Abstract
Membrane proteins control fundamental processes that are inherent to nearly all forms of life such as transport of molecules, catalysis, signaling, vesicle fusion, sensing of chemical and physical stimuli from the environment, and cell-cell interactions. Membrane proteins are harbored within a non-equilibrium fluid-like environment of biological membranes that separate cellular and non-cellular environments, as well as in compartmentalized cellular organelles. One of the classes of membrane proteins that will be specifically treated in this article are transport proteins of plant origin, that facilitate material and energy transfer at the membrane boundaries. These proteins import essential nutrients, export cellular metabolites, maintain ionic and osmotic equilibriums and mediate signal transduction. The aim of this article is to report on the progress of membrane protein functional and structural relationships, with a focus on producing stable and functional proteins suitable for structural and biophysical studies. We interlink membrane protein production primarily through wheat-germ cell-free protein synthesis (WG-CFPS) with the growing repertoire of membrane mimicking environments in the form of lipids, surfactants, amphipathic surfactant polymers, liposomes and nanodiscs that keep membrane proteins soluble. It is hoped that the advancements in these fields could increase the number of elucidated structures, in particular those of plant membrane proteins, and contribute to bridging of the gap between structures of soluble and membrane proteins, the latter being comparatively low.
Introduction
A roadmap to functional and structural characterization of membrane proteins continues to be one of the greatest challenges in the current era of structural biology (Stevens Citation2011). This path has led to a definition of function and structure of selected membrane proteins, and in nearly all cases this road has been accompanied by substantial technical innovation. During this process, development of a suite of novel or significantly modified experimental techniques has been required, that allowed characterization of membrane proteins. A series of protein-specific experimental procedures needed to be tailored during molecular cloning, protein synthesis, purification and stabilization, leading to functional membrane proteins. After these steps were established, crystallization and structural investigations typically followed. Three-dimensional (3D) structure elucidations by X-ray or electron crystallography, Nuclear Magnetic Resonance (NMR) spectroscopy and small-angle scattering (SAS) using X-ray or neutron radiation, completed the structural journey (Abad-Zapatero Citation2012). The major advantage of the SAS methods is that they provide structural information on partially or completely ordered systems, as well as those in membrane mimicking environments. Noteworthy, a shape of dimeric mitochondrial cytochrome c oxidase embedded in asolectin liposomes has recently been revealed by small-angle neutron scattering (Rubinson et al. Citation2012). The structural approaches listed above have been applied for countless soluble proteins with relative ease, as evidenced by the plethora of 3D structures deposited in the Protein Data Bank (PDB; http://www.rcsb.org). However, when the procedures developed for soluble proteins are applied to membrane proteins, bottlenecks occurred at virtually each step during functional and structural characterization. For these reasons, the deposition of atomic structures of membrane proteins in PDB has been slow, although during the last three years the momentum has picked up, mostly for prokaryotic membrane proteins.
Despite making up around 30% of all proteins in organisms, membrane proteins with their 342 unique structures (as at July 2012, ), currently represent less than 0.5% of all structures deposited in PDB (http://blanco.biomol.uci.edu/mpstruc, http://www.mpdb.tcd.ie, http://pdbtm.enzim.hu). Compounding this is the fact that of those 342 membrane structures, less than 10 are of a plant origin. demonstrates the inadequacy of availability of membrane versus soluble protein structures deposited in PDB. This shortfall in high-resolution knowledge is a major impediment in addressing the biological functions of plant membrane proteins, i.e., to contribute to understanding of protein function during normal development of plants and during development of plants under biotic or abiotic stresses. We also do not fully understand ligand binding to plant receptors, solute or ion selectivity of plant transporters, protein-protein interactions or catalytic mechanisms of plant proteins involved in synthesis of, e.g., cell-wall polysaccharides, such as cellulose, which is the most abundant biopolymer on earth. As is generally the case of all eukaryotic membrane proteins, a major reason for a low deposition of plant membrane protein structures seems to be in shortcomings that occur during their production and preparation in functional states, where they ought to adopt native and stable folds (Kumar et al. Citation2012). These shortcomings stem from their predominantly hydrophobic nature and interdependence on the surrounding membrane environments (). This relationship that is crucial for stability of membrane proteins in native cells renders expression and purification of membrane proteins in heterologous systems an arduous task. Another, often overlooked piece of the puzzle is maintaining membrane protein function along the path to structure determination. Collectively, these factors contribute to a perceived ‘lack of effort' in the field of plant membrane protein structural determination, although the value of acquiring such knowledge should not be underestimated.
Figure 1. Growth of released 3D structures of soluble (upper panel) and membrane proteins (lower panel) that are classified in the Protein Data Bank and in the Membrane Protein Database, respectively. The data were adapted from PDB (http://www.rcsb.org) and Membrane Protein Data Bank (http://www.mpdb.tcd.ie) statistics. Yearly growth trends are also shown.
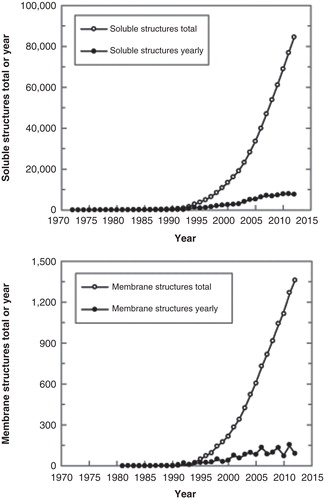
Figure 2. A fluid mosaic model of the cell membrane. Membrane proteins are difficult to produce in the laboratory, because they are often unstable unless bound to lipids. Membrane proteins can be stabilized during CFPS with surfactants and/or lipids (Periasamy et al. Citation2013). The Figure is based on a publicly available image. This Figure is reproduced in colour in Molecular Membrane Biology online.
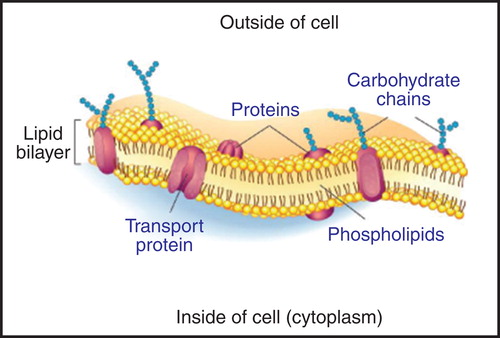
The early in vitro cell-free protein synthesis (CFPS) efforts were focussed on simply understanding translation in the absence of living cells (Nirenberg and Matthaei Citation1961). Later, examples emerged that have used eukaryotic cellular extracts as the CFPS platforms (Spirin et al. Citation1988). In the last decade, CFPS has gathered significant momentum as a valuable tool for preparative-scale synthesis of proteins, affording higher yield, reduced costs and greater reproducibility through automation (Swartz Citation2006). The most commonly used extract for CFPS is derived from bacterial cells, typically of Escherichia coli (Swartz Citation2006, Wuu and Swartz Citation2008, Farrokhi et al. Citation2009). However, owing to the prokaryotic nature of its transcription and translation machinery, these systems are vulnerable to synthesis of incomplete, poorly soluble, incorrectly folded and hence inactive eukaryotic proteins. Of the several extracts available, wheat-germ is better suited to synthesis of plant proteins, most likely due to an analogous tRNA pool, negating the need for codon optimization of the DNA template that encodes the target eukaryotic protein (Endo and Sawasaki Citation2004, Genji et al. Citation2010). Similar to the prokaryotic scenario however, the initial CFPS reactions using wheat-germ extracts suffered from inefficient translation, owing to the presence of an RNA N-glycosidase tritin, ribonucleases and proteases in the wheat endosperm, among other endogenous contaminants (Madin et al. Citation2000). Recently, major inroads have been made to overcome the technical problems of a short-lived translational activity leading to low protein yield, with reports of as high as 14 days of sustained translation yielding milligram protein returns (Sawasaki et al. Citation2002). These developments in CFPS systems led to high-throughput commercial applications (Endo and Sawasaki Citation2004, Tsuboi et al. Citation2008, Rui et al. Citation2011). Several comprehensive reviews outlining the merit of WG-CFPS for production of eukaryotic proteins have been published (Vinarov et al. Citation2006, Takai et al. Citation2008, Ge and Xu Citation2012).
Compared to cell-based expression, all CFPS systems have an upper hand from the point of view of bypassing the energy constraints required for cell survival, and instead channel these resources towards synthesis of a single transcript. The key steps during cell-free synthesis and common evaluation tools of functional and structural properties of synthesized membrane proteins are summarized in . The absence of cells overcomes problems of cytotoxicity, caused by overexpression of high molecular mass proteins and those with multiple hydrophobic membrane-spanning regions. Furthermore, CFPS systems are open in a sense that they readily allow: (i) Operation in batch or continuous exchange (dialysis) modes (Kai et al. Citation2012), (ii) supplementation with a vast array of membrane protein stabilising and activity preserving reagents, in the form of lipids and surfactant-like compounds ( and the section entitled Cell-free protein synthesis and nanotechnology in membrane protein research), and (iii) incorporation of non-natural amino acids such as l-selenomethionine, and stable isotope labelling that facilitate structural determination by X-ray crystallography and NMR spectroscopy, respectively (Kigawa Citation2010). Goren and co-workers (Citation2009) outlined a simple protocol conveying these advantages for bacterial membrane proteins embedded in liposomes. Indeed, most successfully produced membrane proteins are of prokaryotic origin, and despite some exceptions, the sizes of these proteins are well below 80 kDa, or contain fewer than ten membrane-spanning α-helices (Klammt et al. Citation2006). This situation extends to plant membrane proteins, thus a need for a combined ‘therapy' to treat these inadequacies that are a hindrance to the field of plant structural biology, is very much needed.
Figure 3. The key steps during cell-free synthesis of membrane proteins and common tools for evaluation of functional and structural properties of synthesized proteins. The relationship between CFPS and nanotechnology is outlined. The Figure illustrates the two modes of CFPS in batch and continuous exchange modes, and summarizes five main steps during CFPS leading to a functional membrane protein, including optimization of conditions, protein analysis and purification, biophysical, functional and structural characterization. Further description is provided in the relevant sections of the text. This Figure is reproduced in colour in Molecular Membrane Biology online.
