Abstract
The effects of glucocorticoid on lipid metabolism of broiler chicken (Gallus gallus domesticus) skeletal muscle were investigated. Male Arbor Acres chickens (35 days old) were subjected to dexamethasone treatment for 3 days. We found that dexamethasone retards body growth while facilitating lipid accumulation. In M. pectoralis major (PM), dexamethasone increased the expression of glucocorticoid receptor (GR), fatty acid transport protein 1 (FATP1), heart fatty acid-binding protein (H-FABP) and long-chain acyl-CoA dehydrogenase (LCAD) mRNA and decreased the expression of liver carnitine palmitoyltransferase 1 (L-CPT1), adenosine-monophosphate-activated protein kinase (AMPK) α2 and lipoprotein lipase (LPL) mRNA. LPL activity was also decreased. In M. biceps femoris (BF), the levels of GR, FATP1 and L-CPT1 mRNA were increased. AMPKα (Thr172) phosphorylation and CTP1 activity of skeletal muscle were decreased by dexamethasone. In fed chickens, dexamethasone enhanced very low-density lipoprotein receptor (VLDLR) expression and AMPK activity in muscle, but it impaired the expression of LPL and L-CPT1 mRNA and LPL activity in PM and augmented the expression of GR, LPL, H-FABP, L-CPT1, LCAD and AMPKα2 mRNA in BF. Adipose triglyceride lipase (ATGL) protein expression was not affected by dexamethasone. In conclusion, in the fasting state, dexamethasone-induced-retarded fatty acid utilisation may be involved in the augmented intramyocellular lipid accumulation in both glycolytic (PM) and oxidative (BF) muscle tissues. In the fed state, dexamethasone promoted the transcriptional activity of genes related to lipid uptake and oxidation in muscles. Unmatched lipid uptake and utilisation are suggested to be involved in the augmented intramyocellular lipid accumulation.
Introduction
Lipids are a major fuel source for oxidative metabolism, especially in cardiac and skeletal muscles (Coppack et al. Citation1994), and abnormal lipid metabolism underlies the metabolic syndrome. Several functional and structural steps are involved in lipid uptake, transport and oxidation in muscle. Intracellular lipid partitioning towards storage and the incomplete oxidation of fatty acids are linked to diminished insulin sensitivity (Kelley and Mandarino Citation2000; Kelley and Goodpaster Citation2001; de Beaudrap et al. Citation2006; Corcoran et al. Citation2007). Lipid-induced mitochondrial overload combined with incomplete oxidation results in insulin resistance in skeletal muscle (Hancock et al. Citation2008; Koves et al. Citation2008).
Many metabolic abnormalities, including dyslipidaemia and insulin resistance, are characterised by elevated levels of glucocorticoids (Andrews and Walker Citation1999), suggesting that glucocorticoids play an important role in lipid metabolism. Glucocorticoids, as the final effectors of the hypothalamic–pituitary–adrenal axis, participate in the maintenance of whole body homoeostasis and evoke a series of essential physiological responses related to the mobilisation of energy stores and the redistribution of energy towards inhibiting growth (Matteri et al. Citation2000; Post et al. Citation2003). In mammals, glucocorticoids may promote lipid accumulation in muscle cells by influencing the lipid uptake and utilisation. Glucocorticoids play an important role in the development of obesity (Freedman et al. Citation1986), induce increased lipid transport in gastrocnemius (Komamura et al. Citation2003) and facilitate lipid oxidation in the diaphragm muscle of rats (Venkatesan et al. Citation1996).
