Abstract
The ATP-binding cassette (ABC) transporter ProU from Escherichia coli translocates a wide range of compatible solutes and contributes to the regulation of cell volume, which is particularly important when the osmolality of the environment fluctuates. We have purified the components of ProU, i.e., the substrate-binding protein ProX, the nucleotide-binding protein ProV and the transmembrane protein ProW, and reconstituted the full transporter complex in liposomes. We engineered a lipid anchor to ProX for surface tethering of this protein to ProVW-containing proteoliposomes. We show that glycine betaine binds to ProX with high-affinity and is transported via ProXVW in an ATP-dependent manner. The activity ProU is salt and anionic lipid-dependent and mimics the ionic strength-gating of transport of the homologous OpuA system.
Introduction
Osmotic stress is an important environmental parameter that influences the growth and adaptation of (micro)organisms in a given habitat. Microbial cells employ different strategies to overcome the ‘osmotic challenges' in their environment. For instance, under hyperosmotic conditions, bacteria can accumulate osmoprotective compounds or compatible solutes, such as choline, proline, glycine betaine, carnitine and ectoine (Perroudt and Rudulier Citation1985, Grothe et al. Citation1986, Wood et al. Citation2001). In addition to these zwitterionic compounds, organisms may transiently accumulate potassium ions together with glutamate or other anionic species as is best documented for Escherichia coli (Booth and Higgins Citation1990, Ogahara et al. Citation1995). The accumulation of potassium ions is fast but also disturbs ionic homeostasis of the cell, and over time the potassium and counter ion(s) are replaced by neutral or zwitterionic solutes such as trehalose, proline, glycine betaine and others (Sutherland et al. Citation1986, Lucht and Bremer Citation1994). The accumulation of compatible solutes can be achieved via de novo biosynthesis or rapid uptake of solutes from the medium; the latter mechanism can be more effective in terms of costs of metabolic energy.
Osmoregulatory transport systems have been extensively studied in both Gram-positive and Gram-negative bacteria, e.g., OpuA from Lactococcus lactis and Bacillus subtilis, BetP from Corynebacterium glutamicum, OpuC from Pseudomonas syringae, and ProP and ProU from E. coli (Cairney et al. Citation1985, May et al. Citation1986, Kempf and Bremer Citation1995, Kempf et al. Citation1997, Horn et al. Citation2003, Mahmood et al. Citation2006, Citation2009, Karasawa et al. Citation2011, Perez et al. Citation2011, Culham et al. Citation2012). Other biochemically less well-studied systems include: Ota from Methanosarcina mazei, and ProP and ProU from Yersinia enterocolitica (Schmidt et al. Citation2007, Annamalai and Venkitanarayanan Citation2009). The OpuA, OpuC, Ota and ProU systems are all ATP-binding cassette transporters that have a cystathionine-β-synthase (CBS) module fused to the C-terminus of the nucleotide-binding domain. This CBS module has been shown to be critical for the osmoregulation of the OpuA transporter from L. lactis and OpuC from P. syringae (Biemans-Oldehinkel et al. Citation2006, Chen and Beattie Citation2007, Mahmood et al. Citation2009). A particularly interesting system is ProU from E. coli as the osmotic regulation of its expression is known in great detail (Perroudt and Rudulier Citation1985, Higgins Citation1992, Lucht and Bremer Citation1994). So far, little is known of the regulation of the activity of the protein itself but activation by osmotic stress has been observed (Faatz et al. Citation1988). The proU operon includes three open reading frames (ORFs) proV, proW and proX (Gowrishankar Citation1989, Mimmack et al. Citation1989). The proV gene encodes the NBD with the C-terminal tandem CBS domains. ProW encodes the transmembrane domain (TMD) and has an N-terminal ∼ 100 amino acid extension of unknown function (Haardt and Bremer Citation1996). ProX encodes the substrate-binding protein (SBD), which is localized in the periplasm. ProX has been purified to homogeneity and shown to bind glycine betaine with high affinity (KD of ∼ 1 μM); proline betaine is bound with a KD of ∼ 5 μM (May et al. Citation1986, Barron et al. Citation1987, Haardt et al. Citation1995).
Here, we report on the in vitro reconstitution and biochemical characterization of the osmoregulatory ABC transporter ProU from E. coli. We engineered ProX with an N-terminal lipid anchor sequence for efficient tethering of the substrate-binding protein to the surface of proteoliposomes, in which ProVW was reconstituted first (). We show that the ProU system is activated by osmotic stress and that, similar to OpuA, anionic lipids play an important role in the activity and regulation of transport.
Materials and methods
Bacterial strains and growth conditions
For expression and purification of ProX, L. lactis Opu401 was used as host and transformed with pAMP31proX. Opu401 is a derivative of L. lactis NZ9000 with the chromosomal opuA genes deleted (Biemans-Oldehinkel et al. Citation2006). L. lactis was cultivated semi-aerobically in M17 broth (Difco Laboratories, East Molesey, UK) at 30°C pH 6.5, supplemented with 1% (w/v) glucose plus 5 μg/ml erythromycin, using a 10-l pH- and temperature-controlled bioreactor. Cells were harvested at OD600 of ∼ 5, corresponding to the late exponential phase of growth, washed and resuspended in 50 mM potassium phosphate (KPi) pH 7.0 and stored at −80°C after flash-freezing of 40 ml aliquots of cells in liquid nitrogen.
For expression and purification of ProVW, E. coli strain MC1061 (Casadaban and Cohen Citation1980) was used as host and transformed with pBADcLICproVW. The cells were cultivated under vigorous aeration in Luria broth supplemented with 100 μg/ml ampicillin at 37°C and 200 rpm. At A600 of 0.5–0.6 the temperature was decreased to 25°C, and, after equilibration for 15 min, membrane protein expression was induced by the addition of L-arabinose in growth medium at a final concentration of 0.001% (w/v). Cultivation was continued for 3 h and cells were harvested, washed and resuspended in 50 mM KPi pH 7.0.
