Abstract
The mitochondrial ADP/ATP carrier imports ADP from the cytosol into the mitochondrial matrix for its conversion to ATP by ATP synthase and exports ATP out of the mitochondrion to replenish the eukaryotic cell with chemical energy. Here the substrate specificity of the human mitochondrial ADP/ATP carrier AAC1 was determined by two different approaches. In the first the protein was functionally expressed in Escherichia coli membranes as a fusion protein with maltose binding protein and the effect of excess of unlabeled compounds on the uptake of [32P]-ATP was measured. In the second approach the protein was expressed in the cytoplasmic membrane of Lactococcus lactis. The uptake of [14C]-ADP in whole cells was measured in the presence of excess of unlabeled compounds and in fused membrane vesicles loaded with unlabeled compounds to demonstrate their transport. A large number of nucleotides were tested, but only ADP and ATP are suitable substrates for human AAC1, demonstrating a very narrow specificity. Next we tried to understand the molecular basis of this specificity by carrying out molecular-dynamics simulations with selected nucleotides, which were placed at the entrance of the central cavity. The binding of the phosphate groups of guanine and adenine nucleotides is similar, yet there is a low probability for the base moiety to be bound, likely to be rooted in the greater polarity of guanine compared to adenine. AMP is unlikely to engage fully with all contact points of the substrate binding site, suggesting that it cannot trigger translocation.
Introduction
Mitochondrial carriers form a family of transporters that are embedded in the inner mitochondrial membrane. They are responsible for the traffic of a variety of metabolites and small molecules between the cytoplasm and the mitochondrial matrix (Kunji Citation2004, Palmieri Citation2004). The substrate specificity of mitochondrial carriers is quite narrow, as only chemically and structurally related compounds are accepted as substrates for import or export. The mitochondrial ADP/ATP carrier (AAC) transports newly synthesized ATP out of the mitochondrion and in return imports ADP. Due to its abundance in heart mitochondria, it is the best-characterized mitochondrial carrier (Klingenberg Citation2008) and the only one for which a high-resolution structure is available (Pebay-Peyroula et al. Citation2003). Compared to other transporters, mitochondrial carriers are relatively small, comprising only six transmembrane helices connected through very short loops, delineating a central pathway through which the passage of the substrates occurs. The known AAC1 structure resembles the conformation that is open toward the intermembrane space able to bind ADP (Nury et al. Citation2006).
The directionality of adenine nucleotide transport is imposed by the concentration gradients of the substrates and by the electrostatic potential of the inner mitochondrial membrane as the ADP/ATP exchange is electrogenic. In vitro, it was shown that either ADP or ATP can be transported in both ways (Brustovetsky et al. Citation1997, Citation1996). In humans there are four different isoforms, which have different expression patterns (Dolce et al. Citation2005). The substrate specificity of the rat mitochondrial ADP/ATP carrier is largely limited to ADP and ATP (Pfaff and Klingenberg Citation1968). Surprisingly, the carrier does not transport the related pyrimidine nucleotides GDP and GTP. An unresolved issue is whether AMP can be transported or whether it can interfere with ADP and ATP binding. Residues potentially involved in substrate binding were identified by site-directed mutagenesis and photolabeling (Dalbon et al. Citation1988, Heidkamper et al. Citation1996, Muller et al. Citation1997, Dianoux et al. Citation2000), but the extent of the substrate binding site was defined only after the structure became available by sequence analyses (Robinson and Kunji Citation2006, Robinson et al. Citation2008) and molecular-dynamics simulations (Dehez et al. Citation2008, Wang and Tajkhorshid Citation2008). The major contact points of the binding site that interact with the nucleotides consist of positively charged residues that bind the phosphate moieties (contact point I and III) and a hydrophobic pocket that binds the adenine moiety (contact point II) () (Robinson and Kunji Citation2006, Dehez et al. Citation2008, Robinson et al. Citation2008, Wang and Tajkhorshid Citation2008). The definition of the substrate binding site is not sufficient to explain the narrow substrate selectivity, but it is a crucial step to a deeper understanding of the transport mechanism at the molecular level.
Figure 1. Molecular-dynamics simulations assays. (Left) Typical configuration of the bovine-heart AAC embedded in a fully hydrated POPC bilayer (the chloride counter-ions are depicted as green spheres and water molecules are not represented for clarity) with a nucleotide bound at the bottom of the internal cavity of the carrier. (Right) Starting positions of the nucleotides for the 10 binding assays. Cyan and dark-blue surfaces correspond respectively to nucleotides found in the vicinity of the upper and the lower patches of basic residues. The three major contact points of the binding site that interact with the nucleotides are indicated (I: K22, R79; II: G182, I183; III: R279) (Robinson and Kunji Citation2006).