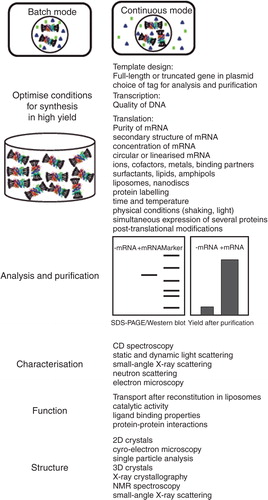
Figure 4. Schematics of assembly of different membrane model systems (a), and detailed views of liposomes (b), tethered bilayers (c) and nanodiscs (d). These environments are suitable for incorporation of membrane proteins from CFPS. The figure is based on Köper et al. (Citation2006), a public image, Braunagel et al. (Citation2011) and Leitz et al. (Citation2006). This Figure is reproduced in colour in Molecular Membrane Biology online.
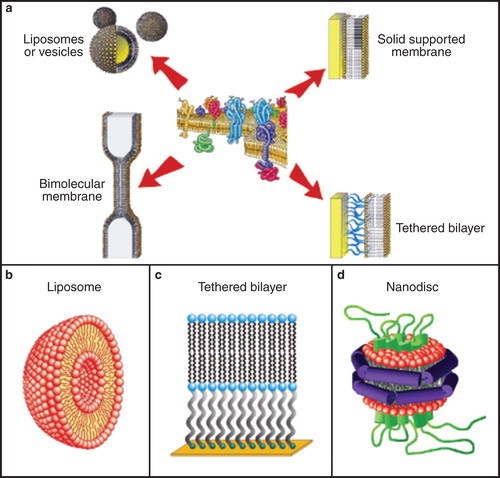
When considering investigation of functional and structural features of membrane proteins, in particular those of transport proteins, nanotechnology and its tools become unavoidably applicable (). The conceptual reason for a very effective merger of these two worlds is the fact that life itself is a nanoscale phenomenon (Mann Citation2008). Nanotechnology as a discipline operates at this level as well, and in reality it is the man-devised experimental research tools of nanotechnology that allow mimicking and modulating biological processes that occur within the cellular membranes. The nanotechnology approaches allow simplifying native environments of membrane proteins, usually by exploiting membrane mimetics (Warschawski et al. Citation2011). Examples of these bilayers include native or artificial unilamellar and multilamellar vesicles, oriented membrane systems, black lipid membranes, solid supported membranes, tethered bilayer membranes, droplet interface bilayers, free standing membranes and nanodiscs, cubosomes, self-assembly lipids, lipid bilayers covalently anchored to carbon nanotubes, as well as bicellar, micellar and reverse micellar, surfactant and amphipol arrangements (Hanke et al. Citation1999 , Köper Citation2007, Sinner et al. Citation2009, Dilanian et al. Citation2011, Conn et al. Citation2011, Früh et al. Citation2011, Warschawski et al. Citation2011, Dayani and Malmstadt Citation2012, Steller et al. Citation2012). The parameters that predispose selections of a specific model membrane system include biological requirements, such as a species- and organelle-specific membrane composition, membrane thickness and curvature that in concert modulate membrane fluidity and stiffness. It is also known that lipid composition can affect topology of a membrane protein, or orientation of its α-helices in a membrane, which underlies membrane protein function (Shinozaki et al. Citation2011). To understand membrane protein requirements for future functional and structural investigations, screening of surfactants and lipids is always a starting challenge to establish whether membrane proteins prepared in heterologous systems can be obtained in soluble, stable and functional states (Warschawski et al. Citation2011). Equally important is to tailor the choice of a model membrane system to the main goal of the study, whether these represent functional assays, e.g., to investigate transport or catalysis with monomeric or oligomerized membrane proteins (Raja Citation2011), or structural basis of membrane protein-protein interactions (Karim et al. Citation2006, Geula et al. Citation2012, Li et al. Citation2012). These studies also aim to optimize lipid environments for structural investigations of membrane proteins, where the goal is to obtain a crystalline material (Guan et al. Citation2006, Kelkar and Chattopadhyay Citation2007, Reichow and Gonen Citation2009, Rajesh et al. Citation2010, Cramer et al. Citation2011, Marassi et al. Citation2011, Stevens Citation2011).
Functional and structural relationships of membrane proteins
Composition of biological membranes and relationship to a nanometer-thin cellular boundary
Phospholipid bilayers at the cellular and extracellular interfaces harbor integral membrane proteins (IMPs) that co-localize between the two opposing environments (). As biological membranes determine integration and distribution of these proteins within their environments, it is logical to assume that a phospholipid bilayer must have transformed into a robust dynamic interface that could sustain the flow of energy and materials occurring between living cells and external environments. This membrane structure embeds the delicate and freely diffusing membrane proteins and serves as a two-dimensional solvent. Because membrane proteins typically inhabit a nanometer-thin cellular boundary, their functional and structural characteristics have developed in concert with both environments. Lipids are tightly integrated into the protein's architecture, where they affect function, structure, quaternary assembly, and stability of membrane proteins (Lee Citation2011). Phospholipids in pro- and eukaryotic organisms are structurally derived from d-glycerol or l-glycerol with ester- or ether-linked saturated and unsaturated diacyl or isoprenoid chains. The properties of both players, i.e., those of lipid and water environments, are chemically and physically sufficient to produce these discrete nano-particles that form bilayered structural platforms of life ubiquitously distributed in Nature (Mann Citation2008).
Phospholipids are biological molecules that typically consist of acyl chains that are around 16–18 carbons in length. These components establish the main constituents of biological membranes. It is remarkable that only up to 1,000 different lipid species occur in natural membranes, although combinatorial permutations of lipid building blocks could theoretically generate thousands of lipid species (Coskun and Simons Citation2011). Other properties of phospholipid components such as chain symmetry, extent of saturation, packing properties, disposition of polar head-groups with bound water molecules as well as sterol content, determine the overall thickness of biological membranes, which is around 5 nm (Bowie Citation2005). The other noteworthy property of biological membranes, in addition to their lateral heterogeneity, is their physical semi-fluidity that is accountable for accommodating membrane proteins and mediating their function (). This non-equilibrium fluid-like environment of membranes, due to the presence of sterols and sphingolipids, also allows formation of membrane or lipid rafts. These raft platforms are thought to be organizing principles of cellular membranes that often entrap membrane proteins, and thus represent the rigid portions of otherwise fluid-like biological membranes (Lauwers and André Citation2006). It has been suggested that lipid rafts play key roles in signal transduction, intracellular sorting, membrane trafficking, cytoskeletal organization, regulation of exocytosis and lipid biosynthesis (Bhat and Panstruga Citation2005, Juhasz et al. Citation2010).
When investigating membrane proteins in vitro, a key to success is to understand the reciprocal relationship between lipid and protein, and the concept of hydrophobic mismatch. This term indicates the extent of variance between the length of the hydrophobic component of membrane protein that spans the biological membrane and the thickness of the lipid bilayer, in which a protein resides. Hydrophobic mismatch can be critical to protein function, as it can induce: (i) Local bilayer stretching (when membrane protein is longer than the thickness of the bilayer), (ii) local bilayer compression (when membrane protein is shorter than the thickness of the bilayer), (iii) tilting of membrane proteins, (iv) membrane protein deformation, and (v) protein aggregation (Parton et al. Citation2011, Hanulová and Weiss Citation2012, Sezgin and Schwille Citation2012). To this end O'Connell and co-workers (Citation2006) showed that BK(Ca) channels forced into phospholipid bilayer mixtures exhibited different gating and conductance following AFM and electrophysiological recordings. These changes can be explained by local changes in lipid-protein interactions between lipid acyl chains and the exterior hydrophobic length of the BK(Ca) channel, leading to retarded thoroughfare of ions through the selectivity filter. Further, Nomura et al. (Citation2012) demonstrated by fluorescence lifetime imaging microscopy that structural dynamics of bacterial mechanoselective channels MscS and MscL clustered in bilayers, depended on the chemical composition of bilayers and the addition of cholesterol that affected their thickness, stiffness and a pressure profile. Ashrafuzzaman and Tuszynski (Citation2012a) suggested that hydrophobic bilayer thickness and channel length mismatch induced an exponential destabilization, while a negative lipid curvature had a linear destabilization effect on a channel.
Molecular structure of plant membrane transport proteins
To illustrate the complexity of plant membrane transport proteins, in this section we describe molecular folds of selected plant transporters (http://blanco.biomol.uci.edu/mpstruc/listAll/list; http://www.mpdb.tcd.ie). Membrane transport proteins fold into two main classes: (i) α-helical structures consisting of α-helices that span membranes at least once. They can exist without external loop regions (e.g., aquaporins; Törnroth-Horsefield et al. Citation2006, Schnurbusch et al. Citation2010) (, panel a) or with loops that protrude from membranes (e.g., HKT transporters, Cotsaftis et al. Citation2012) (, panel b), and (ii) β-barrel structures, which consist of β-strands, e.g., the chloroplast outer envelope membrane proteins (Schleiff et al. Citation2003). The 3D structures of plant β-barrel proteins have not yet been resolved and therefore this type of architecture is illustrated in (panel c) by the bacterial Outer membrane protein A (OmpA) (Pautsch and Schulz Citation2000). A combination of these two main folds, namely of the α-helical and β-barrel folds, produces a novel fold, known as the α-helical barrel (Koronakis et al. Citation2000). This type of structure has also not yet been found in plants, but has been described in bacteria, and is illustrated by the structure of a bacterial efflux pump component (, panel d).
Figure 5. Cartoon representations of molecular folds of plant and bacterial membrane transport proteins. (a) A 3D structure of a spinach aquaporin α-helical bundle structure (PDB ID 1Z98), shown in a tetrameric arrangement (Törnroth-Horsefield et al. Citation2006). Cyan, magenta, yellow and green indicate folds of individual protomers forming a functional tetramer. (b) A 3D model of the rice HKT1;5 transporter showing a complex α-helical structure with extended loops (Cotsaftis et al. Citation2012). (c) A 3D structure of a bacterial membrane domain of OmpA (PDB ID 1QJP) showing its β-barrel fold (Pautsch and Schulz Citation2000). (d) A 3D structure of a bacterial efflux pump component (PDB ID 1EK9) showing an α-helical barrel structure. A trimeric arrangement illustrates a combined fold consisting of the α-helical and β-barrel structural components (Koronakis et al. Citation2000). Green, magenta, and cyan indicate folds of individual protomers forming a functional trimer. Molecular graphics was generated with the PyMol software package (http://www.pymol.org/). This Figure is reproduced in colour in Molecular Membrane Biology online.