Construction of pBADcLICproVW and pNZcLICproVW
The proVW genes were amplified using genomic DNA of E. coli K12 as template and the following oligonucleotides:
ProV fwd nLic: 5′ ATGGTGAGAATTTATATTTTCAAGGTATGGCAATTAAATTAGAAAT
ProW rev nLic 5′ TGGGAGGGTGGGATTT TCA TTACTTAATGAATGGGCGGGTCAG
ProV fwd cLic: 5′ ATGGGTGGTGGATTTGCTATGGCAATTAAATTAGAAAT
ProW rev nLic: 5′ TTGGAAGTATAAATTTTCCTTAATGAATGGGCGGGTC
Both genes were ligated in the pBADnLIC and pBADcLIC vector and modified at the 3′ end to specify a 10-histidine tag, using a ligation-independent cloning method as described in Geertsma and Poolman (Citation2007).
Construction of pAMP31-proX and pAMP31-GGGSAGGGS-proX
The wild-type ProX is produced with a signal sequence for translocation of the protein into the E. coli periplasm. To provide ProX with a lipid anchor, the proX gene was cloned in pAMP31, downstream of the oppA signal sequence and upstream of a sequence specifying a C-terminal six-histidine tag (Picon et al. Citation2000). When expressed in L. lactis, the OppA signal sequence is cleaved at position 23 and the N-terminal Cys residue is lipid modified (Hutchings et al. Citation2009). For this purpose, a unique XhoI site was introduced at position 88 of the oppA gene in pAMP31OppA, which allowed replacing the oppA gene of pAMP31 for proX. The proX gene was amplified by PCR, using genomic DNA of E. coli K12 as template and the oligonucleotides ProX fwd (with XhoI site) and ProX rev (with BamHI) (). The PCR product was digested with XhoI and BamHI and ligated into the vector pAMP31 (cut with XhoI and BamHI). The resulting plasmid was named pAMP31-proX and transformed into L. lactis Opu401 by electroporation.
Table I. Primers used for the construction pAMP31-GGGSAGGGS-proX. Restriction sites are indicated in bold and underlined. Nucleotides different from those in wild-type ProX are indicated in italics.
To increase the space between the lipid anchor and the ProX protein, we introduced a flexible linker (sequence GGGSAGGGS) by inserting the corresponding synthetic DNA fragment into a unique BglII site that was created just after the proX signal sequence. Thus, pAMP31-proX-BglII was digested with XhoI and BglII, and the synthetic DNA with complementary ends was introduced. The synthetic DNA was prepared from complementary oligonucleotides ProX (GGGSAGGGS) fwd and rev; ); the oligonucleotides were annealed by slowly decreasing the temperature from 94–40°C (over 3 min). The resulting plasmid, pAMP31-GGGSAGGGS-proX, was transformed into L. lactis Opu401 by electroporation. Initial experiments showed that the GGGSAGGGS linker is required for substrate transfer from ProX to ProVW, and in the experiments described below pAMP31-GGGSAGGGS-proX was used to produce lipid-anchored ProX ().
Co-transformation with pNZcLICproVW and pAMP31-GGGSAGGGS-proX
L. lactis Opu401 was transformed with pNZcLICproVW and pAMP31-GGGSAGGGS-proX for the simultaneous expression of ProVW and ProX. The mechanism of replication (rolling circle versus theta replication) and antibiotic resistance markers (chloroamphenicol versus erythromycin) of these plasmids differ, which makes them suitable for co-expression studies. 100 ng of each of the plasmid was used for electroporation, after which the cells were plated on GM17 agar, supplemented with 2.5 μg/ml of chloroamphenicol and 2.5 μg/ml erythromycin for plasmid selection.
In vivo uptake experiments
For in vivo glycine betaine uptake, L. lactis Opu401, containing pNZcLICproVW and pAMP31-GGGSAGGGS-proX, was grown in GM17 or GCDM supplemented with 2.5 μg/ml chloroamphenicol plus 2.5 μg/ml erythromycin. At OD600∼ 0.5, nisin A was added at a final concentration of 0.01% (v/v) to induce the expression of proVW. The expression of proX was driven from the constitutive lactococcal promoter P32 (van der Vossen et al. Citation1987). After 2 h of nisin A induction, the cells were washed twice and resuspended to OD600 of ∼ 12.5 (which corresponds to ∼ 2.5 mg/ml protein) in 50 mM HEPES-methylglucamine pH 7.3. For the uptake assays, the cells were diluted to 0.2 mg/ml of total cell protein and pre-energized with 10 mM glucose for 5 min at 30°C. At t = 5 min, 50 mM HEPES-methylglucamine pH 7.3 supplemented with varying amounts of sucrose (0–600 mM) plus [14C]glycine betaine (final concentration of 1 mM; the total reaction mixture was 500 μl) was added, and uptake was monitored over time. To prevent protein synthesis, 25 μg/ml chloroamphenicol plus 25 μg/ml erythromycin was present during the assay. At given time intervals, 80 μl samples were withdrawn and diluted with 2 ml ice-cold assay buffer of equal osmolality and immediately filtered through 0.45 μm cellulose nitrate filters under high vacuum. After washing with 50 mM HEPES-methylglucamine pH 7.3 with the appropriate sucrose concentration, the filters were dried and dissolved in 2 ml scintillation liquid and radioactivity was determined in a scintillation counter.
Membrane vesicle preparation
Membrane vesicles were prepared by lysing the cells in a cell disruption system (high pressure Constant Systems cell disrupters, UK) at a pressure of 39,000 psi for L. lactis and 25,000 psi for E. coli at 4°C in 50 mM potassium phosphate (KPi) with 1 mM MgSO4, 1 mM PMSF, 100 μg/ml DNase plus 100 μg/ml RNase. Subsequently, differential (ultra) centrifugation steps were carried out to separate membrane vesicles from cell debris and cytosolic fractions.
Purification of ProVW
For purification of ProVW, membrane vesicles (10 mg of total protein) of E. coli MC1061/pBADcLICproVW were solubilized with 1% (w/v) dodecyl-β-D-maltoside (DDM) for 45 min at 4°C; the membranes at 5 mg/ml of protein were present in buffer A (50 mM KPi, 200 mM KCl plus 20% glycerol [pH 7.0]) plus 1 mM dithiothreitol (DTT). Following ultracentrifugation at 347,000 g for 15 min, the solubilized material was incubated with Ni-Sepharose (GE Healthcare) (20 mg of protein/ml resin) for 1 h at 4°C, i.e., after the addition of 15 mM imidazole plus 1 mM DTT. Next, the resin was washed with 20 column volumes of buffer A with 0.05% DDM plus 50 mM imidazole. ProVW was eluted in buffer A with 0.05% DDM plus 200 mM imidazole.