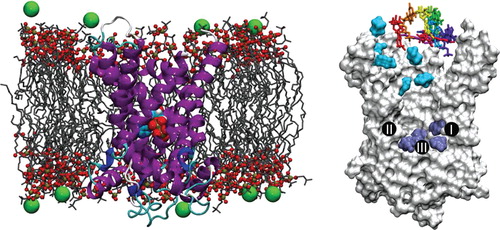
Although the transport selectivity has been explored extensively in mitochondrial membranes (i.e., Duee and Vignais Citation1968, Pfaff and Klingenberg Citation1968), the substrate specificity of AAC has not been studied in isolated systems nor has it been defined at the atomic level. Here we have used two different expression systems to analyze the transport of nucleotides by the major human isoform AAC1 (hAAC1). In parallel, the entrance and binding of these nucleotides into the cavity of AAC1 were followed by molecular-dynamics simulations. The transport assays clearly confirm the selectivity for adenine nucleotides comprising 2 or 3 phosphates. In the light of molecular-dynamics simulations performed herein, the molecular basis that determines the selectivity of the carrier in its conformation opened toward the intermembrane space (IMS) can be established. Interestingly, the numerical experiments described in this contribution suggest that both the phosphate and the base moiety ought to be recognized sequentially to ensure binding. Even though the phosphate binding is similar for GDP (GTP) and ADP (ATP), the base recognition is probably less efficient for guanine compared to adenine nucleotides. Our findings confirm experimentally the molecular basis of nucleotide specificity of the ADP/ATP carrier and shed light on the mechanism of selective binding.
Material and methods
Uptake of radioactively labeled ATP by intact Escherichia coli cells expressing hAAC1
The hAAC1 gene was cloned as a fusion to the periplamsic MBP (MBP-hAAC1) as described previously (Ravaud et al. Citation2012). The recombinant protein was expressed in Escherichia coli strain C43 (DE3) (Miroux and Walker Citation1996). Cells were grown at 37°C in LB (lysogeny broth) medium containing ampicillin (100 mg.l-1) to an A600 of 0.6. Protein expression was induced using 0.1 mM of isopropyl β-D-1-thiogalactopyranoside (IPTG) and took place overnight at 20°C. Cells were harvested by centrifugation for 15 min at 3,000 g and 4°C and washed once in 50 mM potassium phosphate buffer pH 7. Cells were sedimented again (3,000 g, 15 min, 4°C) and the final pellet was resuspended in 50 mM potassium phosphate buffer pH 7 to a cell density of 0.1 g.ml-1 and kept on ice.
The uptake assays were initiated by the addition of 10 μM [α-32P]-labeled ATP (3,000 mCi/mmol; Perkin Elmer) in 30 μl of the IPTG-induced E. coli cells. The incubation was performed at 25°C and was terminated after 30 min by the addition of 1 ml ice-cold potassium phosphate buffer followed by rapid filtration through a 0.45 μm filter (Millipore, France) under vacuum. The filters were washed three times with 1 ml ice-cold potassium phosphate buffer and the radioactivity retained on the filters was quantified in 3.5 ml of water in a Multi-Purpose Scintillation Counter (Beckman Coulter, Fullerton, CA, USA). Competition experiments were carried out with ATP, ADP, AMP-PNP, GDP, and GTP at a final concentration of 100 μM. The nucleotides were added 5 min before the uptake experiment was started and after addition of [α-32P]-labeled ATP, the transport rates were determined at 30 min.
In all experiments, C43 (DE3) E. coli cells harboring the corresponding empty vector (pET-20b vector containing only the periplasmic MBP gene) were used as a control.
Uptake of radioactively labeled ADP by intact Lactococcus lactis cells expressing hAAC1
Lactococcus lactis strain NZ9000 was transformed with vector PNZ8048 containing the gene for the expression of hAAC1, essentially as described (Kunji et al. Citation2003). Pre-cultures of L. lactis were set up overnight in M17 media to grow at 30°C with no aeration to an optical density of ∼ 2.5 (A600nm). The growth in M17 media was initiated at an A600nm of ∼ 0.1 from the pre-culture and grown at 30°C until an A600nm of 0.5–0.7 was reached. Induction of protein expression was performed by addition of filtered M17 medium containing nisin A excreted by strain NZ9700 at a dilution of 1:10000, after which growth was continued for a further 3 h.
Cells were collected at 6,000 g for 10 min at 4°C and washed once in PIPES buffer (10 mM piperazine-1,4-bis-2-ethanesulfonic acid, 50 mM NaCl, pH 7.0). The final pellet was resuspended to A600nm of 20 and stored on ice. After 30 min pre-incubation at room temperature the transport assays performed at 30°C were started by adding an equal volume of [14C]-ADP radiolabel (2.22 GBq mmol-1, Perkin Elmer) to the cell suspension. When inhibitor studies were performed, the inhibitor was added to the mixture 5 min before the uptake experiment commenced. Samples of 15% of the total volume were taken at appropriate time-points, quenched with ice-cold PIPES buffer and filtered on a vacuum manifold through cellulose nitrate membranes (0.45 μm pore, Whatman). The filters were washed with 3 ml of cold buffer and dissolved in 3 ml of liquid scintillant (Ultima Gold AB, Perkin Elmer), which were counted in a TriCarb liquid scintillant counter (Perkin Elmer).