Many regulating factors and pathways are involved in the utilisation of fatty acids by muscle cells. These factors include fatty acid transport protein 1 (FATP1) and heart fatty acid-binding protein (H-FABP), which are important for transport (Veerkamp and Van Moerkerk Citation1993; Maatman et al. Citation1994; Van Nieuwenhoven et al. Citation1995), and carnitine palmitoyltransferase 1 (CPT1) and long-chain acyl-CoA dehydrogenase (LCAD), which play roles in mitochondrial β-oxidation (McGarry et al. Citation1989; Izai et al. Citation1992; McGarry and Brown Citation1997; Eaton Citation2002). Adipose triglyceride lipase (ATGL) is identified as a triglyceride-specific hydrolase in myotubes and skeletal muscle (Haemmerle et al. Citation2006; Watt et al. Citation2008). AMP-activated protein kinase (AMPK), a key indicator of the physiological AMP to adenosine-triphosphate (ATP) ratio, catalyses the phosphorylation of acetyl-CoA carboxylase, leading to a decrease in the concentration of malonyl-CoA. The activation of AMPK results in the stimulation of fatty acid uptake and oxidation in muscle. In mammals, a decrease in AMPK and an increase in malonyl-CoA are linked to insulin resistance (Ye et al. Citation2005). Excess lipid accumulation in skeletal muscle in obesity could be due to dysregulation of the AMPK/malonyl-CoA fuel-sensing system (Ruderman and Saha Citation2006).
The modern broiler chicken has several characteristics (rapid growth rate, high muscle yield and high feed efficiency) that make it an interesting model for muscle development (Halevy et al. Citation2000). Compared with mammals of similar body weight, birds have higher levels of glucose and lower concentrations of insulin (Dupont et al. Citation2004; Braun and Sweazea Citation2008). Moreover, the insulin cascade in chickens appears to be more refractory than that in mammals (Dupont et al. Citation2004, Citation2008), and corticosterone administration induces insulin resistance in chickens (Taouis et al. Citation1993; Dupont et al. Citation1999). In broiler chickens, dexamethasone enhances de novo hepatic lipogenesis while simultaneously retarding the development of skeletal muscle, providing evidence that glucocorticoids cause a redistribution of energy towards fat deposition (Jiang et al. Citation2008; Yuan et al. Citation2008; Cai et al. Citation2009). We have shown in a previous study (Wang et al. Citation2010) that dexamethasone administration promotes intramyocellular lipid accumulation by suppressing lipid oxidation in broiler chicks during their initial growth period (10 days of age), and suggested that the AMPK pathway may be involved in the regulation by glucocorticoids of fatty acid utilisation (Wang et al. Citation2010). In broiler chickens, the maximum body weight and muscle growth rates are achieved at approximately 35 days of age (Scheuermann et al. Citation2003), while fat deposition increases with age (Zerehdaran et al. Citation2004). We thus hypothesised that glucocorticoids may alter intramyocellular lipid accumulation by regulating the fatty acid uptake and utilisation in broiler chickens during the period of maximum muscle growth.
In this study, two different muscle types, oxidative and glycolytic, were investigated. Dexamethasone, which exhibits a high affinity for glucocorticoid receptors (GRs) and a delayed plasma clearance (Foucaud et al. Citation1998), was employed to induce a hyperglucocorticoid milieu. The investigation was done during both feeding and fasting to evaluate the effect of glucocorticoids in the presence or absence of dietary fatty acid. To avoid the possible influence of differing feed intake caused by the glucocorticoid treatment (Lin et al. Citation2004), a pair-fed group (with feed amounts based on the food intake of the dexamethasone-treated chickens) was used.
Materials and methods
Birds and husbandry
Male broiler chicks (Arbor Acres, Gallus gallus domesticus) were obtained from a local hatchery at 1 day of age and reared in an environmentally controlled room. The brooding temperature was maintained at 35°C (65% relative humidity) for the first 2 days, then decreased gradually to 21°C (45% relative humidity) until day 28 and thereafter maintained as such until the end of the experiment (day 38). The light regime was 23 h light: 1 h dark; the dark period was from 20:00 to 21:00 h. All chicks received a starter diet with 21.5% crude protein and 12.37 MJ/kg of metabolisable energy until day 21, after which they received a grower diet with 19.5% crude protein and 12.90 MJ/kg of metabolisable energy (Zhao et al. Citation2009a). All the birds had free access to feed and water during the rearing period. The study was approved by the University and performed in accordance with the ‘Guidelines for Experimental Animal’ of the Ministry of Science and Technology (Beijing, China).