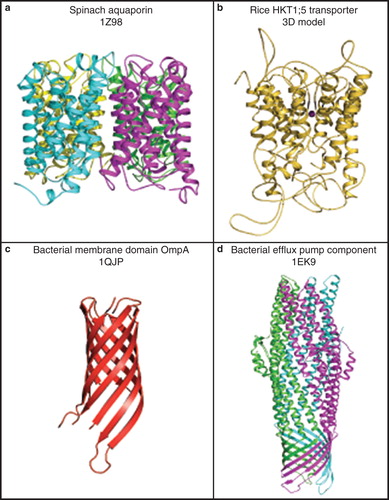
In the following paragraphs we focus on the α-helical architectures with short or protruding loops that have been identified in cereal plants. Both plant membrane transport proteins are intensely investigated in conjunction with abiotic stress tolerance mechanisms, in particular with toxic solute transport such as boric and arsenic acids (Schnurbusch et al. Citation2010), and with Na+ and/or K+ passage (Munns and Tester Citation2008, Cotsaftis et al. Citation2012). The primary interest in the structural studies of these plant membrane transporters is to unravel molecular basis of their solute and ion selectivity. Hence, investigations of transport characteristics of these plant proteins is of great practical significance and can lead to design of improved plants that respond better to toxic or salty conditions. As mentioned above, one approach to understand function of membrane transporters is based on determination of their 3D structures through X-ray (Törnroth-Horsefield et al. Citation2006) or electron (Hite et al. Citation2008) crystallography and SAS (Liu et al. Citation2012), followed by probing function of the key protein residues by mutagenesis. Investigation of other structural properties, such as overall geometry and tilting of α-helices is also of paramount importance (Kelkar and Chattopadhyay Citation2007, Warschawski et al. Citation2011).
The 3D structure of a tetrameric spinach aquaporin (Törnroth-Horsefield et al. Citation2006) allowed molecular modelling of a barley silicon transporter HvNIP2;1, which shows characteristics of a multifunctional aquaporin (Schnurbusch et al. Citation2010). The latter protein is a member of the Nodulin-26-like Intrinsic Protein (NIP) aquaporin family, folds similarly to the spinach form (, panel a) and its 3D model reveals an up-down bundle fold, which houses six membrane helices and two re-entrant helices (Schnurbusch et al. Citation2010). The likely homo-tetrameric organization of HvNIP2;1 could be simulated and it is expected that this higher structural organization could permeate metalloids more effectively. Molecular models of HvNIP2;1 allowed the disposition of interacting amino acid residues to be defined in the pore with respect to the boric, arsenous, silicic, or germanic metalloids. The data from molecular modelling indicated that the four metalloids could be positioned favourably in the HvNIP2;1 pore. Recent progress in the synthesis of bacterial and mammalian aquaporins in a variety of cell-free modes (Hovijitra et al. Citation2009, Kai et al. Citation2010, Xu et al. Citation2010) could pave the way for 3D structure determination of plant aquaporins such as HvNIP2;1, for which currently no 3D structure is available (Schnurbusch et al. Citation2010).
Another example of an α-helical protein with transport function include HKT transporters, also known as HKT ion channels (Munns and Tester Citation2008). These molecules typically consist of 500–560 amino acid residues, and appear to be localized in the plasma membrane of plant cells (Cotsaftis et al. Citation2012). The predicted structure of these proteins shows that, in addition to their α-helical bundle components, the HKT transporters also contain extended loops that protrude beyond the bilayer into the intra- and extra-cytoplasmic space. Currently, there are no resolved atomic structures for any HKT protein in PDB. Consequently, all the structural information available for this class of protein is based on computer predictions. Cotsaftis et al. (Citation2012) have recently predicted eight membrane α-helical motifs in the rice HKT transport protein with both N- and C-termini positioned on the cytoplasmic side of the plasma membrane, and forming a pseudo-tetrameric fold with a central pore (, panel b). The short loops within the central pore form a structural selectivity filter, which underlies transport selectivity. However, this information is uniquely based on structural predictions, including bioinformatics and molecular modelling. Thus, it is imperative to confirm this prognostic information by experimental measurements. Cell-free synthesis approaches promise to enable production of these traditionally difficult-to-express proteins and, coupled with the nanotechnology tools, they could provide a platform to elucidate their structure/function relationships.
Regulation of membrane transport proteins and techniques to determine their function
It has been suggested that membrane transport function is primarily dependent on hydrophobic coupling between the host lipid bilayer and the α-helical membrane-spanning regions of the protein (Ashrafuzzaman and Tuszynski Citation2012b). Changes in this coupling may lead to changes in function due to a transient rearrangement or unfolding of certain parts of transporters (Flores Jiménez and Cafiso Citation2012, Li et al. Citation2012). This is the consequence of changes in bilayer properties, such as membrane curvature, thickness and elastic modulus. Function can be further regulated by the dependence between chemistry of membrane transport proteins and lipids. In particular, annular lipid components maintaining a tight seal around membrane proteins are a key to transport function (Marassi et al. Citation2011). Hite et al. (Citation2008) through electron (2D crystals) and X-ray crystallography (3D crystals) found nine lipids per aquaporin monomer. These lipids formed almost a complete bilayer, and while adopting a range of conformations, they could tightly fill-in space between the adjacent protomers and tetramers. It was remarkable that despite the very different experimental conditions for both structural approaches (electron and X-ray crystallography) the aquaporin structures were virtually identical. In addition to the importance of annular lipids, it has been revealed that additional lipid molecules, e.g., sterols and phosphoinosites could interact with the basic regions of transporters, such as RKH (arginine-lysine-histidine) domains embedded in a membrane, or they could attach to the basic clusters of proteins (Wigoda et al. Citation2010, Lee Citation2011). These biochemical and steric factors require significant considerations, when designing membrane mimetic environments for the study of biomechanical properties of plant transport proteins.
Both in vivo and in vitro techniques are available to investigate the mechanisms and transport functions of membrane proteins. These approaches include traditional and automated patch-clamp techniques (Port-a-Patch or Patchliner instruments), optical (light-scattering) or fluorescent spectroscopy techniques (in normal and stopped-flow modes), fluorescent imaging plate reader assays, electrochemical impedance spectroscopy, refractive index-mediated surface plasmon resonance (SPR) spectroscopy, transistor-based assays using a silicon chip and radiometric approaches with isotopically-labelled substrates. A relevant example that may be broadly applicable to plant membrane proteins includes demonstration that human G protein-coupled receptors, specifically human olfactory receptors, could be synthesized in active forms using WG-CFPS, and that the substrate selectivity and binding could be evaluated by SPR (Kaiser et al. Citation2008). In conjunction with these techniques, an array of biological systems is available, such as whole cell Xenopus laevis oocytes or bilayers of various shapes and configurations that contain reconstituted membrane transport proteins in functional states (Köper Citation2007, Balannik et al. Citation2009 , Brändén et al. Citation2010, Neumann et al. Citation2012, Steller et al. Citation2012). Additionally, single-molecule channel sensors, based on nanopores that sense electrical signals, could be important in real-time detection of molecules transported by plant IMPs. The latter methods have been suggested to be the next generation transport measuring techniques (Gu and Shim Citation2010, Steller et al. Citation2012).
Membrane protein interactomes
To understand the regulation of membrane and other proteins in the context of the membrane and cell-environments, as well as in the context of the cell-cell and intracellular compartments to cytosolic interactions, it will be imperative to determine individual membrane protein interactome hubs in each biological system (Erhardt and Frommer Citation2012). Robotic technologies may play key roles in cataloguing these membrane protein interactomes, most prominently with the use of yeast two-hybrid technologies to map plant and other interactomes (Grefen et al. Citation2007, Lalonde et al. Citation2010, Dreze et al. Citation2011, Erhardt and Frommer Citation2012). Previously, Roman et al. (Citation2006) using SPR spectroscopy demonstrated a selective enrichment of membrane protein binders. With a mitochondrial voltage-dependent anion channel as bait, they found at least 40% of its known ligands and independently confirmed 55 novel functional interactions, some of which unexpectedly fully blocked the channel. Roman et al. (Citation2006) suggested that this highly efficient approach is generally applicable for any membrane or other protein and that it could be automated and scaled up. In a related study, Lalonde et al. (Citation2010) cloned 3,852 open reading frames (ORFs) of Arabidopsis thaliana out of a target list of 8,383, representing membrane and signaling proteins. The mating-based split ubiquitin system was used to screen for potential protein-protein interactions among 490 ORFs in Arabidopsis. A binary robotic screen detected 387 out of 90,370 possible protein-protein interactions. Eighty of 142 membrane-spanning receptor-like kinases tested positive, identifying three homomers, 63 heteromers and 80 protein-protein interactions (Lalonde et al. Citation2010). As for the analysis of the interactome data, these analyses entailed the bioinformatics approaches to identify main signal transduction pathways in the interaction networks, known as signalosomes. It is expected that the key functional signal membrane mediators, also known as hubs, and other abundant regulatory patterns in these networks will be identified in near future. These studies are already underway in Arabidopsis (Loqué et al. Citation2007, Lalonde et al. Citation2008, Erhardt and Frommer Citation2012). Finally, Zhang et al. (Citation2012) applied nanotechnology and mass spectrometry to analyze interactomes of bacterial membrane proteins. Here, the tagged nanodiscs with a reconstituted translocon SecYEG, a maltose transporter MalFGK and a membrane integrase YidC were immobilized, while their loops and other structural elements exposed on each side of the nanodiscs could bind interacting partners from a total bacterial extract. These studies were set up with Stable Isotope Labeling by Amino Acids in Cell Culture-based quantitative proteomics. It is of note that these protein-protein interactions can also be measured by label-free techniques (Baksh et al. Citation2011), such as backscattering interferometry, SPR spectroscopy and isothermal titration calorimetry. The latter approaches allow determination of binding between membrane proteins and their partners in a quantitative manner.