Uptake of radioactively labeled ADP by fused membrane vesicles of Lactococcus lactis expressing hAAC1
Cells expressing hAAC1 were harvested at 6000 g for 10 min at 4°C, washed in PIPES buffer and resuspended in 1/40 of the original volume. Cell lysis was performed by mechanical disruption with one pass at 33,000 pa in a cell disruptor (Constant Cell Disruption Systems, Daventry, UK). Whole cells were removed by a low speed spin at 10,800 g for 10 min at 4°C. Membranes were harvested by two ultracentrifugation steps at 138,000 g for 40 min at 4°C, washing with PIPES buffer in between. Pellets were resuspended in PIPES buffer to a total protein concentration of 5 mg.ml-1 and stored in liquid nitrogen.
E. coli polar lipid extract and egg yolk phosphatidylcholine (both at 20 mg.ml-1 in chloroform, Avanti Polar lipids) were mixed in a 3:1 weight ratio and dried by agitation under nitrogen. The lipids were resuspended in a homogenizer in cold PIPES buffer and frozen in liquid nitrogen. Isolated lactococcal membranes were fused with liposomes by freeze-thawing (Driessen et al. Citation1985). First, membranes (1 mg total protein concentration) were mixed with liposomes (5 mg) in PIPES buffer and a substrate for loading of the fused membranes (5 mM final concentration from 10× stock in PIPES buffer, pH 7). The membrane mixture was frozen in liquid nitrogen and thawed to room temperature seven times before storage in liquid nitrogen to create multilamellar fused membranes. The effect of bovine heart cardiolipin (Avanti Polar Lipids) on transport was investigated by adding different amounts of cardiolipin to the mixed vesicles and liposomes prior to freeze-thawing. Prior to performing transport assays, fused membrane vesicles were extruded 11 times through a polycarbonate nucleopore track etch membrane (1 μm, Whatman) to form single lamellar vesicles of defined size. The extruded membranes were concentrated five-fold by ultracentrifugation at 300,000 g for 30 min at 4°C and resuspended in buffer containing 5 mM substrate. External substrate was removed by passing the fused membranes through a Sephadex G-75 gel filtration column (3.5 ml bed volume, GE Healthcare), eluting in a 1 ml volume. After 30 min pre-incubation at room temperature transport assays were started by diluting the loaded vesicles four-fold into buffer containing [14C]-ADP (2.22 GBq mmol-1, Perkin Elmer) at the stated final concentrations. Uptakes, which were performed at 30°C, were stopped by quenching with ice-cold PIPES buffer and filtration on a vacuum manifold through cellulose nitrate membranes (0.45 μm pore, Whatman). The filters were washed with 3 ml of cold buffer and then dissolved in 3 ml of scintillation liquid (Ultima Gold AB, Perkin Elmer). The amount of accumulated radio-label was determined in a TriCarb liquid scintillation counter (Perkin Elmer).
Molecular-dynamics simulations
The spontaneous association of six nucleotide analogues (AMP, ADP, ATP, GMP, GDP and GTP) was investigated by means of molecular-dynamics (MD) simulations employing a methodology described previously (Dehez et al. Citation2008). The initial molecular assay consisted of the bovine heart AAC in its conformation open toward the cytosol as revealed by X-ray crystallography (PDBID:1OKC) (Pebay-Peyroula et al. Citation2003). After removal of the inhibitor CATR bound to the crystal structure, AAC was inserted in a thermalized, fully hydrated palmitoyloleylphosphatidyl-choline (POPC) bilayer consisting of 228 lipid unit and approximately 10,000 water molecules (). All molecular assemblies were built and analyzed with VMD (Humphrey et al. Citation1996). The net excess charge of AAC was counter-balanced by means of 17 chloride counter ions. This corresponds to a chloride concentration of about 0.1 M. The complete molecular assays involving the carrier in its hydrated membrane environment together with one of the aforementioned nucleotide were described by the all-atom CHARMM27 (Mackerell et al. Citation1998, Feller and Mackerell Citation2000) force field for the protein, the nucleotides, water and ions. A modified united-atom version of the force field was utilized for the lipids (Henin et al. Citation2008). CMAP corrections (Mackerell et al. Citation2004) were introduced for the protein. All MD simulations were carried out using the NAMD (Phillips et al. Citation2005) package, in the isobaric-isothermal ensemble. The temperature and the pressure were kept constant at 300 K and 1 atm using, respectively, Langevin dynamics and the Langevin piston method. The particle mesh Ewald (PME) algorithm was employed to account for long-range electrostatic interactions (Darden et al. Citation1993). The equations of motion were integrated by means of the resp-A multiple-time step algorithm (Tuckerman et al. Citation1992) with a time step of 2 and 4 fs for short- and long-range interactions, respectively. Covalent bonds involving hydrogen atoms were constrained to their equilibrium length by means of the Rattle algorithm (Andersen Citation1983). The molecular assemblies were first equilibrated at 300 K under 1 atm for approximately 100 ns, thereby providing the initial configuration for the subsequent binding assays. Each nucleotide was successively inserted at the mouth of the carrier with 10 randomly chosen initial orientations (). Following a short equilibration of the water molecules around the substrate, a series of unbiased MD trajectories up to 100-ns long was generated. Binding of the substrates was characterized by considering how long-lived – specifically over time scales exceeding 15 ns, and steady is the anchoring of the phosphate and the nucleoside moieties at the bottom of the cavity.