Treatments
Trial 1: Dexamethasone and terminal 12-h fasting
In Trial 1, 60 broilers were randomly divided into 6 pens of 10 birds according to body weight. Beginning on day 35, three pens of chickens were given subcutaneous injections of dexamethasone (2 mg/kg body weight per day according to our previous trial) while the other three pens were subjected to sham treatment (injection of 0.9% saline, vehicle control). Chickens were given injections twice per day at 06:00 and 18:00 h. After 3 days of treatment, eight chickens with a mean body weight of 1715 g were randomly selected from each treatment group and killed by exsanguination after cervical dislocation (Close et al. Citation1997) at 06:00 h on day 38 after 12 h with no feeding. Muscle samples of 3–4 g of both the left M. pectoralis major (PM, fast-twitch glycolytic fibre type muscle) and the left M. biceps femoris (BF, slow-twitch oxidative fibre type muscle) were obtained, snap-frozen in liquid nitrogen and stored at − 70°C for histological analysis, CPT1 activity determination and Western blotting.
Trial 2: Dexamethasone, pair-feeding and terminal 12-h fasting or feeding
In Trial 2, 180 broilers were divided into 9 pens of 20 chickens according to body weight. Beginning on day 35, chickens were randomly subjected to one of the following three treatments (twice per day at 06:00 and 18:00 h) for 3 days: subcutaneous injection of dexamethasone (2 mg/kg body weight per day); sham treatment (injection of 0.9% saline, vehicle control); and pair-fed sham treatment with the same amount of feed provided as that consumed by the dexamethasone-treated chickens during the previous day (Urdaneta-Rincon and Leeson Citation2002). Body weight was recorded daily.
Before samples were obtained, half of the chickens from each pen were randomly assigned to a feeding or a fasting group for 12 h. At 06:00 h on day 38, eight chickens in each treatment group (body weight, mean 1780 g) were selected and killed by exsanguination after cervical dislocation (Close et al. Citation1997). Abdominal fat, cervical and thigh subcutaneous fat and breast and thigh muscle were harvested and weighed. Muscle samples of 1–2 g were obtained from the left PM and BF, snap-frozen in liquid nitrogen and stored at − 70°C for further analysis.
Measurements
For the measurement of total fat content in skeletal muscle, homogenised muscle tissue was mixed with a chloroform/methanol solution (2:1, v/v). The chloroform phase was removed and reduced by evaporation at 40°C under nitrogen, and the fat content was measured as described by Folch et al. (Citation1957).
The accumulation of cytoplasmic lipid droplets was visualised by Oil Red O staining according to the protocol of Lillie and Fullmer (Citation1976). Briefly, tissues were immediately frozen in liquid nitrogen and cut in a Leica CM-1850 cryostat microtome (Leica, Wetzlar, Germany). Then 16-μm thick sections were fixed in 4% formaldehyde for 10 min and stained with filtered 0.5% Oil Red O (Sigma-Aldrich, St Louis, MO, USA), which was made by dissolving in isopropyl alcohol for 15 min at room temperature. Morphometric analysis was performed on 10 randomly chosen fields containing transverse sections of muscle fibres from the muscles of each chicken. The selected fields were photographed using an Olympus CX41 phase contrast microscope (Olympus, Tokyo, Japan). The volume density of each Oil Red O positive fibre within the muscle tissue was determined using the point-counting method described by Weibel (Citation1973).
Lipoprotein lipase (LPL) activity in muscle tissue was determined using a commercial diagnostic kit (Jiancheng, Nanjing, China). One unit of enzyme activity was defined as 1 μmol of non-esterified fatty acid released from 1 mg of muscle tissue protein per hour. The sensitivity of the assay was 0.04 U/ml, and all samples were included in the same assay to avoid inter-assay variability. The intra-assay coefficient of variation was 7.9%.