Purification of ProX
For purification of lipid-anchored ProX, membrane vesicles of L. lactis Opu401/pAMP31-proX were solubilized with 1% DDM for 45 min in buffer B (25 mM KPi, 100 mM KCl plus 10% glycerol [pH 7.0]) at a final concentration of 5 mg/ml of protein. Following ultracentrifugation at 347,000 g for 15 min, the solubilized material was incubated with Ni-Sepharose (20 mg of protein/ml resin) for 1 h at 4°C in the presence of 15 mM imidazole. Next, the resin was washed with 20 column volumes of buffer A with 0.05% DDM plus 50 mM imidazole. The protein was eluted in buffer B with 0.05% DDM plus 200 mM imidazole. Next, the buffer was exchanged to buffer B with 0.05% DDM plus15 mM imidazole for membrane reconstitution or 100 mM KPi plus 0.05% DDM for fluorescence measurements, using a NAP10 column (GE Healthcare).
Membrane reconstitution of ProVW and surface tethering of lipid-anchored ProX
For membrane reconstitution of ProVW, preformed liposomes composed of E. coli polar lipids and L-α-phosphatidyl choline from egg yolk in a ratio of 3:1 (w/w) or synthetic lipid mixtures composed of dioleoyl-phosphatidylethanolamine (DOPE), dioleoyl-phosphatidylglycerol (DOPG) and dioleoyl-phosphatidylcholine (DOPC), at mole ratios specified in the Figure legends, were used. Liposomes were extruded through a 400 nm pore diameter (400nm pore diameter) polycarbonate filter, and, subsequently, detergent-destabilized by adding increasing amounts of Triton X-100; typically, 70–100 μl of 10% (v/v) of Triton X-100 was used for 2.5 ml of liposomes at a lipid concentration 4 mg/ml (Geertsma et al. Citation2008a). Purified ProVW was incorporated in the Triton X-100-destabilized liposomes at a protein-to-lipid ratio (w/w) of 1:100. Subsequently, Biobeads™ were added in steps to remove the detergent (Geertsma et al. Citation2008a); 1 mM DTT was present in all steps of the purification and membrane reconstitution. For membrane tethering of lipid-anchored ProX, proteoliposomes containing ProVW were extruded through a 400 nm polycarbonate filter, diluted to 4 mg/ml in 50 mM KPi pH 7.0 and mixed with purified ProX at a protein-to-lipid ratio of 1:50 (w/w). Residual detergent was removed by incubation of the (proteo)liposomes with Biobeads. The ATP regenerating system (ARS), composed of 10 mM Mg-ATP, 24 mM phosphocreatine plus 2.4 mg/ml creatine kinase in 50 mM KPI, pH 7.0, was included in the proteoliposomes by two cycles of freezing in liquid nitrogen and thawing at room temperature (Doeven et al. Citation2004, Geertsma et al. Citation2008a) ().
Glycine betaine uptake in proteoliposomes
For ATP-driven uptake of glycine betaine into proteoliposomes, the membranes with ARS inside were extruded and resuspended in 100 mM KPi pH 7.0, and, prior to the start of the assay, they were diluted to a final lipid concentration of 5 mg/ml. The assay buffer was composed of 100 mM KPi pH 7.0 plus 0–300 mM KCl. Following 2 min of equilibration at 30°C, the transport reaction was started by the addition of 40 μM [14C]glycine betaine. At given time intervals, 25 μl samples were withdrawn and diluted with 2 ml ice-cold assay buffer (isotonic with the reaction conditions) and immediately filtered through 0.45 μm cellulose nitrate filter. Following washing of the filter with another 2 ml of ice-cold assay buffer, the radioactivity was determined in a scintillation counter.
Glycine betaine binding
Binding of radiolabeled glycine betaine was measured by the precipitation method (Richarme and Kepes Citation1983), which is based on the principle that, upon salting-out of the protein by ammonium-sulfate, the substrate remains trapped in the ligand-binding site. Binding assays were carried out in a volume of 100 μl with 100 μg/ml of purified ProX in 100 mM KPI, pH 7.0, plus 0.05% (w/v) DDM. Following equilibration for 2 min at 30°C, tracer amounts of [3H]-glycine betaine plus varying amounts of non-labeled glycine betaine were added to the assay mixture, yielding final concentrations of glycine betaine ranging from 0.1–20 μM. The binding reaction was quenched after 2 min by dilution of the sample into 2 ml ice-cold 50% (w/v) ammonium sulfate solution. The mixture was filtered rapidly through 0.45 μm pore-size cellulose nitrate filters. The filters were washed once with 2 ml ammonium sulfate solution. Subsequently, the filters were placed in an open plastic vial and dried overnight at room temperature. The radioactivity on the filters was measured via liquid-scintillation counting, using emulsifier plus scintillation liquid (Perkin Elmer).
Immunodetection
Protein samples were analyzed by 12.5% SDS-PAGE electrophoresis, semi-dry electroblotting and immunodetection with a primary antibody directed against a hexa-His tag (Amersham Pharmacia Biotech). Subsequently, the gels were submitted to Chemiluminescence detection, using the Western-Light kit (Tropix Inc).
Miscellaneous
Radiolabeled [N-methyl-14C] choline chloride (55 mCi/mmol) was obtained from Amersham Biosciences, NJ, USA. The [N-methyl-14C] choline chloride was used as precursor for the synthesis of [N-methyl-14C] glycine betaine as described (Landfald and Strom Citation1986). Radiolabeled [3H]-glycine betaine was prepared via a conversion of [3H]-choline chloride (Amersham, specific activity: 2.01*106 MBq/mmol) to glycine betaine. Creatine kinase and creatine phosphate were obtained from Sigma Chemical Co. All other chemicals were of reagent grade and purchased from commercial sources, unless specified otherwise.