Results and discussion
Substrate specificity of the human ADP/ATP carrier
The substrate specificity of the human ADP/ATP carrier hAAC1 was investigated by expressing the carrier in two different bacterial hosts. In the first approach hAAC1 is expressed in E. coli membranes in a functional form by fusing the periplasmic maltose binding protein to the N-terminus of the carrier. It has been shown previously that its main transport properties are retained in this heterologous system (Ravaud et al. Citation2012). [α-32P]ATP uptake experiments revealed an apparent Km for ATP of 23.7 ± 5 μm and a V max of 14.6 ± 0.6 nmol/min/mg of protein consistent with previously published data (De Marcos Lousa et al. Citation2002). The measured transport activity could also be completely inhibited by the addition of the specific inhibitors carboxy-atractyloside (CATR) and bongkrekic acid (BA). To study the substrate specificity, transport of [α-32P]ATP was measured in the presence and absence of 100 μM of competing nucleotides to test whether these compounds can compete with the radio-labeled substrate for binding or can inhibit the transport activity. ATP import was reduced by around 80% by competition with excess ATP or ADP and to a much lower extent by competition with other nucleotides (). ADP exhibited the strongest inhibition on ATP import. In the presence of AMP, the uptake is reduced by about 40%, while in the presence of GTP, GDP and GMP the uptake level remains essentially unaffected.
Figure 2. Effect of the nature of the nucleotide on [α-32P]ATP uptake into E. coli cells expressing hAAC1. IPTG-induced E. coli cells harboring plasmid MBP-hAAC1 were incubated in potassium phosphate buffer containing 10 μM labeled ATP. The uptake assay was performed in the absence or in the presence of 100 μM of non-labeled nucleotides and carried out for 30 min. The uptake was terminated by the addition of cold buffer and the samples were filtered. The rate of transport in the absence of additives (No add.) was set to 100%. The control nucleotide uptake (empty vector) was subtracted. Data are the mean of three independent experiments. The average values with standard deviation are shown.
![Figure 2. Effect of the nature of the nucleotide on [α-32P]ATP uptake into E. coli cells expressing hAAC1. IPTG-induced E. coli cells harboring plasmid MBP-hAAC1 were incubated in potassium phosphate buffer containing 10 μM labeled ATP. The uptake assay was performed in the absence or in the presence of 100 μM of non-labeled nucleotides and carried out for 30 min. The uptake was terminated by the addition of cold buffer and the samples were filtered. The rate of transport in the absence of additives (No add.) was set to 100%. The control nucleotide uptake (empty vector) was subtracted. Data are the mean of three independent experiments. The average values with standard deviation are shown.](/cms/asset/0975a6bb-f473-4f65-be9d-e88d19d3375e/imbc_a_745175_f0002_b.jpg)
In the second approach hAAC1 was expressed in the cytoplasmic membrane of Lactococcus lactis (). Uptake into whole cells of L. lactis expressing AAC1 was observed by the exchange of intracellular adenine nucleotides for radio-labeled ADP, but not in the control strain (). Complete inhibition of uptake was achieved by the addition of 10 μM CATR or 2.5 μM BA in agreement with published data on human AAC isoforms 1, 2 and 3 expressed in yeast mitochondria (De Marcos Lousa et al. Citation2002), AAC4 expressed in E. coli and refolded (Dolce et al. Citation2005) and AAC of rat liver mitochondria (Pfaff and Klingenberg Citation1968). To see if the expression levels and uptake rates could be improved, the N-terminus was truncated, as has been done for other members of the mitochondrial carrier family (Monne et al. Citation2005). The N-terminus of the hAAC1 was truncated (▵1–3 human AAC1) to a length identical to the optimal truncation for the yeast Aac2p ▵2–19, but no significant improvement in the uptake rate was observed (data not shown). Another factor that was investigated was the requirement of cardiolipin, which is known to improve the activity of bovine AAC1 reconstituted in proteoliposomes (Kramer and Klingenberg Citation1980). Lactococcal membranes are suitable for the expression of mitochondrial carriers, as they naturally contain a large amount of cardiolipin (32% of total lipid), but the length and saturation of the alkyl chains may differ from mitochondrial cardiolipin. Different additions of bovine cardiolipin to the membrane fusions were tried, and the optimal one corresponded approximately to 6% of total lipid and led to a 2-fold increase in transport rate (data not shown).