AMPK activity was measured by detecting 32P-labelled phosphate released from [32P]ATP (5 μCi/assay) using Ser-Ala77-Met-Ser79 (SAMS) peptide (His–Met–Arg–Ser–Ala–Met–Ser–Gly–Leu–His–Leu–Val–Lys–Arg–Arg) as the target analogue of acetyl-CoA carboxylase as previously described (Davies et al. Citation1989; Winder and Hardie Citation1996). Tissues were homogenised in a buffer with pH 7.5 (50 mM Tris, 250 mM mannitol, 1 mM Na2EDTA, 1 mM ethylene glycol tetraacetic acid (EGTA), 1 mM dithiothreitol, 1 mM phenylmethanesulphfonyl fluoride, 1 mM benzamidine, 4 μg/ml soybean trypsin inhibitor, 50 mM sodium fluoride and 5 mM sodium pyrophosphate) and centrifuged at 14,000g for 20 min at 4°C. The supernatant proteins were incubated with polyethylene glycol 8000 for 10 min at 4°C. The sediments were washed with the homogenisation buffer, centrifuged at 10,000g, re-suspended in a suspending buffer with a pH of 7.5 (100 mM tris, 50 mM sodium fluoride, 5 mM sodium pyrophosphate, 1 mM Na2EDTA, 1 mM EGTA, 1 mM dithiothreitol, 0.02% sodium azide, 1 mM benzamidine, 4 μg/ml soybean trypsin inhibitor and 10% glycerol) and then incubated with a reaction buffer with a pH of 7.0 [40 mM 4-(2-hydroxyethyl)-1-piperazineethanesulphonic acid, 80 mM sodium chloride, 5 mM magnesium chloride, 0.8 mM ethylenediaminetetraacetic acid (EDTA), 0.8 mM dithiothreitol, 0.2 mM SAMS peptide, 0.2 mM γ-[32P]ATP, 0.2 mM AMP, 8% glycerol and 0.01% triton X-100] for 30 min at 30°C. An aliquot of each supernatant was spotted on phosphocellulose paper and washed with 1% phosphoric acid followed by acetone. The paper was air-dried and the incorporated radioactivity was measured using a SN-6930 scintillation counter (Rihuan, Shanghai, China). Protein concentrations were determined using a protein assay kit (Jiancheng).
CPT1 activity was measured according to the method described by Bieber et al. (Citation1972). The muscle tissue was homogenised in Tris–HCl buffer (pH 7.4) containing 120 mM KCl and 1 mM EGTA and then centrifuged (600g, 4°C, 10 min). The supernatant was collected, filtered and centrifuged (17,000g, 4°C, 10 min) again. The precipitate was suspended in buffer and used in assays of CPT1 activity and protein content. A 50 μl of Tris buffer (116 mM, pH 8.0) containing 1.25 mM EDTA, 0.2% Triton X-100 and 2 mM 5,5′-dithiobis-(2-nitrobenzoic acid) was mixed with 50 μl of 1 mM palmitoyl CoA, 5 μl of 1.20 mM L-carnitine and 50 μl of sample, and the absorbance was recorded at 412 nm for 3 min. CPT1 activity was defined as 1 nmol of CoA-SH released from 1 mg of muscle tissue protein per minute. Protein concentrations were determined using a protein assay kit (Jiancheng).