Results and discussion
Cloning and functional expression of proVW and proX
The osmoregulatory ABC transporter ProU is composed of an ATPase subunit ProV, a TMD subunit ProW and the substrate-binding protein ProX (Gowrishankar Citation1989). ProX is located in the periplasm of E. coli, unlike homologues in Gram-positive bacteria or Archaea where the protein is either fused to a TMD or tethered to the membrane via lipid anchor (Gilson et al. Citation1988, Van der Heide and Poolman Citation2000). To unleash the properties and functional characterization of ProU in vitro, we cloned and expressed proVW and proX separately to maximize protein yield. For functional complementation in vivo, the three proteins were expressed simultaneously in a L. lactis strain defective in glycine betaine uptake. The immunoblots of ProX and ProW expressed in L. lactis, grown in GCDM and GM17, are shown in ; ProV is not visible because it lacks a His-tag. shows that the rate of glycine betaine uptake via ProU expressed in L. lactis increases with the extent of osmotic stress, which is similar to what has been observed when the endogenous OpuA transporter is expressed (Biemans-Oldehinkel et al. Citation2006). However, the absolute rates of glycine betaine uptake via OpuA are approximately 5-fold higher than for ProU, which at least in part reflects a higher expression level of the OpuA transporter (data not shown).
Figure 1. Panel A: Schematic representation of the organization of the subunits in ProU (Panel A); the engineered lipid-anchored ProX, the membrane-embedded TMD ProW, and the NBD ProV are shown. Panel B: Schematic of proteoliposomes with ProVW in two orientations, ProX tethered to the external surface, and the ATP-regenerating system (ARS) in the vesicle lumen. Only right-side-out ProVW plus ProX will facilitate the uptake of glycine betaine. This Figure is reproduced in colour in Molecular Membrane Biology online.
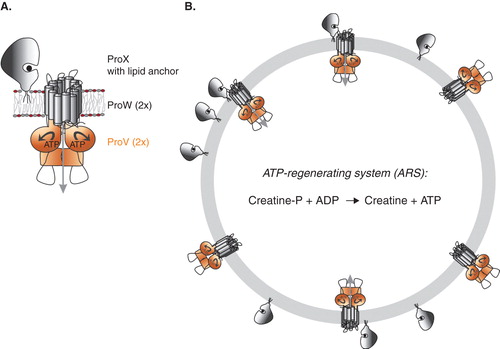
Figure 2. In vivo glycine betaine uptake. Panel A: Expression levels of ProX and ProW in L. lactis Opu401 co-transformed with pNZcLICproVW and pAMP31-GGGSAGGGS-proX and grown in GM17 and GCDM. Panel B: Rate of glycine betaine uptake as a function of sucrose concentration for GCDM- (•) and GM17-grown (○) cells of L. lactis expressing ProVW and ProX. L. lactis Opu401 cells, co-transformed with pNZcLICproVW and pAMP31-GGGSAGGGS -proX, were grown semi-aerobically at 30°C in GM17 and GCDM with 2.5 μg/ml chloroamphenicol plus 2.5 μg/ml erythromycin. Induction with 0.01% (v/v) nisinA was performed for 2 h, prior to harvesting of the cells for the transport assays. Uptake of [14C]glycine betaine (1 mM, final concentration) was assayed in 50 mM HEPES-methylglucamine pH 7.0 in the presence of 10 mM glucose plus 25 μg/ml chloroamphenicol and erythromycin; the final cell concentration in the assay buffer was 0.5 mg/ml. The initial rates of uptake were determined from the linear parts of the progress curves; measurements were done in duplicate. This Figure is reproduced in colour in Molecular Membrane Biology online.
![Figure 2. In vivo glycine betaine uptake. Panel A: Expression levels of ProX and ProW in L. lactis Opu401 co-transformed with pNZcLICproVW and pAMP31-GGGSAGGGS-proX and grown in GM17 and GCDM. Panel B: Rate of glycine betaine uptake as a function of sucrose concentration for GCDM- (•) and GM17-grown (○) cells of L. lactis expressing ProVW and ProX. L. lactis Opu401 cells, co-transformed with pNZcLICproVW and pAMP31-GGGSAGGGS -proX, were grown semi-aerobically at 30°C in GM17 and GCDM with 2.5 μg/ml chloroamphenicol plus 2.5 μg/ml erythromycin. Induction with 0.01% (v/v) nisinA was performed for 2 h, prior to harvesting of the cells for the transport assays. Uptake of [14C]glycine betaine (1 mM, final concentration) was assayed in 50 mM HEPES-methylglucamine pH 7.0 in the presence of 10 mM glucose plus 25 μg/ml chloroamphenicol and erythromycin; the final cell concentration in the assay buffer was 0.5 mg/ml. The initial rates of uptake were determined from the linear parts of the progress curves; measurements were done in duplicate. This Figure is reproduced in colour in Molecular Membrane Biology online.](/cms/asset/2d63c9e9-bc15-4841-86d3-f94765a94a5e/imbc_a_754060_f0002_b.jpg)
To optimize the expression of ProVW in E. coli MC1061/pBADcLICproVW, various inducer (L-arabinose) concentrations, induction times and temperatures were tested. Good expression of ProVW (and avoiding the formation of inclusion bodies; see also Geertsma et al. Citation2008b) was obtained with 0.001% L-arabinose at an induction temperature of 30°C and cells grown in LB medium supplemented with 100 μg/ml ampicillin. L-arabinose was added at an OD600∼ 0.5 and the cells were harvested 3 h later. Inside-out membrane vesicles were isolated as described in Materials and methods, and the expression levels were quantified by immunoblotting, using an anti-Histag antibody. On SDS-PAGE, ProV and ProW display molecular masses around 44 and 37 kDa, respectively (). ProX was expressed in L. lactis with the following modifications: (i) The endogenous signal sequence was replaced for the one from OppA, which allows efficient lipid modification of the N-terminus of the mature protein; (ii) a flexible linker (GGGSAGGGS) was introduced N-terminal to the mature ProX sequence (the first amino acids are indicated below: ADLPGKGITV) and C-terminal to the cleavage site for the signal sequence (underlined) and a short linker (italics) originating from the OppA protein, yielding: MNKLKVTLLASSVVLAATLLSAC GSNQSSS GGGSAGGGSADLPGKGITV as N-terminal sequence; and (iii) a six-histag preceded by a Factor XA cleavage site was engineered at the C-terminus of ProX (added sequence: GSIEGRHHHHHH). As anticipated for lipid-anchored ProX, the protein was found in the membrane fraction of L. lactis cells; the identity of the protein was confirmed by mass spectrometry. The lipid anchor has the advantage that ProX can be tethered to the membrane of liposomes reconstituted with ProVW (). As the interaction of substrate-binding proteins with the translocator subunits of Type I ABC transporters is generally of low affinity Km >50 μM (Prossnitz et al. Citation1989, Dean et al. Citation1992, Doeven et al. Citation2004), membrane tethering offers enormous advantages for the functional reconstitution of the protein complex and monitoring of the transport reaction.