Figure 3. Expression and transport of ADP by the human AAC1 expressed in Lactococcus lactis. (A) (A) Coomassie brilliant blue-stained SDS- PAGE gel of the cytoplasmic membrane of the control and hAAC1-expressing strain. The arrowhead indicates the expressed hAAC1. (B) Radio-labeled ADP uptake by whole Lactococcus cells in the absence of inhibitors (circles) or in the presence of the specific inhibitors bongkrekic acid (squares) or carboxy-atractyloside (triangles). The results are presented as the average and standard deviation from three independent experiments.
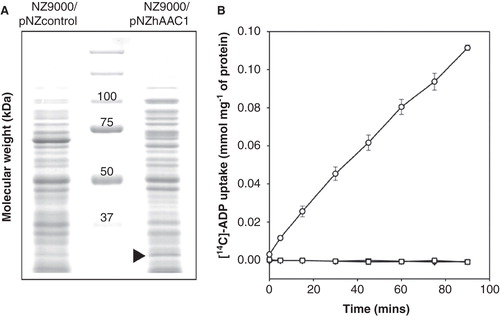
The substrate specificity of hAAC1 was determined by two routes. The first strategy was similar to the one used with E. coli cells. Radio-labeled ADP uptake in whole cells was monitored in the presence of a 1000-fold excess of a non-labeled compound (). High competition for substrate binding or transport inhibition was observed with ADP and ATP, as observed for hAAC1 expressed in E. coli cells. Furthermore, an 85% and 80% reduction in the transport rate was observed with an excess of AMP or ADP-glucose, respectively. NADH reduced the transport rate by 60%, unlike NAD+, which exhibited no effect. Furthermore, the purine nucleotides, (deoxy-thymidine triphosphate, and deoxy-uridine triphosphate, reduced the rate to 45%, but the corresponding di- and monophosphate nucleotides did not. The competition with the oxy-form of uridine triphosphate only led to a reduction of 30%, similar to the guanosine tri- and di-phosphate and FAD, although the latter effect may be due to interference with liquid scintillation counting because of the yellow colour. The purine cytidine-triphosphate, but not the diphosphates or the deoxy form, reduced the uptake by 40%.
Figure 4. Substrate specificity of the human AAC1 protein. (A) Initial uptake rate of radio-labeled ADP in the presence or absence of a 1000-fold higher concentration of the indicated non-radio-labeled compounds. Uptake was started by the addition of 1.25 μM [14C]-ADP to Lactococcus cells at final A600nm of 8 and after 1 h, the uptake was quenched by adding cold buffer and the sample was filtered. (B) Initial uptake rate of radio-labeled ADP into fused membrane vesicles pre-loaded with 5 mM of the indicated compound. Uptake will only occur if the internal compound can be transported out of the vesicle. Substrate exchange was started by the addition of 1.34 μM [14C]-ADP and the initial rate was determined by measuring the accumulated radio-labeled substrate after 10 min. Experiments were carried out in triplicate and the average with standard deviation is shown.
![Figure 4. Substrate specificity of the human AAC1 protein. (A) Initial uptake rate of radio-labeled ADP in the presence or absence of a 1000-fold higher concentration of the indicated non-radio-labeled compounds. Uptake was started by the addition of 1.25 μM [14C]-ADP to Lactococcus cells at final A600nm of 8 and after 1 h, the uptake was quenched by adding cold buffer and the sample was filtered. (B) Initial uptake rate of radio-labeled ADP into fused membrane vesicles pre-loaded with 5 mM of the indicated compound. Uptake will only occur if the internal compound can be transported out of the vesicle. Substrate exchange was started by the addition of 1.34 μM [14C]-ADP and the initial rate was determined by measuring the accumulated radio-labeled substrate after 10 min. Experiments were carried out in triplicate and the average with standard deviation is shown.](/cms/asset/a13c082a-6d63-47c3-818b-4185813fd0ab/imbc_a_745175_f0004_b.jpg)
To test whether these compounds are actually transported by the carrier, unlabeled compound was loaded into the fused membrane vesicles and the uptake of radio-labeled ADP was monitored (). Only when the compound is exported from the fused membrane vesicles can uptake of the label occur. Exchange was observed only in the case of ADP, ATP and potentially dADP and dATP in agreement with literature. No transport was observed for the other competitive inhibitors, such as NADH, dUTP, or AMP.
Our results are consistent with previously reported data indicating the strict specificity of ADP/ATP carriers for ADP and ATP (Pfaff and Klingenberg Citation1968, De Marcos Lousa et al. Citation2002, Dolce et al. Citation2005).