Cell-free protein synthesis and nanotechnology in membrane protein research
In this section we review cell-free protein synthesis (CFPS) and nanotechnology, and highlight how these two technologies can be linked together leading to a new dimension in functional and structural analysis of plant membrane proteins in particular.
Applicability of wheat-germ cell-free protein synthesis (WG-CFPS) for expression of plant membrane proteins
The versatility of extracts derived from wheat embryos for in vitro synthesis of target proteins has seen demand rise sufficiently to justify commercial supply. Although somewhat arduous to prepare, Takai and co-workers (Citation2010) developed a highly stable and active wheat-germ extract that could be prepared through a ‘grind, sieve, wash and extract' protocol. Supplementary components for WG-CFPS can be added to a basic cell-free reaction. These possibilities provide a flexibility to perform WG-CFPS using in-house reagents without a reliance on expensive equipment. Laboratories have become inventive in their quest to streamline reaction conditions and achieve reductions in cost and reagent consumption. Khnouf and co-workers (Citation2011) optimized fabrication of a miniaturized 96-well plate device, to gain a 62-fold increase in luciferase expression through a continuous exchange mode. Recent enhancements such as these will enable a combined realization of high protein yield in a high-throughput mode, which are desired for screening additives to stabilize synthesized membrane proteins for biophysical studies and industrial applications. Later, Nozawa and co-workers (2007) demonstrated WG-CFPS of a chloroplast inner membrane transporter, phosphoenolpyruvate/phosphate translocator 1 from Arabidopsis thaliana. Transport activity was confirmed following co-translational surfactant and lipid screens. In another report, a putative A. thaliana tryptophan/tyrosine permease with 11 membrane α-helices was prepared through WG-CFPS (Sattasuk et al. Citation2011). Sub-organellar localization of the tryptophan/tyrosine permease was assessed by sub-fractionation using a synthesized protein, which penetrated pea seedling chloroplasts, where the permease formed a mature protein and where it integrated into the inner-envelope membrane. The authors note that prior to attempting WG-CFPS, this full length protein could not be prepared. In a related work, a membrane-associated enzyme curdlan synthase (CrdS) from Agrobacterium sp. was synthesized as a full-length protein through WG-CFPS with its catalytic activity preserved (Periasamy et al. Citation2013). Notably, only a catalytic module of CrdS could be expressed in E. coli and not full-length CrdS (Hrmova et al. Citation2010). Although the latter protein is of a bacterial origin, the above-mentioned examples document how the open nature of WG-CFPS permits screening of optimal conditions for production of membrane proteins with functional properties preserved. This useful protein production route for functional analyses under a variety of conditions serves as an early and convenient indicator of protein integrity. These analyses cannot be accomplished through cell-based protein expression approaches. It needs to be emphasized that functional validation, such as that which includes engineering of C-terminal Green Fluorescent Protein (GFP)-linked fusions as reporters of folding states of membrane proteins, should be considered as a prerequisite to allow meaningful conclusions to be drawn for subsequent structural and biophysical studies (Drew et al. Citation2008). A recent study involving both eukaryotic and prokaryotic membrane proteins, including A. thaliana nucleoside transporters AtENT1 and AtENT3 demonstrated the utility of the GFP tagging for this purpose (Müller-Lucks et al. Citation2012).
Membrane mimicking environments conferring membrane protein stability
In the following section we discuss membrane mimicking environments that are directly compatible with WG-CFPS, as well as those environments that have tangible relevance to downstream functional and structural applications of membrane proteins.
Surfactants and surfactant-related molecules
Screening of both prokaryotic and eukaryotic membrane protein production during CFPS most commonly takes place in the presence of surfactants. A wide range is routinely tested for solubilization of synthesized membrane proteins during CFPS. Typically, these surfactants vary from non-ionic species such as n-dodecyl β-d-maltoside, n-decyl β-d-maltoside, Triton X-100, Tween 20 and digitonin, to zwitterionic surfactants such as CHAPS and the Brij range (Klammt et al. Citation2005, Kaiser et al. Citation2008). In the absence of any surfactant or lipid-based environment, membrane proteins aggregate and this mode of synthesis, while often higher yielding may be of limited use if the correct fold and function of the protein cannot be later restored (Isaksson et al. Citation2012). The choice of surfactants needs to be such that they do not impair the translational activity during WG-CFPS. Typically, non-ionic surfactants with a low critical micellar concentration (CMC), i.e., those with a more extended hydrophobic component, are most compatible with cell-free synthesis of membrane proteins. Although significant endeavour is placed into screening of surfactants for cell-free synthesis of membrane proteins, the eventual choice is arrived at empirically. For example Genji et al. (Citation2010) applying the two commonly used surfactants Fos-choline and CHAPS found that these two surfactants counteracted each other's inhibitory effect on the translational activity during WG-CFPS of bacteriorhodopsin. A wide variety of membrane proteins have successfully been synthesized using either E. coli system (Isaksson et al. Citation2012) or WG-CFPS (Nozawa et al. Citation2007), both finding the non-ionic polyoxyethylene surfactant Brij-58 the most successful surfactant additive. A more recent example of application of surfactant-related molecules during CFPS includes hydrophobic peptides, capable of more efficient membrane protein solubilization and higher functional activity, than those synthesized in the presence of traditional surfactants. These molecules have been termed surfactant peptides (Yeh et al. Citation2005, Wang et al. Citation2011) or ‘pepfactants' (Koutsopoulos et al. Citation2012). Wang et al. (Citation2011) reported production of milligram quantities of G-protein coupled receptors, using peptide surfactants in a commercial E. coli CFPS system. In another study, good yields of CrdS were achieved through WG-CFPS with the addition of a series of surfactant peptides (Periasamy et al. Citation2013). As a bonus, CrdS synthesized in the presence of the surfactant peptide Ac-AlaAlaAlaAlaAlaAlaAsp (Ac indicates acetylated; Ala and Asp stand for alanine and aspartic acid, respectively) exhibited catalytic activity, suggesting that CrdS was properly folded. Molecular modelling of membrane proteins should now allow designing surfactants and peptides specifically tailored to individual plant membrane proteins.
Liposomes and other lipid environments
Phospholipid bilayers are conceivably the most biologically relevant environments that are used during WG-CFPS of membrane proteins. One form of phospholipid bilayers are vesicular liposomes that assemble spherically, and in which hydrophobic components are buried, thus their exposure to aqueous environment is minimized (, panel b). As rehydrated lipids spontaneously form multilamellar vesicles of limited practicality, a homogenous distribution of unilamellar liposomes can be obtained by passing a suspension through an extrusion apparatus with a defined pore size. Extruded liposomes are added to the WG-CFPS reactions with reports of between 2.5 and 10 mg/ml, without detriment to membrane protein yield (Periasamy et al. Citation2013). Reports of non-defined liposomes, such as those prepared from acetone-washed asolectin have also been described (Nozawa et al. Citation2011, Periasamy et al. Citation2013). This research revealed that through WG-CFPS expression of AtPPT1 or CrdS, these proteins can be readily co-translationally inserted in liposomes, enriched by density gradient ultra-centrifugation and tested directly for transport or catalytic activities. Phosphocholines such as DMPC (1,2-dimyristoyl-sn-glycero-3-phosphotidylcholine), DOPC (1,2-dioleoyl-sn-glycero-3-phosphocholine) and POPC (1-palmitoyl-2-oleoyl-sn-glycero-3-phosphocholine) are popular lipids used in WG-CFPS due to their chemical stability and low transition temperatures that suit reaction conditions. However, instead of choosing commercially available lipids, it could be useful to screen biologically relevant lipids that occur in native environments of membrane proteins.
Other lipid environments that could be considered appropriate for CFPS include black lipid membranes (Müller and Rudin Citation1967), supported membranes (Sackmann Citation1996) such as hybrid bilayers (Whitesides et al. Citation1991), surface decoupled membranes (Köper Citation2007) like polymer cushioned bilayers (Spinke et al. Citation1992), tethered membranes (Neumann et al. 1999), protein-tethered bilayers (Giess et al. Citation2004) and intact vesicles tethered to a surface (Williams et al. Citation2006) that have previously been reviewed (Köper Citation2007). Some of these lipid environments, e.g., supported membranes are being currently tested using WG-CFPS in our laboratory (Periasamy, Shadiac, Hrmova, unpublished data).
Nanodiscs
The nanodisc technology was developed because protein-surfactant-lipid complexes produced by conventional solubilization often made membrane proteins inactive. In addition, subsequent analyses of membrane proteins have been hampered by poor absorbance and light-scattering properties of protein-surfactant-lipid complexes and their inabilities to form crystalline material. Nanodiscs, originally developed in the laboratory of Sligar and co-workers (Bayburt et al. Citation2002) represent discoidal lipid bilayer patches of a defined diameter that form spontaneously upon removal of surfactants during their preparation. Nanodisc size is pre-determined by the choice of recombinant membrane scaffold protein used during its construction, which functions as an amphipathic belt wrapping the hydrophobic acyl chains of peripheral bilayer lipids. This hydrophobic shielding renders the particles soluble in aqueous solutions. Membrane proteins can be assembled in these nanoparticles following removal of surfactants used during their preparation through dialysis or via adsorption onto hydrophobic beads (Ritchie et al. Citation2009). While nanodiscs are entirely compatible with WG-CFPS, reports of membrane protein insertion into nanodiscs during synthesis are rare (Cappuccio et al. Citation2008). The most successful approach for membrane protein insertion involves reconstitution, following protein preparation and purification in the presence of surfactants (Bayburt and Sligar Citation2010). Contrary to the bounded environment in liposomes, proteins in nanodiscs are accessible from both sides of the bilayer and therefore present a unique opportunity for studying structure, binding and protein-protein interactions of membrane proteins (Borch et al. Citation2011, Glück et al. Citation2011). Examples of plant membrane proteins reconstituted in nanodiscs are rare. One example of this approach is the isolation of trimeric light-harvesting complex (LHCII) from spinach and its reconstitution into asolectin nanodiscs (Pandit et al. Citation2011). The absorbance and fluorescence spectra differed between nanodisc and surfactant-solubilized LHCII, implying differences in conformations of LHCII and their micro-environments. With improvements in nanodisc technology, further examples of embedded membrane proteins extending beyond the well-characterized bacterial integral membrane proteins, such as bacteriorhodopsin and proteorhodpsin, will become possible.