Figure 3. Expression of ProVW and ProX. SDS-PAGE (12% gel) stained with Coomassie brilliant blue. Lane 1: Membrane vesicles of E. coli harboring pBADcLICproVW; lane 2: Purified ProVW; lane 3: Membrane vesicles of L. lactis harboring pAMP31 + GGGSAGGGS + proX; lane 4: Purified ProX; lane 5: proteoliposomes with ProVW; and lane 6: proteoliposomes with ProVW and ProX. This Figure is reproduced in colour in Molecular Membrane Biology online.
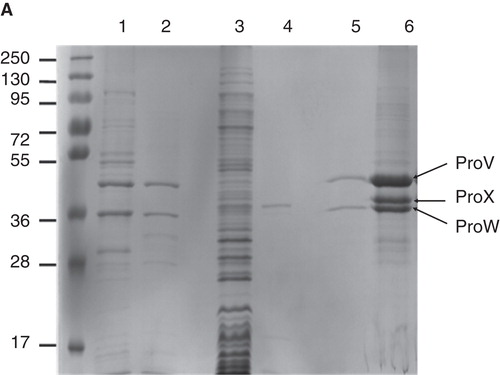
Glycine betaine binding to ProX
Previous studies showed that genuine ProX binds glycine betaine with a KD of ∼ 1 μM (Barron et al. Citation1987, Haardt et al. Citation1995). To test whether or not the lipid anchor has an effect on the binding of glycine betaine by ProX, we used the protein precipitation method of Richarme and Kepes (Citation1983). shows the binding isotherm from which we estimate a KD of 1–2 μM. Thus, we conclude that the lipid anchor and His-tag do not affect the functionality of ProX.
Figure 4. Glycine betaine binding to ProX. Glycine betaine binding to purified ProX with lipid anchor and flexible linker. The lipid-anchored ProX was kept soluble by including of 0.05% (w/v) DDM in the assay buffer (100 mM KPI, pH 7.0). See Materials and methods for further details. This Figure is reproduced in colour in Molecular Membrane Biology online.
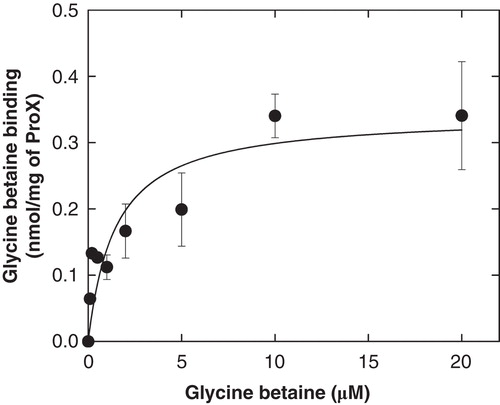
Purification and membrane reconstitution of ProVW-ProX system
For further characterization of the ProU system, large scale fermentations were performed and ProVW and ProX were purified (). As ProV contains 1 cysteine residue in the CBS2 domain and ProW contains 1 cysteine residue in transmembrane helix III, 1 mM of freshly prepared 1 mM DTT was present in each step of membrane vesicle solubilization, protein purification and membrane reconstitution. In fact, ProU lost activity when DTT was omitted in these steps (data not shown). The reconstitution of ProVW and ProX was carried out in three steps: (i) Incorporation of ProVW into liposomes; (ii) membrane-tethering of ProX; and (iii) enclosing in the proteoliposome lumen of an ATP-regenerating system consisting of creatine-phosphate, creatine kinase and Mg-ATP. The procedure is in essence the same as previously described for the OppBCDF-OppA system (Doeven et al. Citation2004) and further specified in (Geertsma et al. Citation2008a), except that in the course of the project, the order of step (ii) and (iii) was reversed. We noted that a higher transport activity, presumably corresponding to a higher reconstitution efficiency, was observed when ProX was tethered to the membrane after the incorporation of the ATP-regenerating system.
Osmotic activation of membrane-reconstituted ProU
Previous studies have shown that the expression of proU is regulated by the osmotic conditions of the medium (Perroudt and Rudulier Citation1985, May et al. Citation1986, Lucht and Bremer Citation1994). Furthermore, there are indications that the activity of ProU is also affected by osmotic stress (e.g., in Faatz et al. Citation1988). To circumstantiate these findings, we determined the regulation of activity of ProU reconstituted in proteoliposomes. To discriminate binding of glycine betaine to surface-tethered ProX from uptake via ProU, we performed a chase experiment with unlabeled glycine betaine. ProX-bound [14C]glycine betaine will be chased upon the addition of an excess of unlabeled glycine betaine, whereas accumulated [14C]glycine betaine will not be chased when the transport reaction is unidirectional. Indeed, a more than 100-fold excess of unlabeled glycine betaine to proteoliposomes that had taken up [14C]glycine betaine for 3 min () did not result in efflux. Also, the presence of external Mg-ATP did not elicit 14C-glycine betaine efflux, demonstrating that inside-out-oriented ProVW (without luminal ProX) does not facilitate transport.