Molecular-dynamics simulations
MD simulations have demonstrated the ability of the 1OKC structure to recognize and bind spontaneously ADP (Dehez et al. Citation2008, Wang and Tajkhorshid Citation2008). Free-energy calculations have further revealed two binding motifs differing solely by the nucleoside position, the phosphate moiety remaining in close contact with the lower patch of basic residues. Anchoring of the substrate deep inside the carrier cavity, therefore, appears to constitute an early event of the overall ADP/ATP exchange mechanism. The spatial organization of two specific patches of basic residues within the cavity confers to the protein a very specific electrostatic signature consisting of a funnel responsible for conveying ADP down the cavity, towards the bottom of the carrier. However robust and fast, the binding process is very sensitive to any alteration of this signature, possibly induced by either single-point mutations of charged residues, or electrostatic screening in response to a high concentration of small anions such as chloride (Krammer et al. Citation2009, Ravaud et al. Citation2012). At the core of the exchange process lies the selectivity of AAC towards ADP and ATP only – neither AMP nor any other nucleotide analogue, e.g., a guanosine phosphate, is transported. In this work, all-atom numerical simulations were employed to gain new insight into the possible role of the 1OKC conformation in the selectivity of the mitochondrial carrier. A series of 10 association experiments were carried out for each of the following nucleotides, AMP, ADP, ATP, GMP, GDP and GTP. In all trajectories, spontaneous binding of the substrate to either the top or the bottom basic patch was monitored. A statistical analysis of the different association assays is reported in and shows that all substrates are recognized by AAC in its conformation open toward the IMS; for all of them at least one stable binding state was observed in agreement with the systematic competition of theses substrates for ADP binding observed in L. lactis expressing AAC1 (). At the bottom of the cavity, the binding motif of the phosphate moiety constitutes the common denominator for all nucleotides involving residues K22, R79, R235 and R279 (). The situation is somewhat different for those nucleosides that are primarily found at two different positions, B1 and B2 (), regardless of the nucleotides. In line with our previous findings (Dehez et al. Citation2008), B1, which overlaps partially with the binding site of CATR revealed by the crystal structure (Pebay-Peyroula et al. Citation2003), involves residues Y186, R187, S227 and G224, whereas in B2, the nucleosides adopt a conformation wherein the base moiety interacts with A284 and S21. Sliding and anchoring of the phosphate group appears to be a fast and robust event of the overall association process (occurring on a time scale spanning approximately 10 ns) independent of the nucleoside and the number of terminal phosphate groups. In sharp contrast, reorientation of the sugar and base moieties occurs on a longer time scale and appears to represent the rate-limiting step of the complete binding event. Interestingly enough, stable association of the nucleoside at the bottom of the cavity seems to be easier in the case of ADP and ATP. For the latter two substrates, anchoring of the phosphate moiety at the bottom of the cavity has led systematically to the binding of the nucleoside group to the carrier. It is noteworthy that the polarity of guanine is about three times higher than adenine (Jasien and Fitzgerald Citation1990) resulting in a weaker interaction with the hydrophobic pocket that binds the adenine moiety (contact point II). Furthermore, it might be argued that the lesser polarity of adenine results in a weaker interaction with the local, oriented electric field created by the inner cavity of the transporter and, hence, to a greater orientational entropy, compared to guanine.
Table I. Statistical analysis of the association assays. Binding is considered successful when the nucleotide is found inside the cavity, with the phosphate and the nucleoside moieties steadily bound to the bottom of the carrier over time scales in excess of 15 ns.
Figure 5. Most populated binding motifs (B1 and B2) revealed by the association-assays. (Left) Global view of the main binding positions of the nucleotides at the bottom of the cavity of AAC near its 1OKC conformation. (Top right) Detailed view of B1 and B2 binding motifs. (Bottom right) Overlap between CATR and B1 binding motifs.
