Abstract
The galanin receptor family comprises of three members, GalR1, GalR2 and GalR3, all belonging to the G-protein-couple receptor superfamily. All three receptors bind the peptide hormone galanin, but show distinctly different binding properties to other molecules and effects on intracellular signaling. To gain insight on the molecular basis of receptor subtype specificity, we have generated a three-dimensional model for each of the galanin receptors based on its homologs in the same family. We found significant differences in the organization of the binding pockets among the three types of receptors, which might be the key for specific molecular recognition of ligands. Through docking of fragments of the galanin peptide and a number of ligands, we investigated the involvement of transmembrane and loop residues in ligand interaction.
Keywords::
Introduction
Galanin is a biologically active neuropeptide binding to its receptors and therefore interfering with various neuronal activities. It is a product of post-translational process of 121AA long prepro-galanin and consists of 30 amino acids in humans (Evans et al. Citation1993). The N-terminal part of galanin, which includes residues 1–14, is highly conserved between species and is therefore considered to play a crucial role in its biological activity and receptor interaction (Branchek et al. Citation2000). The biological function of the C-terminal, which does not take part in receptor binding, is not fully known, but it has been suggested that it is involved in protecting the peptide against proteolytic attacks (Bedecs et al. Citation1995). Galanin primarily plays the role of an inhibitory hyperpolarizing regulator on the release of neurotransmitters. It often inhibits the release of acetylcholine from ventral hippocampus (Fisone et al. Citation1987, Laplante et al. Citation2004), norephedrine and serotonin release from LC and DRN neurons, respectively (Fuxe et al. Citation1998, Hökfelt et al. Citation1998, Kehr et al. Citation2002), glutamate release from hippocampal and hypothalamic slices (Zini et al. Citation1993), and dopamine release from hypothalamic slices (Ericson and Ahlenius Citation1999, Nordström et al. Citation1987). Therefore, galanin has been implicated in regulating several physiological functions such as memory and cognition, seizure, nociception, spinal reflexes, affection, feeding and hormone release (Wrenn and Crawley Citation2001, Robinson Citation2004, Lundström et al. Citation2005, Wiesenfeld-Hallin et al. Citation2005, Robinson et al. Citation2006). Consequently, galanin signaling contributes to disorders like epilepsy, Alzheimer's disease, stroke, emotional disorders and drug addiction by signaling via its receptors (Fathi et al. Citation1997, Branchek et al. Citation2000), all belonging to the G-protein-coupled receptor (GPCR) superfamily. The three galanin receptors share relatively low sequence homology of 45–50% and exhibit differences in intracellular signaling (Florén et al. Citation2000). Stimulation of GalR1 or GalR3 and GalR2 has the opposite effect on development of depression-like phenotype (Kuteeva et al. Citation2008).
Owing to its involvement in processes regulating neurotransmitters and the development of the nervous system, galanin receptors are important drug targets. Development of subtype-selective ligands is a challenging task partially due to a lack of understanding of galanin receptor subtype-specific roles in brain physiology. Despite the recent discovery of inhibitory non-peptide compounds (Konkel et al. Citation2006, Zhang et al. Citation2009) able to bind and regulate the function of galanin receptors, finding subtype-specific, high-affinity binding compounds is highly desired. Application of molecular modeling can be helpful in identifying important interactions and pharmacophores that can be verified by, e.g., site-directed mutagenesis. The aim in molecular docking is to find an optimized conformation for both the protein and the ligand such that the free energy of the whole system is at a local minimum (Lengauer and Rarey Citation1996) being one of biologically representative states. Docking plays an important role in drug design on many levels from binding sites search, through virtual screening of large ligand libraries, and detailed studies of interaction mechanism where finding the state in which ligand and the receptor protein are geometrically and energetically in the most favorable state is crucial for successful drug activity (Teodoro et al. Citation2001).
To investigate differences between galanin receptors and to identify key receptor-ligand interactions underlying receptor subtype specificity we have generated three-dimensional models of galanin receptors subtypes and performed for the first time flexible-ligand docking studies of galanin peptide fragment (2–6) and several other substrates. Previously published work discusses rigid protein-protein docking of galanin and exhibits limitations to study receptor specificity: Predefined galanin geometry that cannot fit to protein increasing false-negative complex discrimination due to steric clashes and galanin receptors models build only on rhodopsin structure (Parthiban and Shanmughavel Citation2007). Introduction of flexibility makes it possible to overcome the first obstacle and using recently solved structures of adrenergic receptors makes it possible to build better GPCR models (Runesson et al. Citation2010).
In addition to that, criteria such as positions of the transmembrane region in sequence alignments, volume and solvent accessible surface of binding site help to rank protein and complex models. Finally, GPCR subtype-specific ligand binding properties are determined by co-occurrence of residues found previously.
Materials and methods
illustrates the modeling strategy that we applied to study galanin receptors' ligand binding properties.
Figure 1. Overview of structure prediction and docking pipeline applied to galanin receptors: Multiple sequence alignments of targets and templates; manual corrections of alignments based on predicted membrane topology and documented features of binding residues. The alignments are used for the creation of a large number of potential receptor models. Model filtering and selection of best models based on energy scoring function and cavity volume, docking of ligands, selection of best scoring poses and receptor-ligand contact analysis. This Figure is reproduced in colour in Molecular Membrane Biology online.