Figure 5. Glycine betaine uptake in proteoliposomes. ProU was reconstituted in liposomes composed of E. coli polar lipids and L-α-phosphatidyl choline from egg yolk in a ratio of 3:1 (w/w); the final ProVW concentration was 0.05 mg/ml. Panel A: [14C]-glycine betaine uptake (40 μM, final concentration) assayed in 100 mM KPi, pH 7.0 plus 300 mM KCl with an ATP-regenerating system enclosed in the vesicle lumen, composed of E. coli polar lipids plus L-α-phosphatidyl choline from egg yolk in a ratio of 3:1 (w/w). Time course of uptake (•); after 3 min of uptake 5 mM Mg-ATP () or 5 mM glycine betaine (○) was added to the assay mixture. Panel B: [14C]-glycine betaine uptake (40 μM, final concentration) assayed in 100 mM KPi, pH 7.0, under iso-osmotic (▵,▴) and hyperosmotic (○,•) conditions (100 mM KPi + 300 mM KCl); with (open symbols) and without (closed symbols) 5 mM glycine betaine in the stop buffer. Panel C: Rate of glycine betaine uptake as a function of KCl concentration, added to 100 mM KPi, pH 7.0 assay buffer. This Figure is reproduced in colour in Molecular Membrane Biology online.
![Figure 5. Glycine betaine uptake in proteoliposomes. ProU was reconstituted in liposomes composed of E. coli polar lipids and L-α-phosphatidyl choline from egg yolk in a ratio of 3:1 (w/w); the final ProVW concentration was 0.05 mg/ml. Panel A: [14C]-glycine betaine uptake (40 μM, final concentration) assayed in 100 mM KPi, pH 7.0 plus 300 mM KCl with an ATP-regenerating system enclosed in the vesicle lumen, composed of E. coli polar lipids plus L-α-phosphatidyl choline from egg yolk in a ratio of 3:1 (w/w). Time course of uptake (•); after 3 min of uptake 5 mM Mg-ATP () or 5 mM glycine betaine (○) was added to the assay mixture. Panel B: [14C]-glycine betaine uptake (40 μM, final concentration) assayed in 100 mM KPi, pH 7.0, under iso-osmotic (▵,▴) and hyperosmotic (○,•) conditions (100 mM KPi + 300 mM KCl); with (open symbols) and without (closed symbols) 5 mM glycine betaine in the stop buffer. Panel C: Rate of glycine betaine uptake as a function of KCl concentration, added to 100 mM KPi, pH 7.0 assay buffer. This Figure is reproduced in colour in Molecular Membrane Biology online.](/cms/asset/c036ce02-4a5a-433a-884b-76d665c12ab5/imbc_a_754060_f0005_b.jpg)
shows that the rate of glycine betaine is stimulated significantly when the external salt concentration is raised by 300 mM KCl; the basal assay medium is composed of 100 mM KPi, pH 7.0. In this experiment, we monitored the uptake reaction in the absence and presence of 5 mM glycine betaine in the stop buffer. In the absence of glycine betaine in the stop buffer, the offset at the y-axis represents the amount of [14C]glycine betaine bound to ProX. The actual uptake (rate) is obtained by subtracting the bound glycine betaine or by including 5 mM glycine betaine in the stop buffer. shows that rapid binding is followed by true transport (accumulation) of [14C]glycine betaine. The rate of uptake increases with the salt concentration and a sigmoidal dependence was observed (), similar to the activation of OpuA (Van der Heide et al. Citation2001).
Effect of anionic lipids on ProU activity
The experiments described heretofore were carried out with ProU reconstituted in liposomes composed of E. coli polar lipids plus L-α-phosphatidyl choline from egg yolk in a ratio of 3:1 (w/w). The fraction of phosphatidylethanolamine in E. coli can be up to 75%; the remaining lipids are mostly anionic, i.e., phosphatidylglycerol (PG) and cardiolipin, typically at 20 and 5 mole% (Cronan Citation2003). The relative amounts of PE, PG and cardiolipin vary with osmotic stress as shown by Romantsov et al. (Citation2009). As osmotic (ionic) regulation of OpuA has been shown to be critically dependent on the fraction of anionic lipids (Biemans-Oldehinkel et al. Citation2006), we reconstituted ProU in 50 mole% of DOPE (zwitterionic, non-bilayer-forming lipid) plus varying ratios of DOPG (anionic, bilayer-forming lipid) and DOPC (zwitterionic, bilayer-forming lipid). shows that ProU activity was not affected by the osmotic stress, i.e., KCl concentration in the range of 0–300 mM in assay buffer composed of 100 mM KPi, pH7.0 (above 300 mM KCl the activity decreased) when DOPG was absent or present at 12 mole%. Importantly, osmotic stress-activated activity was observed at 25 mole% of DOPG and even more so at 38 mole%. The activation was instantaneous, that is, within seconds following the addition of threshold levels of KCl. Although the fraction of anionic lipids in E. coli can vary somewhat (Romantsov et al. Citation2009), such long-term adaptation is probably not relevant for osmoregulation via ProU. Instead, the anionic lipids are needed to make the ionic-gating mechanism of ProU effective. The above findings are reminiscent of what we observed for OpuA from L. lactis (Biemans-Oldehinkel et al. Citation2006), and they suggest that electrostatic interactions between the anionic membrane surface and a protein domain are intrinsic to the osmotic gating of the transporter. Moreover, with 38 mole% of DOPG, maximal ProU activity was observed at 300–400 mM KCl added to the basal assay medium, which is well in the range where the transporter needs to be active in vivo. For instance, the steady state levels of K+ ions increase from 0.15 M to more than 0.5 M when the osmolarity of the medium is increased from 0.1–1.2 Osm (Epstein and Schultz Citation1965, Sutherland et al. Citation1986, Cayley et al. Citation1991).
Figure 6. Effect of anionic lipids on glycine betaine uptake. ProU was reconstituted in liposomes composed of 50 mol% DOPE and 0 (▪), 12 (∇), 25 (), 38 (○), 50 (•) mol% DOPG, plus 50, 38, 25, 12 and 0 mol% DOPC. The ATP-regenerating system was enclosed in the vesicle lumen; the final ProVW concentration in the assay medium was 0.05 mg/ml. The rate of [14C]-glycine betaine uptake (40 μM, final concentration) was assayed in 100 mM KPi, pH 7.0, and different concentrations of KCl. This Figure is reproduced in colour in Molecular Membrane Biology online.
