007. Crystal structure of the heme-IsdC complex, the central conduit of the Isd iron/heme uptake system in Staphylococcus aureus.. J Biol Chem 282:10625–10631.
- Spirig T, Weiner EM, Clubb RT. 2011. Sortase enzymes in Gram-positive bacteria. Mol Microbiol 82:1044–1059.
- Sprencel C, Cao Z, Qi Z, Scott DC, Montague MA, Ivanoff N, 2000. Binding of ferric enterobactin by the Escherichia coli periplasmic protein fepB. J Bacteriol 182:5359–5364.
- Stauff DL, Bagaley D, Torres VJ, Joyce R, Anderson KL, Kuechenmeister L, 2008. Staphylococcus aureus HrtA is an ATPase required for protection against heme toxicity and prevention of a transcriptional heme stress response. J Bacteriol 190:3588–3596.
- Stauff DL, Skaar EP. 2009. The heme sensor system of Staphylococcus aureus.. Contrib Microbiol 16:120–135.
- Stevens MK, Porcella S, Klesney-Tait J, Lumbley S, Thomas SE, Norgard MV, 1996. A hemoglobin-binding outer membrane protein is involved in virulence expression by Haemophilus ducreyi in an animal model. Infect Immun 64:1724–1735.
- Stojiljkovic I, Hwa V, Larson J, Lin L, So M, Nassif X. 1997. Cloning and characterization of the Neisseria meningitidis rfaC gene encoding alpha-1,5 heptosyltransferase I. FEMS Microbiol Lett 151:41–49.
- Tai SS, Lee CJ, Winter RE. 1993. Hemin utilization is related to virulence of Streptococcus pneumoniae.. Infect Immun 61:5401–5405.
- Thomas KL, Leduc I, Olsen B, Thomas CE, Cameron DW, Elkins C. 2001. Cloning, overexpression, purification, and immunobiology of an 85-kilodalton outer membrane protein from Haemophilus ducreyi.. Infect Immun 69:4438–4446.
- Tidmarsh GF, Klebba PE, Rosenberg LT. 1983. Rapid release of iron from ferritin by siderophores. J Inorg Biochem 18:161–168.
- Tomasz A. 1979. From penicillin-binding proteins to the lysis and death of bacteria: A 1979 view. Rev Infect Dis 1:434–467.
- Ton-That H, Liu G, Mazmanian SK, Faull KF, Schneewind O. 1999. Purification and characterization of sortase, the transpeptidase that cleaves surface proteins of Staphylococcus aureus at the LPXTG motif. Proc Natl Acad Sci USA 96:12424–12429.
- Ton-That H, Mazmanian SK, Faull KF, Schneewind O. 2000. Anchoring of surface proteins to the cell wall of Staphylococcus aureus. Sortase catalyzed in vitro transpeptidation reaction using LPXTG peptide and NH(2)-Gly(3) substrates. J Biol Chem 275:9876–9881.
- Torres VJ, Pishchany G, Humayun M, Schneewind O, Skaar EP. 2006. Staphylococcus aureus IsdB is a hemoglobin receptor required for heme iron utilization. J Bacteriol 188:8421–8429.
- Touhami A, Jericho MH, Beveridge TJ. 2004. Atomic force microscopy of cell growth and division in Staphylococcus aureus.. J Bacteriol 186:3286–3295.
- Trias J, Jarlier V, Benz R. 1992. Porins in the cell wall of mycobacteria. Science 258:1479–1481.
- Tsolis RM, Baumler AJ, Heffron F, Stojiljkovic I. 1996. Contribution of TonB- and Feo-mediated iron uptake to growth of Salmonella typhimurium in the mouse. Infect Immun 64:4549–4556.
- Vollmer W, Blanot D, De Pedro MA. 2008. Peptidoglycan structure and architecture. FEMS Microbiol Rev 32:149–167.
- Vollmer W, Seligman SJ. 2010. Architecture of peptidoglycan: More data and more models. Trends Microbiol 18:59–66.
- Ward CG, Bullen JJ, Rogers HJ. 1996. Iron and infection: New developments and their implications. J Trauma 41:356–364.
- Wayne R, Neilands JB. 1975. Evidence for common binding sites for ferrichrome compounds and bacteriophage phi 80 in the cell envelope of Escherichia coli.. J Bacteriol 121:497–503.
- Weiss WJ, Lenoy E, Murphy T, Tardio L, Burgio P, Projan SJ, 2004. Effect of srtA and srtB gene expression on the virulence of Staphylococcus aureus in animal models of infection. The structure of sortase B, a cysteine transpeptidase that tethers surface protein to the Staphylococcus aureus cell wall IsdG and IsdI, heme-degrading enzymes in the cytoplasm of Staphylococcus aureus. J Antimicrob Chemother 4:4.
- Xiao Q, Jiang X, Moore KJ, Shao Y, Pi H, Dubail I, 2011. Sortase independent and dependent systems for acquisition of haem and haemoglobin in Listeria monocytogenes. Mol Microbiol 80:1581–1597.
- Yem DW, Wu HC. 1978. Physiological characterization of an Escherichia coli mutant altered in the structure of murein lipoprotein. J Bacteriol 133:1419–1426.
- Young KD. 2006. Too many strictures on structure. Trends Microbiol 14:155–156.
- Zhang Z, Aboulwafa M, Smith MH, Saier MH Jr. 2003. The ascorbate transporter of Escherichia coli.. J Bacteriol 185:2243–2250.
- Zhu H, Liu M, Lei B. 2008a. The. surface protein Shr of Streptococcus pyogenes binds heme and transfers it to the streptococcal heme-binding protein Shp. BMC Microbiol 8:15.
- Zhu H, Xie G, Liu M, Olson JS, Fabian M, Dooley DM, 2008b. Pathway for heme uptake from human methemoglobin by the iron-regulated surface determinants system of Staphylococcus aureus.. J Biol Chem 283:18450–18460.
- Zhu W, Hunt DJ, Richardson AR, Stojiljkovic I. 2000. Use of heme compounds as iron sources by pathogenic neisseriae requires the product of the hemO gene. J Bacteriol 182:439–447.
- Zuber B, Chami M, Houssin C, Dubochet J, Griffiths G, Daffe M. 2008. Direct visualization of the outer membrane of mycobacteria and corynebacteria in their native state. J Bacteriol 190:5672–5680.