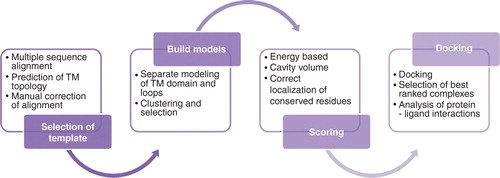
Protein templates for homology modeling
The templates of the homology model chosen were: The crystal structures of the human beta2 adrenergic receptors, PDB ID: 2R4R and 2R4S (Rasmussen et al. Citation2007), PDB ID: 2RH1 (Cherezov et al. Citation2007); turkey beta1 adrenergic receptor PDB ID: 2VT4 (Warne et al. Citation2008) and squid rhodopsin from Todarodes pacificus PDB ID: 2Z73 (Murakami and Kouyama Citation2008). All these crystal structures belong to the rhodopsin family in the G-protein-couple receptor (GPCR) superfamily that is characterized by seven transmembrane (TM) α-helices (Probst et al. 1992.). The structure files were downloaded from PDB (Berman et al. Citation2000). The sequence identities of GalR with the templates were between 23 and 28% (). For modeling, both multiple and single sequence alignments were used.
Table I. Sequence identity between galanin receptors and template alignments.
Sequence alignment and topology prediction
Sequence alignment between galanin receptors and the templates were created using Kalign 2.0 (Lassmann and Sonnhammer Citation2005), a method employing the Wu-Manber string-matching algorithm. TMHMM 2.0 (Krogh et al. Citation2001) and Topcons (Bernsel et al. Citation2009) were used to obtain transmembrane topology prediction of the receptors. TM segments were identified by combining the predicted TM topology predictions for the galanin receptors with the TM segments observed in the crystal structures of the templates. These were then used to manually alter the multiple sequence alignments with the aim to align the helical regions and loops of the sequences and simultaneously reduce the gaps present within helical regions.
Additional factors such as conserved residues among GPCRs suggested to be involved in important structural and functional features were also considered (Baldwin Citation1994). In particular, we used a pair of cysteine residues creating a disulfide bond between extracellular loop (ECL) 1 and ECL 2 present in all three galanin receptors and two known motifs found in GPCR family also present in galanin receptors: DRY motif at the boundary of TM helix 2 and intracellular loop 2, known to be important in receptor signaling; and NPXXY motif at the C-term end of TM helix 7, which is known to play a significant role in signaling and receptor activation (Gales Citation2000, Fritze et al. Citation2003).
Structure prediction, validation criteria and comparison of receptors
The three-dimensional structural models for the three galanin receptors GalR1 (349 AA), GalR2 (387 AA), and GalR3 (368 AA) were built with MODELLER9v1 (Eswar et al. Citation2007). Initially, five models were built for each receptor and template. Best models were identified using a combination of model quality scores: The Discrete Optimized Protein Energy (DOPE) score, a statistical potential used to assess homology models (Eswar et al. Citation2007), the GA341 score, a method used for model assessment based on the percentage sequence identity between the template and the model (Melo et al. Citation2002), and by visually inspecting the model, in particular the binding site region. To control the quality of models, we checked whether specific residues implicated to be involved in ligand binding were accessible (). Finally, loop refinement was applied to ECLs and to the N-termini. The loops with the lowest energies were used in the final model.
Table II. Amino acid residues involved in ligand binding to galanin receptor subtypes.
The receptors' structures were compared by calculation of displacement and inter-atomic contacts of corresponding amino acid side chains. Two residues were assumed to be in contact if any of the atoms were within 4.0Å. A list of interacting residues includes those found in mutation studies of galanin receptors ().
All cavities were detected automatically without preselecting any binding residues by CASTp program (Dundas et al. Citation2006).
Ligands selected for docking
The applicability of flexible ligand docking is limited to systems with maximally 32 rotatable bonds. When selecting all σ-σ and π-σ bonds as rotatable in the galanin case this limit is reached by the five first amino acids. It has been shown that the first glycine moiety does not play an important role in binding (Wang et al. Citation1997). Therefore, the five amino acid-long galanin fragment (WTLNS) starting from 2nd position in the sequence was chosen for the docking studies. Additional ligands selected for docking include the agonist compound galnon (Saar et al. Citation2002), the RGRGNW peptide, i.e., the first six residues of M1145 (Runesson et al. Citation2009), in which Gly1 is substituted with five AA long amphipathic peptide RGRGN, and two synthetic compounds, the antagonist SNAP37889 (1,3-dihydro-1-phenyl-3-[[3- (trifluoromethyl)phenyl]imino]-2H-indol-2-one) and its variant SNAP398299 1- (3- (2- (pyrrolidin-1-yl)ethoxy) phenyl)-3- (3- (trifluoromethyl)phenylimino)indolin-2-one (Konkel et al. Citation2006). As for the SNAP398299 compound, two docking conformations were chosen for the docking to GalR3, the linear conformation of the ligand, which is energetically and sterically preferred, and the bent conformation. All ligands were built using the Maestro program and were minimized with the OPLS2005 force field using Ligprep (Schrodinger, LLC, NY, USA).