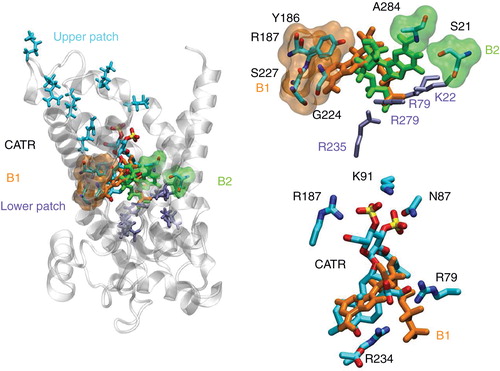
Conclusion
In this contribution, the selectivity of the mitochondrial human AAC has been explored employing two expression assays for the carrier, namely E. coli and L. lactis. Experiments of competitive inhibition reveal a clear preference for the ADP (dADP) and ATP (dATP) tandem, albeit do not rule out the possible interaction with alternate substrates, e.g., AMP, GMP, GDP and GTP, as soon as an appreciable ratio between the concentrations in substrate and inhibitor is involved. These experiments, however, provide only a convoluted picture of the binding specificity and transport selectivity of the latter. Uptake experiments of radio-labeled ADP into fused membrane vesicles preloaded with inhibitors, nevertheless, indicate that, inasmuch as transport is concerned, selectivity only favors ADP and ATP. At the molecular level, numerical simulations indicate that all the nucleotides considered in the computational section of the present study bind to the carrier in a nonspecific fashion through their phosphate moiety, which is consistent with the experiments of competitive inhibition at high concentration ratio. Binding of the base to the carrier appears to be more specific and has been observed systematically for both ADP and ATP once their phosphate group is anchored steadily within the protein. These results suggest that the chemical signature of the nucleobase and the topology of the nucleotide, namely the distance between the base and the terminal phosphate moiety, constitute two stringent criteria likely to modulate the binding of nucleotide analogues to AAC in a conformation akin to the CATR inhibited-state. These observations provide the background for rationalizing the substrate specificity of the carrier, but need to be further complemented to unveil the molecular basis of the transport selectivity of AAC towards ADP and ATP only. In this context, one of the remaining, most challenging tasks consists in isolating alternative AAC conformations and transient binding states that accompany substrate translocation.
Acknowledgements
The collaboration was funded by the European Community's Seventh Framework Programme FP7/2007–2013 under grant agreement no. HEALTH-F4-2007-201924, EDICT Consortium. The work was also supported by the Agence Nationale de la Recherche (ANR Trans-MIT and MIT-2M projects). We thank P. Machillot for his technical help in the uptake experiments, N. Cherradi and O. Filhol-Cochet for access to the radioactivity room (CEA/iRTSV). The Grand Equipement National de Calcul Informatique and Centre Informatique National de l’Enseignement Supérieur are gratefully acknowledged for provision of computer time.
Declaration of interest: The authors report no conflicts of interest. The authors alone are responsible for the content and writing of the paper.
References
- Andersen HC. 1983. A “velocity” version of the Shake algorithm for molecular dynamics calculations. J Comput Phys 52:24–34.
- Brustovetsky N, Bamberg E, Gropp T, Klingenberg M. 1997. Biochemical and physical parameters of the electrical currents measured with the ADP/ATP carrier by photolysis of caged ADP and ATP. Biochemistry 36:13865–13872.
- Brustovetsky N, Becker A, Klingenberg M, Bamberg E. 1996. Electrical currents associated with nucleotide transport by the reconstituted mitochondrial ADP/ATP carrier. Proc Natl Acad Sci USA 93:664–668.
- Dalbon P, Brandolin G, Boulay F, Hoppe J, Vignais PV. 1988. Mapping of the nucleotide-binding sites in the ADP/ATP carrier of beef heart mitochondria by photolabeling with 2-azido[alpha-32P]adenosine diphosphate. Biochemistry 27:5141–5149.
- Darden T, York D, Pederson L. 1993. Article mesh Ewald: An Nlog(N) method for Ewald sums in large systems. J Chem Phys 98:10089–10092.
- De Marcos Lousa C, Trézéguet V, Dianoux AC, Brandolin G, Lauquin GJ. 2002. The human mitochondrial ADP/ATP carriers: Kinetic properties and biogenesis of wild-type and mutant proteins in the yeast S. cerevisiae. Biochemistry 41:14412–14420.
- Dehez F, Pebay-Peyroula E, Chipot C. 2008. Binding of ADP in the mitochondrial ADP/ATP carrier is driven by an electrostatic funnel. J Am Chem Soc 130:12725–12733.
- Dianoux AC, Noel F, Fiore C, Trézéguet V, Kieffer S, Jaquinod M, 2000. Two distinct regions of the yeast mitochondrial ADP/ATP carrier are photolabeled by a new ADP analogue: 2-azido-3′-O-naphthoyl-[beta-32P]ADP. Identification of the binding segments by mass spectrometry. Biochemistry 39:11477–11487.
- Dolce V, Scarcia P, Iacopetta D, Palmieri F. 2005. A fourth ADP/ATP carrier isoform in man: Identification, bacterial expression, functional characterization and tissue distribution. FEBS Lett 579:633–637.
- Driessen AJ, de Vrij W, Konings WN. 1985. Incorporation of beef heart cytochrome c oxidase as a proton-motive force-generating mechanism in bacterial membrane vesicles. Proc Natl Acad Sci USA 82:7555–7559.
- Duée ED, Vignais PV. 1968. Atracyloside-sensitive translocation of phosphonic acid analogues of adenine nucleotides in mitochondria. Biochem Biophys Res Commun 30:546–553.
- Feller SE, Mackerell AD Jr. 2000. All-atom empirical force field for nucleic acids: I. Parameter optimization based on small molecule and condensed phase macromolecular target data. J Phys Chem B104:7510–7515.