Synthetic surfactant polymers and new generations of surfactants
Although a variety of surfactants are non-inhibitory to translation during WG-CFPS, the presence of surfactants in excess of CMC often leads to synthesis of non-functional membrane proteins as membrane-spanning hydrophobic and other regions become oversaturated by surfactants. In recent years, a new class of synthetic amphiphilic polymers known as ‘amphipols' has emerged (Popot Citation2010, Popot et al. Citation2011).These entities could be applied co-translationally during CFPS or following CFPS to re-fold membrane proteins into their native states. The advantage of using amphipols over nanodiscs for membrane proteins prepared through cell-free synthesis is that a membrane scaffold protein is not required to be present during synthesis, and thus this component will not obscure spectroscopic characterization of a membrane protein (Popot Citation2010).
Klammt and co-workers (2011) performed CFPS of three major families of G-protein coupled receptors in the presence of a chemically modified fructose-based polymer known as NVoy. The TROSY-HSQC NMR spectra revealed stable and folded regions of receptors with ligand-binding specificity of these receptors retained. These observations highlighted the applicability of the NVoy surfactants for cell-free synthesis, although, certain amphiphiles such as A8-35 and sulfonated amphipols are inhibitory to WG-CFPS (Periasamy et al. Citation2013). The reason for this inhibitory behaviour of amphipols is not fully understood and could result from either functional de-stabilization of ribosomes contained in wheat germ, or that amphipols directly interfere with the structure of ribosomes. It has also been suggested that styrene maleic acid, A8-35 and sulfonated amphipols may interact with basic patches on surfaces of ribosomal nanoparticles (Bazzacco et al. Citation2012). In such cases, these amphipoles are better suited for reconstitution of membrane proteins, following their synthesis in the presence of another stabilising agent. Finally, surfactant-free nanosized lipid-based polymer-stabilized particles, known as ‘lipodisq', have been prepared from conventional lipids and amphipols, such as styrene maleic acid copolymer (Orwick et al. Citation2012). After lipodisq particles are assembled, they can accommodate membrane proteins. The resultant proteo-lipodisq particles are investigated by electron paramagnetic resonance (EPR), circular dichroism (CD) and NMR spectroscopy. Their obvious advantage is that proteo-lipodisq particles do not contain a membrane scaffold protein and that surfactants are not required for their preparation. In conclusion, Zhang et al. (Citation2011) suggested that design of synthetic amphiphilic polymeric surfactants will continue, as new generations of these compounds could shrink the sizes of protein-surfactant micelles, balance hydrophobicity and hydrophilicity of membrane proteins, and prove useful for the bilayer-based crystallization approaches.
Incorporation of membrane proteins into nanoparticles in functional states
While the consensus is that the suitability of a given surfactant is protein-specific, often, polymeric surfactants such as the Brij series are apparent choices for initial testing of membrane protein yields (e.g., Nozawa et al. Citation2007). However, these surfactants interact tightly with membrane proteins and this makes their removal for future functional and structural characterization problematic. Removal or exchange for lipids is commonly needed, as surfactants often interfere with transport assays or crystallization trials. This occurs most commonly through dialysis or adsorption. For surfactants with high CMCs, such as n-octyl β-d-glucoside, dialysis can be a successful removal strategy. On the other hand, those with low CMCs, such as Brij-58 cannot diffuse through the pores of dialysis membranes readily, since most of their micelles are too large. An alternative technique of removal of organic surfactants from aqueous solutions is adsorption on neutral and macroporous polymeric bio-beads (Periasamy et al. Citation2013). Once the membrane proteins are re-assembled with suitable surfactants or lipids, they can be reconstituted in liposomes and nanodiscs in functional states or tested in crystallization trials.
Unlike many other classes of surfactants, polymeric surfactant substitutes such as NVoy (Klammt et al. Citation2011) and styrene maleic acid copolymer (Knowles et al. Citation2009) have been reported to be compatible with mass spectrometry, and CD and NMR spectroscopy, which allowed size and shape properties of target membrane proteins to be investigated. Here, Knowles et al. (Citation2009) integrated α-helical bundle and β-barrel membrane proteins in the styrene maleic acid copolymer/dimyristoyl phosphatidylcholine particles and showed formation of discrete dispersed discoidal particles with membrane proteins.
One of the inexpensive sources of plant-derived lipids that can be used for synthesis of functional plant membrane proteins in cell-free systems, or reconstituted in nanoparticles, is a soybean asolectin lipid extract. This lipid extract represents a complex mixture of phospholipids such as lecithin, cephalin and phosphatidylinositol in equal proportions, along with minor amounts of other phospholipids and polar lipids. This mixture is purified from a crude soybean source, so it is likely to also contain sterols, sphingolipids, other lipid species and even small amounts of proteins. One drawback of the asolectin lipid extracts is their undefined composition that could lead to irreproducibility of data obtained from biophysical measurements. Although, this can be remedied by simulating the composition of a soybean asolectin lipid extract, as the individual components are commercially available. It is notable that the soybean asolectin lipid extract has been used for 2D membrane protein crystallization (Barrera et al. Citation2005).
Reconstitution of membrane proteins is closely related to membrane protein topology in the individual nanoparticles. Thus, membrane topology becomes of paramount importance to analyse structural aspects of proteo-liposomes. Neves and co-workers (Citation2009) demonstrated that both lipid type and surfactant removal following proteo-liposome purification could impact upon the directional orientation of a membrane protein reconstituted in liposomes, as measured by fluorescence anisotropy. To this end, WG-CFPS synthesis allows precise lipid conditions to be screened, while simultaneously overcoming the need for a surfactant. In addition to the lipid composition and hydrophobic mismatch considerations described earlier, another factor for correctly oriented and functional proteo-liposomes relates to the curvature of the spheres, defined by the pore size of the extrusion filter. Two useful methods to overcome this include: (i) Use of extrusion filters with pore sizes several hundred nanometers in diameter to generate Large Unilamellar Vesicles (LUV), and (ii) formation of Giant Unilamellar Vesicles (GUV), for example through electroformation (Varnier et al. Citation2010). Proteo-liposomes made through the latter technique can range from 1–100 μm in diameter. The biological relevance of these particles allows meaningful conclusions to be drawn from functional studies of plant transport and other proteins (Kreir et al. Citation2008, Kusters et al. Citation2011).
Outlook and perspectives
Over a period where the linear assemblies of millions of genes have been defined, structures of more than 83,000 soluble proteins are deposited in PDB, but only 1027 (342 unique) membrane proteins in the Membrane Protein Database are available. From this simple comparison it is evident that conceptual and technological challenges associated with determination of 3D structures of membrane proteins are enormous. As these challenges are being conquered by way of applying tunable synchrotron radiation for small and weakly diffracting crystals that membrane proteins often form (Abad-Zapatero Citation2012), membrane protein crystallography benefits also from high-throughput methods for crystallization of membrane proteins and semi-automated methods for solving their structures (Abad-Zapatero Citation2007, Citation2012). Nevertheless, one serious bottleneck still remains. Due to the fragile natures of membranes and difficulties associated with isolating membrane proteins from their native environments, molecular studies of membrane proteins are often based on heterologous production of membrane proteins (Farrokhi et al. Citation2009, Hrmova and Fincher Citation2009). This conclusion is fully applicable to plant membrane proteins that also occur in only minute absolute quantities in plants. While cell-based expression systems based on yeast, such as Pichia pastoris will continue to play a significant role in eukaryotic membrane protein expression, the cell-free alternative based on wheat-germ provides an opportunity for synthesis of toxic and fastidious membrane proteins. Considering that structural studies depend on sample quality, the main goal during CFPS and subsequent purification should be retention of function, particularly with a decreasing dependence on large sample volumes that are needed for crystallization (Bill et al. Citation2011).
At a slightly higher level, 3D structural information of membrane proteins will enable the molecular details of their partner protein interactions to be defined. Important cellular processes such as signal transduction, the assembly of multi-protein transcription complexes or the activation of membrane transporters might all involve interactions between two or more proteins (Li et al. Citation2012). Structural biology technologies for membrane proteins are problematical but not undefeatable. Nevertheless, in the future, it will be necessary to develop more robust methods and strategies or modify existing ones, particularly for plant membrane protein expression and crystallization. The prerequisite for structural determinations is the ability to produce sufficiently pure and properly folded proteins in low milligram quantities for crystallization. Both in vivo and in vitro methodologies are available for membrane protein production and crystallization (Bill et al. Citation2011, Periasamy et al. Citation2013), and in particular crystallization approaches will constantly continue developing (Cherezov Citation2011, Conn et al. Citation2012, Darmanin et al. Citation2012, Eddy et al. Citation2012). Membrane proteins that are refractory to X-ray diffraction could be approached by 2D crystallization followed by electron crystallography, although only a handful of the membrane protein structures has been determined so far (http://blanco.biomol.uci.edu/mpstruc/listAll/list). Presently, these approaches, in combination with SAS and electron crystallography, represent the viable roadmaps to structure determination of membrane proteins (Hite et al. Citation2008, Reichow and Gonen Citation2009, Dilanian et al. 2011).
Due to the recent developments in nanotechnology and due to its application to function and structure of plant and other membrane proteins, we could predict that the future has arrived for one particular tactic. This approach involves linking CFPS and nanotechnology that ultimately would facilitate structural investigations and lead to atomistic descriptions. For example, detailed studies may aim to elucidate structures of open and closed conformations of plant channels and transporters embedded in nanodiscs, and taking into account specific composition of plant membranes under physiologically relevant conditions.