Docking
The docking of ligands into receptor structure was performed using Glide (Schrodinger, LLC, NY). Protein Preparation Wizard was applied to initial preprocessing and optimization of hydrogen bond network followed by energy minimization with OPLS2005 force field. Next, using the receptor grid generation tool, a grid representing intermolecular interactions was prepared with the grid box being centered on selected residues in the binding site. Optimized ligands were subsequently docked to the receptor selecting the XP mode. The molecular docking was carried out without any constraints. Post-docking minimization was performed and ligands with root-mean-square deviation (RMSD) < 0.5Å were discarded to avoid similar poses. Analysis of the docking results was based on three factors including estimation of ligand binding energy, contact analysis, and clustering of docked poses. First, most poses tend to share one of preferred orientation in the binding pocket; thus, RMSD clustering was employed to select representatives of each cluster and narrow down the number of possible solutions. Secondly, Glide score and E-model scoring functions produced independent binding energy rankings of all poses and clusters. The Glide score is preferred in predicting differences in binding affinities between different ligands while E-model, which is a combination of energy grid score, Glide score, and the internal energy of the ligand, is preferred at ranking different poses of the same ligand (Halgren et al. Citation2004). Interactions with key residues as found in literature served as additional criteria. Low energy clusters with members showing preference in binding to these residues were preferred.
All Figures presenting 3D structures of protein and complexes were created with Pymol (De Lano Citation2002).
Results
Galanin receptors sequence alignment
The sequence identities between GalR1 and GalR2 and between GalR1 and GalR3 are 37% and 33%, respectively, while GalR2 and GalR3 share a higher degree of identity at 55% (). The lowest similarity is observed at the N- and C-terminal. In , TM helices are represented by rectangular boxes indicating the start and end of each helix and are connected with convex or concave lines representing extracellular and intracellular loops. Multiple sequence alignments were modified to remove gaps to improve alignment of TM domains (according to TM prediction). Applying this strategy improved modeling of helices that tend to be broken in unsupervised models. Nevertheless, final definitions of TM domains differ slightly between predictions produced by TMHMM and Topcons (blue) and in the final model (red) (overall helices tend to be longer than predicted). Most importantly, modified alignments result in potential binding residues placed in receptor cavities. Multiple sequence alignments of target and template sequences before and after manual alignment correction for each receptor subtype are shown in Supplementary Figures S1–3 (available online).
Figure 2. Multiple sequence alignment between the three galanin receptors and template structures (2R4R, 2RH1, 2ZIY). Rectangular boxes show the start and end residue positions for each transmembrane helix of GalR1, GalR2, and GalR3 subtypes. Convex lines connecting boxes denote the extracellular loops and the concave lines denote the intracellular loops. Colours highlight specific residues: in yellow – motifs characteristic to GPCR receptors; in blue – start and end of the transmembrane helices predicted by TMHMM; in red – start and end of the transmembrane helices in our models of the receptors; in green – overlap of residues in blue and red. Amino acids compared in are highlighted in purple; in italic are residues tested in site-directed mutagenesis studies (); in bold are residues in direct contact with ligands as found by docking (). This Figure is reproduced in colour in Molecular Membrane Biology online.
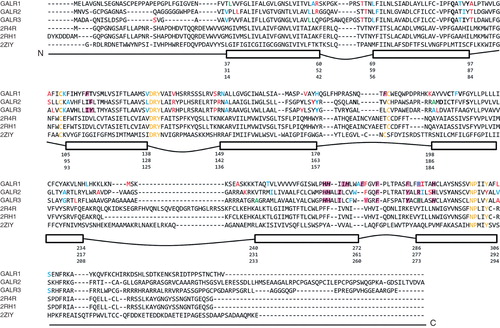
Table III. Cavity volumes and surface areas.
Table IV. Comparison of galanin receptors binding sites: Measuring spatial differences of residues positions corresponding in sequence and/or structural alignments. RMS calculated after molecular fit of TM backbone.
Table V. Selected ligand binding residues in galanin receptors found by docking studies.
General characteristics of receptor models
The three-dimensional models for each galanin receptor subtype were built based on manually adjusted multiple sequence alignments. Details of selection process are described in the Supplementary Data, available online. All finally selected models share the common structural features known to GPCR, consisting of an extracellular N-terminus, seven TM helices connected by three intracellular loops and three ECLs, and ending with an intracellular C-terminus (Probst et al. Citation1992). In all three cases, the seven TM α-helices are arranged compactly forming the core of the receptor, and are arranged in a counterclockwise fashion when viewed from the extracellular side, agreeing with the helical and loop arrangements observed in GPCR (Baldwin Citation1993, Schwartz Citation1994, Palczewski et al. Citation2000). Also, the helix at the C-termini parallel to the membrane, known also as helix 8 is present in all models. This helix is observed in all currently available crystal structures and it has been suggested that it acts as a conformational switch for the receptor (Krishna et al. Citation2002).
The lengths of C- and N-termini are varying between the three receptor subtypes. At the C-terminal, 37 residues in GalR1, 30 residues in GalR2 and 13 residues in GalR3, are not modeled. Since the N-terminal may play an important role in ligand binding, it is incorporated in receptor models and is 80 residues long in GalR2, 62 residues long in GalR3 but only 47 residues long in GalR1.
Finally, our galanin receptor models are in accordance with the positive-inside rule (von Heijne Citation1989). The number of positively charged residues found on the helices at the boundary with the intracellular side (15 for GalR1, 11 for GalR2, and 11 for GalR3) is higher than on the boundary with the extracellular site (four for GalR1, three for GalR2, and six for GalR3).