- Heidkamper D, Müller V, Nelson DR, Klingenberg M. 1996. Probing the role of positive residues in the ADP/ATP carrier from yeast. The effect of six arginine mutations on transport and the four ATP versus ADP exchange modes. Biochemistry 35:16144–16152.
- Hénin J, Shinoda W, Klein ML. 2008. United-atom acyl chains for CHARMM phospholipids. J Phys Chem B 112:7008–7015.
- Humphrey W, Dalke A, Schulten K. 1996. VMD – visual molecular dynamics. J Mol Graphics 97:25–27.
- Jasien P, Fitzgerald G. 1990. Molecular dipole moments and polarizabilities from local density functional calculations: Application to DNA base pairs. J Chem Phys 93:2554.
- Klingenberg M. 2008. The ADP and ATP transport in mitochondria and its carrier. Biochim Biophys Acta 1778:1978–2021.
- Krämer R, Klingenberg M. 1980. Enhancement of reconstituted ADP, ATP exchange activity by phosphatidylethanolamine and by anionic phospholipids. FEBS Lett 119:257–260.
- Krammer EM, Ravaud S, Dehez F, Frelet-Barrand A, Pebay-Peyroula E, Chipot C. 2009. High-chloride concentrations abolish the binding of adenine nucleotides in the mitochondrial ADP/ATP carrier family. Biophys J 97:L25–L27.
- Kunji ER. 2004. The role and structure of mitochondrial carriers. FEBS Lett 564:239–244.
- Kunji ER, Slotboom DJ, Poolman B. 2003. Lactococcus lactis as host for overproduction of functional membrane proteins. Biochim Biophys Acta 1610:97–108.
- Mackerell AD Jr, Bashford D, Bellott M, Dunbrack RL, Evanseck JD, Field MJ, 1998. All-atom empirical potential for molecular modeling and dynamics studies of proteins. J Phys Chem B102:3586–3616.
- Mackerell AD Jr, Feig M, Brooks CL 3rd. 2004. Extending the treatment of backbone energetics in protein force fields: Limitations of gas-phase quantum mechanics in reproducing protein conformational distributions in molecular dynamics simulations. J Comput Chem 25:1400–1415.
- Miroux B, Walker JE. 1996. Over-production of proteins in Escherichia coli: Mutant hosts that allow synthesis of some membrane proteins and globular proteins at high levels. J Mol Biol 260:289–298.
- Monne M, Chan KW, Slotboom DJ, Kunji ER. 2005. Functional expression of eukaryotic membrane proteins in Lactococcus lactis. Protein Sci 14:3048–3056.
- Müller V, Heidkamper D, Nelson DR, Klingenberg M. 1997. Mutagenesis of some positive and negative residues occurring in repeat triad residues in the ADP/ATP carrier from yeast. Biochemistry 36:16008–16018.
- Nury H, Dahout-Gonzalez C, Trézéguet V, Lauquin GJ, Brandolin G, Pebay-Peyroula E. 2006. Relations between structure and function of the mitochondrial ADP/ATP carrier. Annu Rev Biochem 75:713–741.
- Palmieri F. 2004. The mitochondrial transporter family (SLC25): Physiological and pathological implications. Pflugers Arch 447:689–709.
- Pebay-Peyroula E, Dahout-Gonzalez C, Kahn R, Trézéguet V, Lauquin GJ, Brandolin G. 2003. Structure of mitochondrial ADP/ATP carrier in complex with carboxyatractyloside. Nature 426:39–44.
- Pfaff E, Klingenberg M. 1968. Adenine nucleotide translocation of mitochondria. 1. Specificity and control. Eur J Biochem 6:66–79.
- Phillips JC, Braun R, Wang W, Gumbart J, Tajkhorshid E, Villa E, 2005. Scalable molecular dynamics with NAMD. J Comput Chem 26:1781–1802.
- Ravaud S, Bidon-Chanal A, Blesneac I, Machillot P, Juillan-Binard C, Dehez F, 2012. Impaired transport of nucleotides in a mitochondrial carrier explains severe human genetic diseases. ACS Chem Biol 7:1164–1169.
- Robinson AJ, Kunji ER. 2006. Mitochondrial carriers in the cytoplasmic state have a common substrate binding site. Proc Natl Acad Sci USA 103:2617–2622.
- Robinson AJ, Overy C, Kunji ER. 2008. The mechanism of transport by mitochondrial carriers based on analysis of symmetry. Proc Natl Acad Sci USA 105:17766–17771.
- Tuckerman M, Berne BJ, Martyna GJ. 1992. Reversible multiple time scale molecular dynamics. J Chem Phys 97:1990–1999.
- Wang Y, Tajkhorshid E. 2008. Electrostatic funneling of substrate in mitochondrial inner membrane carriers. Proc Natl Acad Sci USA 105:9598–9603.