However, membrane protein functional and activation trajectories could not be unravelled from crystal structures determined by X-ray or electron crystallography alone, as crystal structures typically represent the time-spaced averaged spatial representations of a given protein. The information about regulation of function of membrane proteins will be derived from combined studies with NMR spectroscopy (Yi et al. Citation2009) and by molecular dynamics simulations, which ironically depend on availabilities of atomic structures, in the absence or presence of cognate ligands and other protein partners (Sansom et al. Citation2008, Treptow and Klein Citation2012, Wu et al. Citation2012). In these studies with transporters it is revealed that the membrane α-helices bend, kink, rotate and modify their interactions leading to formation of pores, which are the structural determinants responsible for transport properties of channels (Yi et al. Citation2009). Progressive techniques, such as time-resolved wide angle X-ray scattering, permit real-time structural dynamics of proteins to be elucidated at high resolution (Andersson et al. Citation2009). Through subtractive analysis, conformational states of proteins during transport can be determined over a nanosecond time course. It is believed that these approaches offer promising tools to reveal fully atomic structures of the active and resting states of transport proteins. This information will allow us to understand how these proteins are regulated by large family of cognate ligands and other allosteric molecules (Treptow and Klein Citation2012). It is encouraging that in recent years, molecular dynamics calculations are increasingly combined with experimental approaches. Here, researchers assess properties and stability of membrane proteins such as melting (Mancusso et al. Citation2011), using light scattering or scanning fluorimetry (Senisterra et al. Citation2010), EPR spectroscopy (Flores Jiménez and Cafiso Citation2012), and fluorescence energy transfer and CD spectroscopy (Li et al. Citation2012) combined with mutagenesis (Wu et al. Citation2012). Most of these techniques also assist in making conclusions about protein stability that are fundamental to protein crystallography.
In conclusion, important inroads have been made in membrane protein functional and structural investigations. The precise definition of plant membrane protein function and the roles of other proteins regulating this function will continue to be a research priority. It is guaranteed that this research will be of an inter-disciplinary nature coupling both experimental and theoretical data that can be jointly used to develop testable hypotheses. The advent of the nanotechnology era and its linking to CFPS will undoubtedly deliver novel membrane protein compatible nano-tools. We project that this trend should lead to an increase of elucidated plant membrane protein structures at atomic levels.
Acknowledgements
This work was supported by the grants from the Australian Research Council and the Grains Research & Development Corporation, by the South Australian Government, and by the DP120100900 and LP120100201 grants from the Australian Research Council awarded to MH. We are grateful to the members of the Structural Biology Group at the Australian Centre for Plant Functional Genomics of the University of Adelaide, who over the years have contributed to WG-CFPS and nanotechnology.
Declaration of interest: The authors report no conflicts of interest. The authors alone are responsible for the content and writing of the paper.
References
- Abad-Zapatero C. 2007. Notes on protein crystallography: quo vadis structural biology? Acta Cryst D 54:687–689.
- Abad-Zapatero C. 2012. Notes of a protein crystallographer: On the high-resolution structure of the PDB growth rate. Acta Cryst D 68:613–617.
- Andersson M, Malmerberg E, Westenhoff S, Katona G, Cammarata M, Wöhri AB, 2009. Structural dynamics of light-driven proton pumps. Structure 17:1265–1275.
- Ashrafuzzaman M, Tuszynski J. 2012a. Regulation of channel function due to coupling with a lipid bilayer. J Comput Theoret Nanosci 9:564–570.
- Ashrafuzzaman M, Tuszynski J. 2012b. Ion pore formation in lipid bilayers and related energetic considerations. Curr Med Chem 19:1619–1634.
- Baksh MM, Kussrow AK, Mileni M, Finn MG. 2011. Label-free quantification of membrane-ligand interactions using backscattering interferometry. Nat Biotech 29:357–360.
- Balannik V, Obrdlik P, Inayat S, Steensen C, Wang J, Rausch JM, 2009. Solid-supported membrane technology for the investigation of the influenza A virus M2 channel activity. Pflugers Arch 459:593–605.
- Barrera FN, Renart ML, Molina ML, Poveda JA, Encinar JA, Fernández AM, 2005. Unfolding and refolding in vitro of a tetrameric, α-helical membrane protein: The prokaryotic potassium channel KcsA. Biochemistry 44:14344–14352.
- Bayburt TH, Sligar SG. 2010. Membrane protein assembly into nanodiscs. FEBS Lett 584:1721–1727.
- Bayburt TH, Grinkova YV, Sligar SG. 2002. Self-assembly of discoidal phospholipid bilayer nanoparticles with membrane scaffold proteins. Nanoletters 2:853–856.
- Bazzacco P, Billon-Denis E, Sharma KS, Catoire LJ, Mary S, Le Bon C, 2012. Nonionic homopolymeric amphipols: Application to membrane protein folding, cell-free synthesis, and solution nuclear magnetic resonance. Biochemistry 51:1416–1430.
- Bhat RA, Panstruga R. 2005. Lipid rafts in plants. Planta 223:5–19.
- Bill RM, Henderson PJF, Iwata S, Kunji ERS, Michel H, Neutze R, 2011. Overcoming barriers to membrane protein structure determination. Nat Biotechnol 29:335–340.
- Borch J, Roepstorff P, Møller-Jensen J. 2011. Nanodisc-based co-immunoprecipitation for mass spectrometric identification of membrane-interacting proteins. Mol Cell Proteomics 10:O110.
- Bowie J. 2005. Solving the membrane protein folding problem. Nature 438:581–589.
- Brändén M, Tabaei SR, Fischer G, Neutze R, Höök F. 2010. Refractive-index-based screening of membrane-protein-mediated transfer across biological membranes. Biophys J 99:124–133.
- Braunagel J, Junghans A, Köper I. 2011. Membrane-based sensing approaches. Aust J Chem 64:54–61.
- Cappuccio JA, Blanchette CD, Sulchek TA, Arroyo ES, Kralj JM, Hinz AK, 2008. Cell-free co-expression of functional membrane proteins and apolipoprotein, forming soluble nanolipoprotein particles. Mol Cell Proteomics 7:2246–2253.
- Cherezov V. 2011. Lipidic cubic phase technologies for membrane protein structural studies. Curr Opin Struct Biol 21:559–566.
- Conn CE, Mulet X, Moghaddam MJ, Darmanin C, Waddington LJ, Sagnella SM, 2011. Enhanced uptake of an integral membrane protein, the dopamine D2L receptor, by cubic nanostructured lipid nanoparticles doped with Ni(II) chelated EDTA amphiphiles. Soft Matter 7:567–578.
- Conn CE, Darmanin C, Mulet X, Le Cann S, Kirby N, Drummond CJ. 2012. High-throughput analysis of the structural evolution of the monoolein cubic phase in situ under crystallogenesis conditions. Soft Matter 8:2310–2321.
- Cotsaftis O, Plett D, Shirley N, Tester M, Hrmova M. 2012. A two-staged model of Na+ exclusion in rice explained by 3D modeling of HKT transporters and alternative splicing. PLoS One 7:e39865.
- Coskun U, Simons K. 2011. Cell membranes: The lipid perspective. Structure 19:1543–1548.
- Cramer WA, Zakharov SD, Saif Hasan S, Zhang H, Baniulis D, Zhalnina MV, 2011. Membrane proteins in four acts: Function precedes structure determination. Methods 55:415–420.
- Dayani Y, Malmstadt N. 2012. Lipid bilayers covalently anchored to carbon nanotubes. Langmuir 28:8174–8182.
- Darmanin C, Conn CE, Newman J, Mulet X, Seabrook SA, Liang YL, 2012. High-throughput production and structural characterization of libraries of self-assembly lipidic cubic phase materials. ACS Comb Sci 14:247–252.
- Dilanian RA, Darmanin C, Varghese JN, Wilkins SW, Oka T, Yagi N, 2011. A new approach for structure analysis of two-dimensional membrane protein crystals using X-ray powder diffraction data. Protein Sci 20:457–464.
- Drew D, Newstead S, Sonoda Y, Kim H, von Heijne G, Iwata S. 2008. GFP-based optimization scheme for the overexpression and purification of eukaryotic membrane proteins in Saccharomyces cerevisiae. Nat Protein 3:784–798.
- Dreze M, Carvunis AR, Charloteaux B, Galli M, Pevzner SJ, Tasan M, 2011. Evidence for network evolution in an Arabidopsis interactome map. Science 333:601–607.
- Eddy MT, Ong TC, Clark L, Teijido O, van der Wel PC, Garces R, 2012. Lipid dynamics and protein-lipid interactions in 2D crystals formed with the β-barrel integral membrane protein VDAC1. J Am Chem Soc 134:6375–6387.
- Endo Y, Sawasaki T. 2004. High-throughput, genome-scale protein production method based on the wheat germ cell-free expression system. J Struct Funct Genomics 5:45–57.
- Erhardt DW, Frommer WB. 2012. New technologies for 21st century plant science. Plant Cell 24:374–394.
- Farrokhi N, Hrmova M, Burton RA, Fincher GB. 2009. Heterologous and cell-free protein expression systems. In: Somers D, Langridge P, Gustafson P, editors. Plant genomics. Humana Press Inc. Totowa, USA: Methods in Molecular Biology series. 513:175–198.
- Flores Jiménez RH, Cafiso DS. 2012. The N-terminal domain of a TonB-dependent transporter undergoes a reversible stepwise denaturation. Biochemistry 51:3642–3650.
- Früh V, Ijzerman AP, Siegal G. 2011. How to catch a membrane protein in action: A review of functional membrane protein immobilization strategies and their applications. Chem Rev 111:640–656.
- Ge X, Xu J. 2012. Cell-free protein synthesis as a promising expression system for recombinant proteins. Methods Mol Biol 824:565–578.
- Genji T, Nozawa A, Tozawa Y. 2010. Efficient production and purification of functional bacteriorhodopsin with a wheat-germ cell-free system and a combination of Fos-choline and CHAPS detergents. Biochem Biophys Res Commun 400:638–642.
- Geula S, Ben-Hail D, Barmatz V. 2012. Structure-based analysis of VDAC1: N-terminus location, translocation, channel gating and association with anti-apoptotic proteins. Biochem J 444:475–485.
- Giess F, Friedrich MG, Heberle J, Naumann R, Knoll W. 2004. The protein-tethered lipid bilayer: A novel mimic of the biological membrane. Biophys J 87:3213–3220.
- Glück JM, Koenig BW, Willbold D. 2011. Nanodiscs allow the use of integral membrane proteins as analytes in surface plasmon resonance studies. Anal Biochem 408:46–52.