Comparison of binding sites
In order to compare binding sites of the three galanin receptor subtypes, we calculated volumes of binding cavity and compared residues responsible for receptor activity. Visual inspection revealed the binding cavity in GalR3 as much narrower than in the other two receptors (). In order to quantify these differences, solvent accessible surface and volume of binding pockets were calculated using the CASTp program. Among all cavities detected, those on the extracellular site were summed up and their total volumes and surfaces are presented in . The selection of loops in the modeling procedure was guided to favor a larger volume of resulting models (see minimal and maximal V before and after loop refinement in ). The volume of the GalR2 binding cavity is roughly twice the size of GalR1 and three times larger than the cavity volume of GalR3. However, the solvent accessible surface area of the GalR3 cavity is of similar size as in GalR1, indicating the narrow and tunnel-like shape of the binding cavity. Such a cavity would prefer longer but not bulky ligands, whereas GalR1 should be less specific in ligand shape recognition.
Figure 3. Binding sites of galanin receptors subtypes with best binding ligands: (A) GalR1 and (B) GalR2 docked to galanin(2-6) and SNAP398299; GalR3 in complex with (C) two poses of galanin(2-6) and (D) two poses of SNAP398299. This Figure is reproduced in colour in Molecular Membrane Biology online.
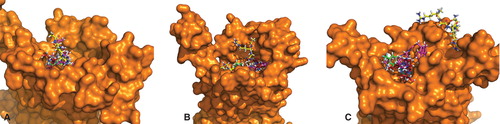
Analysis of sequence alignments reveals almost perfect concordance of TM helices 1–3, which is reflected by the smallest differences in predicted structures. The region between ECL2 and TM helix 7 is more variable and contributes to receptor subtype specificity by both affecting binding cavity size as well as changing the pattern of ligand interacting residues. In comparison with GalR2, the largest changes are contributed by ECL2 residues: Tyr177 and 173 for GalR1 and by corresponding Tyr161 and Tyr166 in GalR3. In GalR1, the pocket is more shallow than in GalR2 due to orientation of Tyr96 (TM helix 2), Met119 (TM helix 3), His264 and His267 (TM helix 6) and His289 (TM helix 7). Similarly, in GalR3 the deepest part of the cavity is different mainly due Tyr103 and His99 (TM helix 3), Ile255 (TM helix 6) and Arg273 and His 277 (TM helix 7). In addition, the upper part of the cavity is made narrow by residues close to the N-termini: Pro12, Phe24 (TM helix 1), Tyr83 and Gln79 (TM helix 2) and Leu274 (TM helix 7). Thus orientation of these particular residues may influence residue subtype specificity, even though the backbone structures of TM segments are perfectly overlapped in all cases.
Secondly, we calculated the RMSD of corresponding residues using the assumption that binding site residues that are the key for receptor activity should be exposed in order to interact with ligands. shows a comparison of some side chains arrangements after alignment of receptors TM domains; all comparisons are listed in . As expected, the largest differences were observed between amino acid in ECLs with the exception of a few hydrophobic residues in ECL3 that share similar orientation in all receptor subtypes. Overall, the smallest differences can be seen between conserved residues in TM helices 6 and 7 that participate in ligand binding (). For example, in both GalR1 compared with GalR2 and in GalR2 compared with GalR3 corresponding His residues are well aligned (RMSD < 1.5Å). Clustering of hydrophobic residues plays a role in all receptor subtypes but precise placement of side chains is not required. Positions of corresponding Phe, Tyr and Ile residues in TM helix 6 and ECL3 are relatively more similar in comparison of GalR1 and GalR3 (RMSD < 6Å) than in comparisons between GalR1 and GalR2 and between GalR2 and GalR3 (RMSD > 7Å). A detailed description of the differences is presented in Supplementary Data, online.
Docking
To investigative the binding properties of galanin receptors, we docked galanin(2-6), peptide RGRGNW, galnon, SNAP37889 and SNAP398299, to two sets of models (with and without disulfide bonds) of galanin receptor subtypes. Disulfide bond is predicted by similarity to other GPCRs to link TM helix 2 with ECL 3 (108–187 in GalR1). None of the ligands were able to dock inside the binding pocket when disulfide bond was present. All ligand poses were scattered outside of the pocket and no significant clusters were found proving lack of specificity in binding.
Docking to models without disulfide bonds reveals ligands buried in the central cavity to degree depending on ligand size and cavity dimensions (). Each docking yields a number of clusters showing a general reproducibility of ligand binding modes. The ligands are stabilized in the binding pocket through hydrogen bonding and van der Waals, π-π and polar interactions. The docked poses were clustered and the cluster with the best E-model score was selected for further studies.
The docking studies show that the receptor subtypes have different ligand affinities. They differ in binding energy and specificity, estimated by the presence of hydrogen bonding or other orientation-dependent interactions, and in binding precision, smaller number of more populated clusters vs. large number of lower populated clusters (see Supplementary Data for detailed description as well as Tables S1, S2 and S3, available online). RGRGNW binds more specific to GalR2 than to GalR1, and shows no binding to GalR3. Differences are present also for docking of galanin(2-6), which binds less specific to GalR1 and GalR3. The synthetic indol-2-one analogs and galnon are generally less specific binders than RGRGNW and galanin(2-6). We did not detect any specificity in SNAP37889 binding. SNAP398299 binds slightly more specific to GalR1 and galnon to GalR2. If ligands are sorted by binding affinity to respective receptor subtypes, the order can be formed as follows: GalR1 – galnon > galanin(2-6) > SNAP398299 > RGRGNW > SNAP37889; GalR2 – galanin(2-6) > galnon > RGRGNW > SNAP398299 > SNAP37889; GalR3 – galnon > galanin(2-6) > SNAP398299 > SNAP37889.