![Figure 6. Effect of anionic lipids on glycine betaine uptake. ProU was reconstituted in liposomes composed of 50 mol% DOPE and 0 (▪), 12 (∇), 25 (), 38 (○), 50 (•) mol% DOPG, plus 50, 38, 25, 12 and 0 mol% DOPC. The ATP-regenerating system was enclosed in the vesicle lumen; the final ProVW concentration in the assay medium was 0.05 mg/ml. The rate of [14C]-glycine betaine uptake (40 μM, final concentration) was assayed in 100 mM KPi, pH 7.0, and different concentrations of KCl. This Figure is reproduced in colour in Molecular Membrane Biology online.](/cms/asset/e489631a-b137-4a10-b9bc-618e99bf17e1/imbc_a_754060_f0006_b.jpg)
Conclusions
In conclusion: The glycine betaine transporter ProU from E. coli was functionally expressed (in L. lactis and E. coli), and the components were purified and reconstituted in proteoliposomes. Lipid-tethering of ProX allowed us to observe glycine betaine uptake in ProVW-containing proteoliposomes. The uptake of glycine betaine in whole cells and in proteoliposomes is regulated by osmotic stress, presumably via an increase in the internal salt concentration as observed for OpuA from L. lactis (ionic strength-gating) and BetP from C. glutamicum (K+-gating). We have not explicitly demonstrated salt-gating of ProU but the parallels with the OpuA system are striking, i.e., for both systems the fraction of anionic lipids is critical for osmoregulated transport, the activity increases with the salt concentration, and both have a CBS module linked to the NBD that has been implicated in osmosensing. The salt dependencies of ProU and OpuA differ somewhat as do the intracellular concentrations of K+ and the fractions (and types) of anionic lipids in the corresponding parent cells, E. coli and L. lactis, respectively. We speculate that the mechanism of osmotic regulation of ProU in E. coli is in essence similar to what has been observed for OpuA in L. lactis. The two transporters have likely evolved to match the physicochemical conditions (internal ionic strength, K+ concentration, water activity, lipid composition) that E. coli and L. lactis face. We are currently exploring the possibility to crystallize the OpuA and ProU proteins to gain further insight in the mechanism of transport and regulation of these ABC transporters.
Acknowledgements
This work was supported by grants from the Netherlands Organisation for Scientific research (NWO, Top-subsidy grant 700.56.302 to BP), the EU (EDICT program). We acknowledge the HEC (higher education commission), Pakistan, for financial support under the HEC overseas scholarship program. We thank Gea Schuurman-Wolters for the construction pBADcLICProVW and pNZcLICProVW, and Alicja Filipowicz-Szymanska for the MS analyses.
Declaration of interest: The authors report no conflicts of interest. The authors alone are responsible for the content and writing of the paper.
References
- Annamalai T, Venkitanarayanan K. 2009. Role of proP and proU in betaine uptake by Yersinia enterocolitica under cold and osmotic stress conditions. Appl Environ Microbiol 75:1471–1477.
- Barron A, Junga JU, Villarejo M. 1987. Purification and characterization of a glycine betaine-binding protein from Escherichia coli. J Biol Chem 262:11841–11846.
- Biemans-Oldehinkel E, Mahmood NABN, Poolman B. 2006. A sensor for intracellular ionic strength. Proc Natl Acad Sci USA 103:10624–10629.
- Booth IR, Higgins CF. 1990. Enteric bacteria and osmotic stress: Intracellular potassium glutamate as a secondary signal of osmotic stress? FEMS Microbiol Rev 6:239–246.
- Cairney J, Booth IR, Higgins CF. 1985. Osmoregulation of gene expression in Salmonella typhimurium: ProU encodes an osmotically induced betaine transport system. J Bacteriol 164:1224–1232.
- Casadaban MJ, Cohen SN. 1980. Analysis of gene control signals by DNA fusion in Escherichia coli. J Mol Biol 138:179–107.
- Cayley S, Lewis BA, Guttman HJ, Record MT. 1991. Characterization of the cytoplasm of Escherichia coli K-12 as a function of external osmolarity. Implications for protein-DNA interactions in vivo. J Mol Biol 222:281–300.
- Chen C, Beattie GA. 2007. Characterization of the osmoprotectant transporter OpuC from Pseudomonas syringae and demonstration that cystathionine-beta-synthase domains are required for its osmoregulatory function. J Bacteriol 189:6901–6912.
- Cronan JE. 2003. Bacterial membrane lipids: Where do we stand? Annu Rev Microbiol 57:203–224.
- Culham DE, Meinecke M, Wood JM. 2012. Impacts of the osmolality and the lumenal ionic strength on osmosensory transporter ProP in proteoliposomes. J Biol Chem 287:27813–27822.
- Dean DA, Hor LI, Shuman HA, Nikaido H. 1992. Interaction between maltose-binding protein and the membrane-associated maltose transporter complex in Escherichia coli. Mol Microbiol 6:2033–2040.
- Doeven MK, Abele R, Tampe R, Poolman B. 2004. The binding specificity of OppA determines the selectivity of the oligopeptide ATP-binding cassette transporter. J Biol Chem 279:32301–32307.
- Epstein W, Schultz SG. 1965. Cation transport in Escherichia coli: V. Regulation of cation content. J Gen Physiol 49:221–234.
- Faatz E, Middendorf A, Bremer E. 1988. Cloned structural genes for the osmotically regulated binding-protein-dependent glycine betaine transport system (ProU) of Escherichia coli K-12. Mol Microbiol 2:265–279.
- Geertsma E, Mahmood NABN, Schuurman-Wolters G, Poolman B. 2008a. Membrane reconstitution of ABC transporters and assays of translocator function. Nature Protoc 3:256–266.
- Geertsma ER, Groeneveld M, Slotboom DJ, Poolman B. 2008b. Quality control of overexpressed membrane proteins. Proc Natl Acad Sci USA 105:5722–5727.
- Geertsma ER, Poolman B. 2007. High-throughput cloning and expression in recalcitrant bacteria. Nature Meth 4:705–707.
- Gilson E, Alloing G, Schmidt T, Claverys JP, Dudler R, Hofnung M. 1988. Evidence for high affinity binding-protein dependent transport systems in gram-positive bacteria and in mycoplasma. EMBO J 7:3971–3974.
- Gowrishankar J. 1989. Nucleotide sequence of the osmoregulatory proU operon of Escherichia coli. J Bacteriol 171:1923–1931.