- Goren MA, Nozawa A, Makino S, Wrobel RL, Fox BG. 2009. Cell-free translation of integral membrane proteins into unilamelar liposomes. Meth Enzymol 463:647–673.
- Grefen C, Lalonde S, Obrdlik P. 2007. Split-ubiquitin system for identifying protein-protein interactions in membrane and full-length proteins. Curr Prot Neurosci 5; Unit 5.27.
- Gu L-Q, Shim JW. 2010. Single molecule sensing by nanopores and nanopore devices. Analyst 135:441–451.
- Guan L, Smirnova IN, Verner G, Nagamoni S, Kaback HR. 2006. Manipulating phospholipids for crystallization of a membrane transport protein. Proc Natl Acad Sci USA 103:1723–1726.
- Hanke G, Bowsher C, Jones MN, Tetlow I, Emes M. 1999. Proteoliposomes and plant transport proteins. J Exp Bot 50:1715–1726.
- Hanulová M, Weiss M. 2012. Membrane-mediated interactions – a physico-chemical basis for protein sorting. Mol Membr Biol 29:177–185.
- Hite RK, Gonen T, Harrison SC, Walz T. 2008. Interactions of lipids with aquaporin-0 and other membrane proteins. Pflugers Arch 456:651–661.
- Hovijitra NT, Wuu JJ, Peaker B, Swartz JR. 2009. Cell-free synthesis of functional aquaporin Z in synthetic liposomes. Biotechnol Bioeng 104:40–49.
- Hrmova M, Fincher GB. 2009. Functional genomics and structural biology in the definition of gene function. In: Somers D, Langridge P, Gustafson P, editors. Plant genomics. Humana Press Inc. Totowa, USA: Methods in Molecular Biology series. 513:199–127.
- Hrmova M, Stone BA, Fincher GB. 2010. High-yield production, refolding and molecular modelling of the catalytic module of (1,3)-β-d-glucan (curdlan) synthase from Agrobacterium sp. Glycocon J 27:461–476.
- Isaksson L, Enberg J, Neutze R, Göran Karlsson B, Pedersen A. 2012. Expression screening of membrane proteins with cell-free protein synthesis. Protein Expr Purif 82:218–225.
- Juhasz J, Davis JH, Sharom FJ. 2010. Fluorescent probe partitioning in giant unilamellar vesicles of ‘lipid raft' mixtures. Biochem J 430:415–423.
- Kai L, Kaldenhoff R, Lian J, Zhu X, Dötsch V, Bernhard F, 2010. Preparative scale production of functional mouse aquaporin 4 using different cell-free expression modes. PLoS One 5:e12972.
- Kai L, Roos C, Haberstock S, Proverbio D, Ma Y, Junge F, 2012. Systems for the cell-free synthesis of proteins. Methods Mol Biol 800:201–225.
- Kaiser L, Graveland-Bikker J, Steuerwald D, Vanberghem M, Herlihy K, Zhang S. 2008. Efficient cell-free production of olfactory receptors: Detergent optimization, structure, and ligand binding analyses. Proc Natl Acad Sci USA 105:15726–15731.
- Karim CB, Zhang Z, Howard EC, Torgersen KD, Thomas DD. 2006. Phosphorylation-dependent conformational switch in spin-labeled phospholamban bound to SERCA. J Mol Biol 358:1032–1040.
- Kelkar DA, Chattopadhyay A. 2007. The gramicidin ion channel: A model membrane protein. Biochim Biophys Acta 1768:2011–2025.
- Khnouf R, Chapman BD, Fan ZH. 2011. Fabrication optimization of a miniaturized array device for cell-free protein synthesis. Electrophoresis 32:3101–3107.
- Kigawa T. 2010. Cell-free protein production system with the E. coli crude extract for determination of protein folds. In: Endo Y, Ueda T, Takai K, editors. Cell-free protein production: Methods and protocols. Totowa. USA: Humana Press Inc. Methods in Molecular Biology series. 607:101–111.
- Klammt C, Schwarz D, Fendler K, Haase W, Dötsch V, Bernhard F. 2005. Evaluation of detergents for the soluble expression of alphahelical and beta-barrel-type integral membrane proteins by a preparative scale individual cell-free expression system. FEBS J 272:6024–6038.
- Klammt C, Schwarz D, Löhr F, Schneider B, Dötsch V, Bernhard F. 2006. Cell-free expression as an emerging technique for the large scale production of integral membrane protein. FEBS J 273:4141–4153.
- Klammt C, Perrin MH, Maslennikov I, Renault L, Krupa M, Kwiatkowski W, 2011. Polymer-based cell-free expression of ligand-binding family B G-protein coupled receptors without detergents. Protein Sci 20:1030–1041.
- Knowles TJ, Finka R, Smith C, Lin Y-P, Dafforn T, Overduin M. 2009. Membrane proteins solubilized intact in lipid containing nanoparticles bounded by styrene maleic acid copolymer. J Am Chem Soc 131:7484–7485.
- Koronakis A, Sharff A, Koraonakis E, Luisi B, Hugues C. 2000. Crystal structure of the bacterial membrane protein TolC central to multidrug efflux and protein export. Nature 405:914–919.
- Koutsopoulos S, Kaiser L, Eriksson HM, Zhang S. 2012. Designer peptide surfactants stabilize diverse functional membrane proteins. Chem Soc Rev 41:1721–1728.
- Köper I, Schiller S, Giess F, Naumann R, Knoll W. 2006. Functional tethered bimolecular lipid membranes (tBLMs). In: Leitmannova Liu A, editor. Advances in planar lipid bilayers and liposomes. The Netherlands: Elsevier. Vol. 3:37–53.
- Köper I. 2007. Insulated tethered bilayer lipid membranes to study membrane proteins. Mol Biosyst 3:651–657.
- Kreir M, Farre C, Beckler M, George M, Fertig N. 2008. Rapid screening of membrane protein activity: Electrophysiological analysis of OmpF reconstituted in proteoliposomes. Lab Chip 8:587–595.
- Kumar A, Ward P, Katre UV, Mohanty S. 2012. A novel and simple method of production and biophysical characterization of a mini-membrane protein, Ost4p: A subunit of yeast oligosaccharyl transferase. Biopolymers 97:499–507.
- Kusters I, Mukherjee N, de Jong MR, Tans S, Koçer A, Driessen AJ. 2011. Taming membranes: Functional immobilization of biological membranes in hydrogels. PLoS One 6:e20435.
- Lalonde S, Rhee S, Loqué D, Ehrhardt DW, Frommer WB. 2008. Molecular and cellular approaches for the detection of protein-protein interactions: Latest techniques and current limitations. Plant J 53:610–635.
- Lalonde S, Sero A, Pratelli R, Pilot G, Chen J, Sardi MI, 2010. A membrane protein/signaling protein interaction network for Arabidopsis version AMPv2. Front Physiol 1:24–24/14.
- Lee AG. 2011. Biological membranes: The importance of molecular detail. Trends Biochem Sci 36:493–500.
- Leitz AJ, Bayburt TH, Barnakov AN, Springer BA, Sligar SG. 2006. Functional reconstitution of β2-adrenergic receptors utilizing self-assembling Nanodisc technology. BioTechniques 40:601–610.
- Lauwers E, André B. 2006. Association of yeast transporters with detergent-resistant membranes correlates with their cell-surface location. Traffic 7:1045–1059.
- Thomas Li J, James ZM, Dong X, Karim CBDD. 2012. Structural and functional dynamics of an integral membrane protein complex modulated by lipid headgroup charge. J Mol Biol 418:379–389.
- Liu H, Hexemer A, Zwart PH. 2012. The small angle scattering toolbox (SASTBX): An open-source software for biomolecular small-angle scattering. J Appl Cryst 45:587–593.
- Loqué D, Lalonde S, Looger L, von Wirén N, Frommer WF. 2007. Trans-activation and cooperativity in an ammonium transporter complex. Nature 446:195–198.
- Madin K, Sawasaki T, Ogasawara T, Endo Y. 2000. A highly efficient and robust cell-free protein synthesis system prepared from wheat embryos: Plants apparently contain a suicide system directed at ribosomes. Proc Natl Acad Sci USA 97:559–564.
- Mancusso R, Karpowich NK, Czyzewski BK, Wang DN. 2011. Simple screening method for improving membrane protein thermostability. Methods 55:324–329.
- Mann S. 2008. Life as a nanoscale phenomenon. Angew Chem Int Ed 47:5306–5320.
- Marassi FM, Das BB, Lu GJ, Nothnagel HJ, Park SH, Son WS, 2011. Structure determination of membrane proteins in five easy pieces. Methods 55:363–369.
- Munns R, Tester M. 2008. Mechanisms of salinity tolerance. Ann Rev Plant Biol 59:651–681.
- Müller-Lucks A, Bock S, Wu B, Beitz E. 2012. Fluorescent in situ folding control for rapid optimization of cell-free membrane protein synthesis. PLoS ONE 7:e42186.
- Müller P, Rudin DO. 1967. Action potential phenomena in experimental bimolecular lipid membranes. Nature 213:603–604.
- Neumann J, Hennig M, Wixforth A, Rädler J, Schneider MF. 2012. Transport, separation and accumulation of proteins on supported lipid bilayers. Nano Lett 10:2903–2908.
- Neves P, Lopes SC, Sousa I, Garcia S, Eaton P, Gameiro P. 2009. Characterization of membrane protein reconstitution in LUVs of different lipid composition by fluorescence anisotropy. J Pharm Biomed Anal 49:276–281.
- Nirenberg MW, Matthaei JH. 1961. The dependence of cell-free protein synthesis in E. coli upon naturally occurring or synthetic polyribonucleotides. Proc Natl Acad Sci USA 4:1588–1602.
- Nomura T, Cranfield CG, Deplazes E, Owen D, Macmillan A, Battle AR, 2012. Differential effects of lipids and lyso-lipids on the mechanosensitivity of the mechanosensitive channels MscL and MscS. Proc Natl Acad Sci USA 109:8770–8775.
- Nozawa A, Nanamiya H, Miyata T, Linka N, Endo Y, Weber AP, 2007. A cell-free translation and proteoliposome reconstitution system for functional analysis of plant solute transporters. Plant Cell Physiol 48:1815–2180.