Detailed pattern of interactions
Persistent interaction of ligands with specific residues may indicate they importance in receptor function; most commonly interacting residues are collected in .
Here we briefly discuss ligand binding in GalR1 and GalR2. Experimentally validated predictions of GalR3 residues are presented elsewhere (Runesson et al. Citation2010). Site-directed mutagenesis and molecular modeling of GalR1 localize potential binding sites at the extracellular loops and the superficial regions of the receptor (Berthold et al. Citation1997, Kask et al. Citation1998). From our docking studies of GalR1, we were able to identify a number of amino-acid residues that may play a role in ligand binding (pharmacophores); h-bonding of Gln92 and Tyr96 in TM helix 2, h-bonding Cys108 and hydrophobic interactions of Met119, Phe115 and Thr116 in TM helix 3, hydrophobic Val206 in TM helix 5 and π-π interacting His267 in TM helix 6. Interestingly, two of the selected pharmacophores were confirmed by previous experimental work. When studying GalR1 subtype, a binding site for galanin suggests residue His267 in TM helix 6 to be involved in binding of synthetic compounds (Kask et al. Citation1998). In addition, Phe115 has been proposed to play a role in galanin binding (Berthold et al. Citation1997) and is structurally important for the binding site of the receptor (Church et al. Citation2002).
Subsequently, potential pharmacophores were identified in GalR2: hydrophobic interactions of Met109 and Phe106 in TM helix 3, h-bonding Thr173, Tyr160, and Tyr164 in ECL 2 and hydrogen bonding Asp188 and Thr191 in TM helix 5. Two other residues identified as important in GalR2 ligand binding are located in TM helix 6: Ile256 (hydrophobic interaction) and His253 (cation-π). Alanin mutagensis of Ile256 results in loss of binding and receptor activation by GalR2 selective galanin(2-11) and also the cause for a lower binding affinity for full length galanin (Lundström et al. Citation2007). Also, alanin mutant of His253 results in a complete loss of galanin binding (Lundström et al. Citation2007).
Both His253 in GalR2 and His264 in GalR1, interact with the tryptophan residue in galanin(2-6). In a ligand binding study of GalR2 (Lundström et al. Citation2007), two conserved histidine residues in TM helix 6 – His253 and His252 – were shown to be significant in ligand binding to GalR2, and were previously suggested to interact with tryptophan in the galanin peptide (Berthold et al. Citation1997, Church et al. Citation2002). These findings indicate a possible parallel role of His264 in GalR1.
Discussion
The use of multiple crystal structures of GPCRs in the structure prediction process enabled us to create improved structures of the three galanin receptors. The quality of the models was controlled in three steps: Energy, cavity volume and orientation of specific residues. Models of GalR1 and GalR3 have similar properties (cavity volume and arrangement of specific residues in binding sites) and are remarkably different from GalR2.
The major difficulty in getting the correct models representing receptor's open state lay in the incorporating of extracellular disulfide bond predicted by similarity to other GPCR structures. In docking to models without disulfide bond, all ligands under study were docked inside a binding pocket formed in between in the core of the receptor; large peptide ligands interact at the same time with the TM helices and extracellular loops. This characteristic binding is also postulated for other neuropeptides including NPY (Sautel et al. Citation1995, Sjödin et al. Citation2006), angiotensin (Clément et al. Citation2005), and somatostatin (Strnad and Hadcock Citation1995). However, docking to models with this disulfide bond present yielded no good binding poses for any ligands, indicating that the reduced form must exist for ligand binding.
Galnon was found to be amongst the best binders, binding with approximately equal energy and mode to all three galanin receptors, thus not showing any particular selectivity for any of the receptor subtypes. This confirms previous findings where galnon was binding to other receptors, consequently leading to a decrease in its usefulness in binding studies (Florén et al. Citation2005, Lu et al. Citation2005). From the two non-peptide antagonists: SNAP37889 and its variant SNAP398299, the latter binds stronger to all receptors. The difference is most clearly noted in a more specific binding to GalR1 and GalR3. Simultaneously the binding specificity of galanin(2-6) decreases in following order: GalR2 > GalR3 > GalR1, i.e., SNAP398299 binds to GalR3 with more specificity, as is confirmed experimentally (Konkel et al. Citation2006). Finally, RGRGNW binds more specifically to GalR2 than to GalR1, and shows no binding to GalR3. This observation is in accordance with a study of binding to galanin receptors in cellular models presenting M1145 as GalR2-specific agonist (Runesson et al. Citation2009).