- Grothe S, Krogsrud RL, Mcclellan DJ, Milner JL, Wood JM. 1986. Proline transport and osmotic stress response in Escherichia coli K-12. J Bacteriol 166:253–259.
- Haardt M, Bremer E. 1996. Use of phoA and lacZ fusions to study the membrane topology of ProW, a component of the osmoregulated ProU transport system of Escherichia coli. J Bacteriol 178:5370–5381.
- Haardt M, Kempf B, Faatz E, Bremer E. 1995. The osmoprotectant proline betaine is a major substrate for the binding-protein-dependent transport system ProU of Escherichia coli K-12. Mol Gen Genet 246:783–786.
- Higgins CF. 1992. ABC transporters: From microorganisms to man. Annu Rev Cell Biol 8. 67–113.
- Horn C, Bremer E, Schmitt L. 2003. Nucleotide dependent monomer/dimer equilibrium of OpuAA, the nucleotide-binding protein of the osmotically regulated ABC transporter OpuA from Bacillus subtilis. J Mol Biol 334:403–419.
- Hutchings MI, Palmer T, Harrington DJ, Sutcliffe IC. 2009. Lipoprotein biogenesis in Gram-positive bacteria: Knowing when to hold 'em, knowing when to fold 'em. Trends Microbiol 17:13–21.
- Karasawa A, Erkens GB, Berntsson RPA, Otten R, Wolters GKS, Mulder FAA, 2011. Cystathionine β-Synthase (CBS) domains 1 and 2 fulfill different roles in ionic strength sensing of the ATP-binding cassette (ABC) transporter OpuA. J Biol Chem 286:37280–37291.
- Kempf B, Bremer E. 1995. OpuA, an osmotically regulated binding protein-dependent transport system for the osmoprotectant glycine betaine in Bacillus subtilis. J Biol Chem 270:16701–16713.
- Kempf B, Gade J, Bremer E. 1997. Lipoprotein from the osmoregulated ABC transport system OpuA of Bacillus subtilis: Purification of the glycine betaine binding protein and characterization of a functional lipidless mutant.. J Bacteriol. 179:6213–6220.
- Landfald B, Strom AR. 1986. Choline-glycine betaine pathway confers a high level of osmotic tolerance in Escherichia coli. J Bacteriol 165:849–855.
- Lucht JM, Bremer E. 1994. Adaptation of Escherichia coli to high osmolarity environments: Osmoregulation of the high-affinity glycine betaine transport system ProU. FEMS Microbiol Rev 14:3–20.
- Mahmood NA, Biemans-Oldehinkel E, Patzlaff JS, Schuurman-Wolters GK, Poolman B. 2006. Ion specificity and ionic strength dependence of the osmoregulatory ABC transporter OpuA. J Biol Chem 281:29830–29839.
- Mahmood NA, Biemans-Oldehinkel E, Poolman B. 2009. Engineering of ion sensing by the cystathionine beta-synthase module of the ABC transporter OpuA. J Biol Chem 284:14368–14376.
- May G, Faatz E, Villarejo M, Bremer E. 1986. Binding protein dependent transport of glycine betaine and its osmotic regulation in Escherichia coli K12. Mol Gen Genet 205:225–233.
- Mimmack ML, Gallagher MP, Pearce SR, Hyde SC, Booth IR, Higgins CF. 1989. Energy coupling to periplasmic binding protein-dependent transport systems: Stoichiometry of ATP hydrolysis during transport in vivo. Proc Natl Acad Sci USA 86:8257–8261.
- Ogahara T, Ohno M, Takayama M, Igarashi K, Kobayashi H. 1995. Accumulation of glutamate by osmotically stressed Escherichia coli is dependent on pH. J Bacteriol 177:5987–5990.
- Perez C, Koshy C, Ressl S, Nicklisch S, Kramer R, Ziegler C. 2011. Substrate specificity and ion coupling in the Na+/betaine symporter BetP. EMBO J 30:1221–1229.
- Perroudt B, Rudulier DL. 1985. Glycine betaine transport in Escherichia coli: Osmotic modulation. J Bacteriol 161:393–301.
- Picon A, Kunji ERS, Lanfermeijer FC, Konings WN, Poolman B. 2000. Specificity mutants of the binding protein of the oligopeptide transport system of Lactococcus lactis. J Bacteriol 182:1600–1608.
- Prossnitz E, Gee A, Ames GF. 1989. Reconstitution of the histidine periplasmic transport system in membrane vesicles. Energy coupling and interaction between the binding protein and the membrane complex. J Biol Chem 9:5006–5014.
- Richarme G, Kepes A. 1983. Study of binding protein-ligand interaction by ammonium sulfate-assisted adsorption on cellulose esters filters. Biochim Biophys Acta 42:16–24.
- Romantsov T, Guan Z, Wood JM. 2009. Cardiolipin and the osmotic stress responses of bacteria. Biochim Biophys Acta 1788:2092–2100.
- Schmidt S, Pfluger K, Kogl S, Spanheimer R, Muller V. 2007. The salt-induced ABC transporter Ota of the methanogenic archaeon Methanosarcina mazei Go1 is a glycine betaine transporter. FEMS Microbiol Lett 277:44–49.
- Sutherland L, Cairney J, Elmore MJ, Booth IR, Higgins CF. 1986. Osmotic regulation of transcription: Induction of the proU betaine transport gene is dependent on accumulation of intracellular potassium. J Bacteriol 168:805–814.
- Van der Heide T, Poolman B. 2000. Osmoregulated ABC-transport system of Lactococcus lactis senses water stress via changes in the physical state of the membrane. Proc Natl Acad Sci USA 97:7102–7106.
- van der Heide T, Stuart MC, Poolman B. 2001. On the osmotic signal and osmosensing mechanism of an ABC transport system for glycine betaine. EMBO J 20:7022–7032.
- Van der Vossen JMBM, Van der Lelie D, Venema G. 1987. Isolation and characterization of Streptococcus cremoris Wg2-specific promoters. Appl Environ Microbiol 53: 2452–2457.
- Wood JM, Bremerb E, Csonkac LN, Kraemerd R, Poolman B, van der Heide T, 2001. Osmosensing and osmoregulatory compatible solute accumulation by bacteria. Comp Biochem Physiol A Mol Integr Physiol 130:437–460.