- Nozawa A, Ogasawara T, Matsunaga S, Iwasaki T, Sawasaki T, Endo Y. 2011. Production and partial purification of membrane proteins using a liposome-supplemented wheat cell-free translation system. BMC Biotechnol 11:35.
- O'Connell RJ, Yuan C, Johnston LJ, Rinco O, Probodh I, Treistman SN. 2006. Gating and conductance changes in BK(Ca) channels in bilayers are reciprocal. J Membr Biol 213:143–153.
- Orwick MC, Judge PJ, Procek J, Lindholm L, Graziadei A, Engel A, 2012. Detergent-free formation and physicochemical characterization of nanosized lipid-polymer complexes: lipodisq. Angew Chem Int Ed 51:4653–4657.
- Pandit A, Shirzad-Wasei N, Wlodarczyk LM, van Roon H, Boekema EJ, Dekker JP, 2011. Assembly of the major light-harvesting complex II in lipid nanodiscs. Biophys J 101:2507–2515.
- Parton DL, Klingelhoefer JW, Sansom MSP. 2011. Aggregation of model membrane proteins, modulated by hydrophobic mismatch, membrane curvature, and protein class. Biophys J 101:691–699.
- Pautsch A, Schulz GE. 2000. High-resolution structure of the OmpA membrane domain. J Mol Biol 298:273–282.
- Periasamy A, Shadiac N, Amalraj A, Garajová S, Nagarajan Y, Waters S, Mertens HD, Hrmova M. 2013. Cell-free protein synthesis of membrane (1,3)-β-D-glucan (curdlan) synthase: Co-translational insertion in liposomes and reconstitution in nanodiscs. Biochimica et Biophysica Acta - Biomembranes 1828:743–757.
- Popot JL. 2010. Amphipols, nanodiscs, and fluorinated surfactants: Three nonconventional approaches to studying membrane proteins in aqueous solutions. Annu Rev Biochem 79:737–775.
- Popot JL, Althoff T, Bagnard D, Banères JL, Bazzacco P, Billon-Denis E, 2011. Amphipols from A to Z. Annu Rev Biophys 4:379–408.
- Raja M. 2011. The potassium channel KcsA: A model protein in studying membrane protein oligomerization and stability of oligomeric assembly? Arch Biochem Biophys 510:1–10.
- Rajesh S, Knowles T, Overduin M. 2010. Production of membrane proteins without cells or detergents. New Biotech 28:250–254.
- Reichow SL, Gonen T. 2009. Lipid-protein interactions probed by electron crystallography. Curr Opin Struct Biol 19:560–565.
- Ritchie TK, Grinkova YV, Bayburt TH, Denisov IG, Zolnerciks JK, Atkins WM, 2009. Chapter 11 – Reconstitution of membrane proteins in phospholipid bilayer nanodiscs. Methods Enzymol 464:211–231.
- Roman I, Figys J, Steurs G, Zizi M. 2006. Hunting interactomes of a membrane protein: Obtaining the largest set of voltage-dependent anion channel-interacting protein epitopes. Mol Cell Proteomics 5:1667–1680.
- Rubinson KA, Pokalsky C, Krueger S, Prochaska LJ. 2012. Structure determination of functional membrane proteins using small-angle neutron scattering (SANS) with small, mixed-lipid liposomes: Native beef heart mitochondrial cytochrome c oxidase forms dimers. Protein J. DOI 10.1007/s10930-012-9455-0, 1–12.
- Rui E, Fernandez-Becerra C, Takeo S, Sanz S, Lacerda MV, Tsuboi T, 2011. Plasmodium vivax: Comparison of immunogenicity among proteins expressed in the cell-free systems of Escherichia coli and wheat germ by suspension array assays. Malar J 10:192.
- Sackmann E. 1996. Supported membranes: scientific and practical applications. Science 271:43–48.
- Sansom MSP, Scott KA, Bond PJ. 2008. Coarse-grained simulation: A high-throughput computational approach to membrane proteins. Biochem Soc Trans 36:27–32.
- Sattasuk K, Nozawa A, Tozawa Y, Kakinuma Y, Akita M. 2011. In vitro protein import of a putative amino acid transporter from Arabidopsis thaliana into chloroplasts and its suborganellar localization. Biosci Biotechnol Biochem 75:2200–2206.
- Sawasaki T, Ogasawara T, Morishita R, Endo Y. 2002. A cell-free protein synthesis system for high-throughput proteomics. Proc Natl Acad Sci USA 99:14652–14657.
- Schnurbusch T, Hayes J, Hrmova M, Baumann U, Ramesh SA, Tyerman SD, 2010. Boron toxicity tolerance in barley through reduced expression of the multifunctional aquaporin HvNIP2;1. Plant Physiol 153:1706–1715.
- Schleiff E, Eichacker LA, Eckart K, Becker T, Mirus O, Stahl T, 2003. Prediction of the plant beta-barrel proteome: A case study of the chloroplast outer envelope. Protein Sci 12:748–759.
- Senisterra GA, Ghanei H, Khutoreskaya G, Dobrovetsky E, Edwards AM, Privé GG, 2010. Assessing the stability of membrane proteins to detect ligand binding using differential static light scattering. J Biomol Screen 15:314–320.
- Sezgin E, Schwille P. 2012. Model membrane platforms to study protein-membrane interactions. Mol Membr Biol 29:144–154.
- Shinozaki Y, Sumitomo K, Tanaka A, Kasai N, Torimitsu K. 2011. Examination of ion channel protein orientation in supported lipid bilayers. Appl Phys Express 4:107001–107003.
- Sinner EK, Ritz S, Naumann R, Schiller S, Knoll W. 2009. Self-assembled tethered bimolecular lipid membranes. Adv Clin Chem 49:159–179.
- Spinke J, Yang J, Wolf H, Liley M, Ringsdorf H, Knoll W. 1992. Polymer-supported bilayer on a solid substrate. Biophys J 63:1667–1671.
- Spirin AS, Baranov VI, Ryabova LA, Ovodov SY, Alakhov YB. 1988. A continuous cell-free translation system capable of producing polypeptides in high yield. Science 242:1162–1164.
- Steller L, Kreir M, Salzer R. 2012. Natural and artificial ion channels for biosensing platforms. Anal Bioanal Chem 402:25–28.
- Stevens RC. 2011. A roadmap to membrane protein structures. Methods 55:271–271.
- Swartz J. 2006. Developing cell-free biology for industrial applications. J Ind Microbiol Biotechnol 33:476–485.
- Takai K, Sawasaki T, Endo Y. 2010. Practical cell-free protein synthesis system using purified wheat embryos. Nat Protoc 5:227–238.
- Takai K, Sawasaki T, Endo Y. 2008. Chapter 2. Development of key technologies for high-throughput cell-free protein production with the extract from wheat embryos. Adv Protein Chem Struct Biol 75:53–84.
- Törnroth-Horsefield S, Wang Y, Hedfalk K, Johanson U, Karlsson M, Tajkhorshid E, 2006. Structural mechanism of plant aquaporin gating. Nature 439:688–694.
- Treptow W, Klein ML. 2012. Computer simulations of voltage-gated cation channels. J Phys Chem Lett 29:1017–1023.
- Tsuboi T, Takeo S, Iriko H, Jin L, Tsuchimochi M, Matsuda S, 2008. Wheat germ cell-free system-based production of malaria proteins for discovery of novel vaccine candidates. Infect Immun 76:1702–1708.
- Varnier A, Kermarrec F, Blesneac I, Moreau C, Liguori L, Lenormand JL, 2010. A simple method for the reconstitution of membrane proteins into giant unilamellar vesicles. J Membr Biol 233:85–92.
- Vinarov DA, Loushin Newman CL, Markley JL. 2006. Wheat germ cell-free platform for eukaryotic protein production. FEBS J 273:4160–4169.
- Wang X, Corin K, Baaske P, Wienken CJ, Jerabek-Willemsen M, Duhr S, 2011. Peptide surfactants for cell-free production of functional G protein-coupled receptors. Proc Natl Acad Sci USA 108:9049–9054.
- Warschawski DE, Arnold AA, Beaugrand M, Gravel A, Chartrand É, Marcotte I. 2011. Choosing membrane mimetics for NMR structural studies of transmembrane proteins. Biochim Biophys Acta 1808:1957–1974.
- Whitesides GM, Mathias JP, Seto CT. 1991. Molecular self-assembly and nanochemistry: A chemical strategy for the synthesis of nanostructures Science. 254:1312–1319.
- Wigoda N, Ma X, Moran N. 2010. Phosphatidylinositol 4,5-bisphosphate regulates plant K(+) channels. Biochem Soc Trans 38:705–709.
- Williams T, Vareiro MMLM, Jenkins ATA. 2006. Fluorophore-encapsulated solid-supported bilayer vesicles: A method for studying membrane permeation processes. Langmuir 22:6473–6476.
- Wu HH, Chen CC, Chen CM. 2012. Replica exchange Monte-Carlo simulations of helix bundle membrane proteins: Rotational parameters of helices. J Comput Aided Mol Des 26:363–374.
- Wuu JJ, Swartz JR. 2008. High yield cell-free production of integral membrane proteins without refolding or detergents. Biochim Biophys Acta 1778:1237–1250.
- Xu Z, Lian J, Cai J. 2010. Efficient expression of aquaporin Z in Escherichia coli cell-free system using different fusion vectors. Protein Pept Lett 17:181–185.
- Yeh JI, Du S, Tortajada A, Paulo J, Zhang S. 2005. Peptergents: Peptide detergents that improve stability and functionality of a membrane protein, glycerol-3-phosphate dehydrogenase. Biochemistry 44:16912–16919.
- Yi M, Cross T, Zhou HX. 2009. Conformational heterogeneity of the M2 proton channel and structural model for channel activation Proc Natl Acad Sci USA. 106:13311–13316.
- Zhang Q, Tao H, Hong WX. 2011. New amphiphiles for membrane protein structural biology. Methods 55:318–323.
- Zhang XX, Chan CS, Bao H, Fang Y, Foster LJ, Duong F. 2012. Nanodiscs and SILAC-based mass spectrometry to identify a membrane protein interactome. J Proteome Res 11:1454–1459.