Experimental studies also show that the galanin peptide binds with a high affinity to all three galanin receptor subtypes. The shortened N-terminal versions of galanin, galanin(1-16) have been shown to bind equally well to GalR1 and GalR2 as compared to non-truncated version (Wang et al. Citation1997). A much stronger effect is observed for truncation of the N-terminal glycine; galanin(2-11) completely loses affinity towards GalR1 (Liu et al. Citation2001). Both of these facts are reflected in our study: galanin(2-6) binds significantly stronger to GalR2 and with a lower binding energy for the GalR1 and GalR3 subtypes. This difference for receptor subtype selectivity of shorter galanin fragment may be explained by the influence of non-specific binding of the highly hydrophobic AGYLLGP-galanin(7-13) over less hydrophobic C-termini. Binding mode could be reverted with hydrophobic residues packed together and C-termini more likely packed on the top of receptor ECLs and exposed to solvent. Moreover, the volume of the central cavity and space in between ECLs allows fitting of at most penta- or hexapeptide. Other peptides known to interact with galanin receptors could potentially adopt a similar mode of binding. Galanin-like peptide (GALP) (Lawrence and Fraley Citation2010), for instance, is twice as long but contains fragment (33–46) identical with galanin. Ligand docking attempts to dock longer galanin peptides to the receptors failed as the proposed helical model of the N-terminal region of galanin was not able to enter the binding pocket in any of the receptors, which questions validity of the previously proposed helical galanin structure (Morris et al. Citation1995, Ohman et al. Citation1998). Both unconstrained geometry optimization and flexible ligand docking presented here do not prefer helical conformation.
In comparison of ligand binding across the three galanin receptors, the most favorable binding energies were assigned to GalR2 complexes. However, this does not imply ligand selectivity for the GalR2 subtype. In particular, indol-2-one analogs (Swanson et al. Citation2005) were reported as selective activators of GalR3, while the N-terminal truncated galanin(2-29) shows higher affinity for GalR2 (Wang et al. Citation1997). This observation may be explained by our docking results presenting favorable pharmacophores in the binding pocket of GalR2 enabling high affinity binding of a number of ligands inside the cavity of the receptor.
Conclusions
By applying homology modeling, we were able to build three-dimensional structures of the three human galanin receptor subtypes. Docking of ligand to these models has drawn a more detailed picture of the binding pocket of galanin receptors and of the ligand-receptor interactions, which is a good starting point for any drug design strategies. On the other hand it may serve as a reliability test for the receptor model by itself. The protocol was positively verified on GalR3. Competitive binding studies of GalR3 mutants allow us to predict residues crucial for receptor activity (Runesson et al. Citation2010).
Supplementary Data and Tables S1, S2 and S3.
Download MS Word (98.6 KB)Acknowledgements
The EU 7th Framework Program is gratefully acknowledged for support to the EDICT project, contract No: FP7-HEALTH-F4-2007-201924. This work was also supported by grants from the Swedish National Research Councils and SSF (the Foundation for Strategic Research) to AE.
Declaration of interest: The authors report no conflicts of interest. The authors alone are responsible for the content and writing of the paper.
References
- Baldwin JM. 1993. The probable arrangement of the helices in G protein-coupled receptors. EMBO J 12:1693–1703.
- Baldwin JM. 1994. Structure and function of receptors coupled to G proteins. Curr Opin Cell Biol 6:180–190.
- Bedecs K, Langel Ü, Bartfai T. 1995. Metabolism of galanin and galanin (1-16) in isolated cerebrospinal fluid and spinal cord membranes from rat. Neuropeptides 29:137–143.
- Berman HM, Westbrook J, Feng Z, Gilliland G, Bhat TN, Weissig H, 2000. The protein data bank. Nucleic Acids Res 28:235–242.
- Bernsel A, Viklund H, Hennerdal A, Elofsson A. 2009. TOPCONS: Consensus prediction of membrane protein topology. Nucleic Acids Res 37:W465–W468.
- Berthold M, Kahl U, Juréus A, Kask K, Nordvall G, Langel U, 1997. Mutagenesis and ligand modification studies on galanin binding to its GTP-binding-protein-coupled receptor GalR1. Eur J Biochem/FEBS 249:601–606.
- Branchek TA, Smith KE, Gerald C, Walker MW. 2000. Galanin receptor subtypes. Trends Pharmacolog Sci 21:109–117.
- Cherezov V, Rosenbaum DM, Hanson MA, Rasmussen SGF, Thian FS, Kobilka TS, 2007. High-resolution crystal structure of an engineered human beta2-adrenergic G protein–coupled receptor. Science (NY) 318:1258–1265.
- Church WB, Jones KA, Kuiper DA, Shine J, Iismaa TP. 2002. Molecular modelling and site-directed mutagenesis of human GALR1 galanin receptor defines determinants of receptor subtype specificity. Protein Engineer 15:313–323.
- Clément M, Martin SS, Beaulieu M-E, Chamberland C, Lavigne P, Leduc R, 2005. Determining the environment of the ligand binding pocket of the human angiotensin II type I (hAT1) receptor using the methionine proximity assay. J Biologic Chem 280:27121–27129.
- De Lano W. 2002. The Pymol molecular graphics system.
- Dundas J, Ouyang Z, Tseng J, Binkowski A, Turpaz Y, Liang J. 2006. CASTp: Computed atlas of surface topography of proteins with structural and topographical mapping of functionally annotated residues. Nucleic Acids Res 34:W116–W118.
- Ericson E, Ahlenius S. 1999. Suggestive evidence for inhibitory effects of galanin on mesolimbic dopaminergic neurotransmission. Brain Res 822:200–209.
- Eswar N, Webb B, Marti-Renom MA, Madhusudhan MS, Eramian D, Shen M-Y, 2007. Comparative protein structure modeling using MODELLER. Curr Protoc Prot Sci/ edit board Coligan JE, Chapt. 2, Unit 2.9.
- Evans H, Baumgartner M, Shine J, Herzog H. 1993. Genomic organization and localization of the gene encoding human preprogalanin. Genomics 18:473–477.
- Fathi Z, Cunningham AM, Iben LG, Battaglino PB, Ward SA, Nichol KA, 1997. Cloning, pharmacological characterization and distribution of a novel galanin receptor. Brain Res Mol Brain Res 51:49–59.
- Fisone G, Wu CF, Consolo S, Nordström O, Brynne N, Bartfai T, 1987. Galanin inhibits acetylcholine release in the ventral hippocampus of the rat: Histochemical, autoradiographic, in vivo, and in vitro studies. Proc Natl Acad Sci USA 84:7339–7343.
- Florén A, Land T, Langel Ü. 2000. Galanin receptor subtypes and ligand binding. Neuropeptides 34:331–337.
- Florén A, Sollenberg U, Lundström L, Zorko M, Stojan J, Budihna M, 2005. Multiple interaction sites of galnon trigger its biological effects. Neuropeptides 39:547–558.
- Fritze O, Filipek S, Kuksa V, Palczewski K, Hofmann KP, Ernst OP. 2003. Role of the conserved NPxxY(x)5,6F motif in the rhodopsin ground state and during activation. Proc Natl Acad Sci USA 100:2290–2295.
- Fuxe K, Jansson A, Diaz-Cabiale Z, Andersson A, Tinner B, Finnman UB, 1998. Galanin modulates 5-hydroxytryptamine functions. Focus on galanin and galanin fragment/5-hydroxytryptamine1A receptor interactions in the brain. Annals NY Acad Sci 863:274–290.
- Gales C. 2000. Mutation of Asn-391 within the conserved NPXXY motif of the cholecystokinin B receptor abolishes Gq protein activation without affecting its association with the receptor. J Biolog Chem 275:17321–17327.
- Halgren TA, Murphy RB, Friesner RA, Beard HS, Frye LL, Pollard WT, 2004. Glide: A new approach for rapid, accurate docking and scoring. 2. Enrichment factors in database screening. J Medicinal Chem 47:1750–1759.
- Hökfelt T, Xu ZQ, Shi TJ, Holmberg K, Zhang X. 1998. Galanin in ascending systems. Focus on coexistence with 5-hydroxytryptamine and noradrenaline. Annals NY Acad Sci 863:252–263.
- Kask K, Berthold M, Kahl U, Juréus A, Nordvall G, Langel U, 1998. Mutagenesis study on human galanin receptor GalR1 reveals domains involved in ligand binding. Annals NY Acad Sci 863:78–85.
- Kehr J, Yoshitake T, Wang F-H, Razani H, Gimenez-Llort L, Jansson A, 2002. Galanin is a potent in vivo modulator of mesencephalic serotonergic neurotransmission. Neuropsychopharmacology 27:341–356.
- Konkel MJ, Packiarajan M, Chen H, Topiwala UP, Jimenez H, Talisman IJ, 2006. Amino substituted analogs of 1-phenyl-3-phenylimino-2-indolones with potent galanin Gal3 receptor binding affinity and improved solubility. Bioorgan Medicin Chem Lett 16:3950–3954.
- Krishna AG, Menon ST, Terry TJ, Sakmar TP. 2002. Evidence that helix 8 of rhodopsin acts as a membrane-dependent conformational switch. Biochemistry 41:8298–8309.
- Krogh A, Larsson B, von Heijne G, Sonnhammer EL. 2001. Predicting transmembrane protein topology with a hidden Markov model: Application to complete genomes. J Molec Biol 305:567–580.
- Kuteeva E, Hökfelt T, Wardi T, Ögren SO. 2008. Galanin, galanin receptor subtypes and depression-like behaviour. Cellular Molec Life Sci 65:1854–1863.
- Laplante F, Crawley JN, Quirion R. 2004. Selective reduction in ventral hippocampal acetylcholine release in awake galanin-treated rats and galanin-overexpressing transgenic mice. Regulat Peptides 122:91–98.
- Lassmann T, Sonnhammer ELL. 2005. Kalign – an accurate and fast multiple sequence alignment algorithm. BMC Bioinform 6:298.
- Lawrence CB, Fraley GS. 2010. Galanin-like peptide: Neural regulator of energy homeostasis and reproduction. EXS 102:263–280.
- Lengauer T, Rarey M. 1996. Computational methods for biomolecular docking. Current Opin Struct Biol 6:402–406.
- Liu HX, Brumovsky P, Schmidt R, Brown W, Payza K, Hodzic L, 2001. Receptor subtype-specific pronociceptive and analgesic actions of galanin in the spinal cord: Selective actions via GalR1 and GalR2 receptors. Proc Natl Acad Sci USA 98:9960–9964.
- Lu X, Lundström L, Bartfai T. 2005. Galanin (2-11) binds to GalR3 in transfected cell lines: Limitations for pharmacological definition of receptor subtypes. Neuropeptides 39:165–167.
- Lundström L, Elmquist A, Bartfai T, Langel U. 2005. Galanin and its receptors in neurological disorders. Neuromolec Med 7:157–180.
- Lundström L, Sollenberg UE, Bartfai T, Langel U. 2007. Molecular characterization of the ligand binding site of the human galanin receptor type 2, identifying subtype selective interactions. J Neurochem 103:1774–1784.
- Melo F, Sánchez R, Sali A. 2002. Statistical potentials for fold assessment. Protein Sci 11:430–448.
- Morris MB, Ralston GB, Biden TJ, Browne CL, King GF, Iismaa TP. 1995. Structural and biochemical studies of human galanin: NMR evidence for nascent helical structures in aqueous solution. Biochemistry 34:4538–4545.
- Murakami M, Kouyama T. 2008. Crystal structure of squid rhodopsin. Nature 453:363–367.
- Nordström O, Melander T, Hökfelt T, Bartfai T, Goldstein M. 1987. Evidence for an inhibitory effect of the peptide galanin on dopamine release from the rat median eminence. Neurosci Lett 73:21–26.
- Ohman A, Lycksell PO, Juréus A, Langel U, Bartfai T, Gräslund A. 1998. NMR study of the conformation and localization of porcine galanin in SDS micelles. Comparison with an inactive analog and a galanin receptor antagonist. Biochemistry 37:9169–9178.
- Palczewski K, Kumasaka T, Hori T, Behnke CA, Motoshima H, Fox BA, 2000. Crystal structure of rhodopsin: A G protein-coupled receptor. Science (NY) 289:739–745.
- Parthiban M, Shanmughavel P. 2007. Three dimensional modeling of N-terminal region of galanin and its interaction with the galanin receptor. Bioinformation 2:119–125.
- Probst WC, Snyder LA, Schuster DI, Brosius J, Sealfon SC. 1992. Sequence alignment of the G-protein coupled receptor superfamily. DNA Cell Biol 11:1–20.
- Rasmussen SGF, Choi H-J, Rosenbaum DM, Kobilka TS, Thian FS, Edwards PC, 2007. Crystal structure of the human beta2 adrenergic G-protein-coupled receptor. Nature 450:383–387.
- Robinson JK. 2004. Galanin and cognition. Behav Cognit Neurosci Rev 3:222–242.
- Robinson JK, Bartfai T, Langel Ü. 2006. Galanin/GALP receptors and CNS homeostatic processes. CNS Neurolog Disorders Drug Targets 5:327–334.
- Runesson J, Saar I, Lundström L, Järv J, Langel U. 2009. A novel GalR2-specific peptide agonist. Neuropeptides 43:187–192.
- Runesson J, Sollenberg UE, Jurkowski W, Yazdi S, Eriksson EE, Elofsson A, 2010. Determining receptor-ligand interaction of human galanin receptor type 3. Neurochem Int 57:804–811.
- Saar K, Mazarati AM, Mahlapuu R, Hallnemo G, Soomets U, Kilk K, 2002. Anticonvulsant activity of a nonpeptide galanin receptor agonist. Proc Natl Acad Sci USA 99:7136–7141.
- Sautel M, Martinez R, Munoz M, Peitsch MC, Beck-Sickinger AG, Walker P. 1995. Role of a hydrophobic pocket of the human Y1 neuropeptide Y receptor in ligand binding. Molec Cellular Endocrinol 112:215–222.
- Schwartz TW. 1994. Locating ligand-binding sites in 7TM receptors by protein engineering. Current Opin Biotechnol 5:434–444.
- Sjödin P, Holmberg SKS, Akerberg H, Berglund MM, Mohell N, Larhammar D. 2006. Re-evaluation of receptor-ligand interactions of the human neuropeptide Y receptor Y1: A site-directed mutagenesis study. Biochem J 393:161–169.
- Strnad J, Hadcock JR. 1995. Identification of a critical aspartate residue in transmembrane domain three necessary for the binding of somatostatin to the somatostatin receptor SSTR2. Biochem Biophys Res Communic 216:913–921.
- Swanson CJ, Blackburn TP, Zhang X, Zheng K, Xu Z-QD, Hökfelt T, 2005. Anxiolytic- and antidepressant-like profiles of the galanin-3 receptor (Gal3) antagonists SNAP 37889 and SNAP 398299. Proc Natl Acad Sci USA 102:17489–17494.
- Teodoro ML, Phillips GN Jr, Kavraki LE. 2001. Molecular docking: A problem with thousands of degrees of freedom. IEEE Int Conf Robotics Automat 960–966.
- Wang S, Hashemi T, He C, Strader C, Bayne M. 1997. Molecular cloning and pharmacological characterization of a new galanin receptor subtype. Molec Pharmacol 52:337–343.
- Warne T, Serrano-Vega MJ, Baker JG, Moukhametzianov R, Edwards PC, Henderson R, 2008. Structure of a beta1-adrenergic G-protein-coupled receptor. Nature 454: 486–491.
- Wiesenfeld-Hallin Z, Xu X-J, Crawley JN, Hökfelt T. 2005. Galanin and spinal nociceptive mechanisms: Recent results from transgenic and knock-out models. Neuropeptides 39:207–210.
- Wrenn CC, Crawley JN. 2001. Pharmacological evidence supporting a role for galanin in cognition and affect. Progress Neuro-Psychopharmacol Biolog Psychiatry 25:283–299.
- Zhang L, Robertson CR, Green BR, Pruess TH, White HS, Bulaj G. 2009. Structural requirements for a lipoamino acid in modulating the anticonvulsant activities of systemically active galanin analogues. J Medicinal Chem 52:1310–1316.
- Zini S, Roisin MP, Armengaud C, Ben-Ari Y. 1993. Effect of potassium channel modulators on the release of glutamate induced by ischaemic-like conditions in rat hippocampal slices. Neurosci Lett 153:202–205.
- von Heijne G. 1989. Control of topology and mode of assembly of a polytopic membrane protein by positively charged residues. Nature 341:456–458.