mRNA expression was measured by real-time reverse transcription polymerase chain reaction (RT-PCR). Briefly, total RNA from PM and BF was extracted using Trizol (Invitrogen, San Diego, CA, USA). The quantity and quality of the isolated RNA were determined with a biophotometer (Eppendorf, Hamburg, Germany) and agarose gel electrophoresis. Reverse transcription was performed in RT reactions (10 μl) consisting of 500 ng total RNA, 5 mM MgCl2, 1 μl RT buffer, 1 mM dNTP, 2.5 U reverse transcriptase from Avian Myeloblastosis Virus (AMV), 0.7 nM oligo d(T) and 10 U ribonuclease inhibitor (TaKaRa, Dalian, China). cDNA was amplified in a 20 μl PCR containing 0.2 μM of each primer (Sangon, Shanghai, China) and SYBR® green master mix (TaKaRa). Each cycle consisted of a 5 s denaturation step at 95°C, followed by a 34 s annealing and extension steps at 60°C. mRNA expression was quantified according to the comparative CT method (2− ΔΔCT) of Livak and Schmittgen (Citation2001). The sensitivity of the assay was 8.0 pg total RNA, and all samples were included in the same assay for one gene to avoid inter-assay variability. The intra-assay coefficient of variation was 0.97%. The mRNA levels of target genes were normalised to glyceraldehyde 3-phosphate dehydrogenase (GAPDH) mRNA and 18S ribosomal RNA (18SrRNA) (ΔCT). The ΔCT was calibrated against an average from the control chickens. The number of target molecules relative to the control was calculated using 2− ΔΔC. Therefore, all gene transcription results are reported as the n-fold difference relative to the control. The primer sequences for chicken GR, very low-density lipoprotein receptor (VLDLR), LPL, FATP1, H-FABP, liver carnitine palmitoyltransferase 1 (L-CPT1), LCAD, AMPKα2, GAPDH and 18SrRNA are listed in . The PCR products were verified by electrophoresis on a 0.8% agarose gel and by DNA sequencing. Standard curves were generated using pooled cDNA from the samples being assayed. All samples were run in duplicate, and primers were designed to span an intron to avoid genomic DNA contamination.
Table I. Gene-specific primer of related genes.
Protein extracts (80 μg) were electrophoresed in 7.5–10% sodium dodecyl sulfate (SDS) polyacrylamide gels according to the method of Laemmli (Citation1970). Separated proteins were then transferred onto nitrocellulose membranes at 100 V for 1 h at 4°C in Tris-glycine buffer containing 20% methanol. Membranes were blocked for 1 h and immunoblotted overnight at 4°C with a 1:1000 dilution of an antibody against either phospho-AMPKα (Thr172) or AMPKα (Cell Signaling Technology, Beverly, MA, USA) and ATGL (Cell Signaling Technology). These antibodies have previously been validated for use with chicken samples (Proszkowiec-Weglarz et al. Citation2006; Proszkowiec-Weglarz and Richards Citation2009; Lee et al. Citation2009). Protein detection was performed using goat anti-rabbit IgG (H+L)-horseradish peroxidase (HRP)-conjugated secondary antibody (1:2000, Bio-Rad, Richmond, CA, USA) or goat anti-mouse IgG (H+L)-HRP-conjugated secondary antibody (1:1000, Beyotime, Jiangsu, China) with enhanced chemiluminescence (ECL) plus Western blotting detection reagents (Beyotime). β-Actin was used as an internal control (Beyotime). Protein concentrations were determined using the BicinChoninic Acid assay kit (Beyotime). Western blots were quantified using ImageJ 1.43 software (National Institutes of Health, Bethesda, MD, USA) after densitometric scanning of the films. The sensitivity of the assay was 60 ng protein, and all samples were included in the same assay for one protein to avoid inter-assay variability. The intra-assay coefficient of variation was < 10%.
Statistical analysis
All the data were subjected to one-way ANOVA with the Statistical Analysis Systems statistical software package (Version 8e, SAS Institute, Cary, NC, USA), and the main effect of dexamethasone treatment was evaluated. Homogeneity of variances among groups was confirmed using Bartlett's test (SAS Institute). When the main effect of treatment was significant, differences between means were assessed by Duncan's multiple range analysis. Means were considered significantly different at P < 0.05.
Results
Trial 1: Dexamethasone and terminal 12-h fasting
Both the histological analysis and the quantification of positive muscle fibres in PM (P < 0.0001, F(1,14) = 84.16) and BF (P < 0.0001, F(1,14) = 188.29) indicated that dexamethasone had a significant stimulatory effect on intramyocellular lipid accumulation compared with control ().
Figure 1. Oil Red O staining of skeletal muscle for cytoplasmic lipid droplets (indicated by arrows) showing effect of dexamethasone treatment (DEX, daily subcutaneous injection of 2 mg/kg body weight for 3 days, B,D) on lipid accumulation in myocytes of PM (A,B) and BF (C,D) from broiler chickens in Trial 1. The scale bar in (A) represents 50 μm. (E) Indicates the volume density (Vv × 40) of Oil Red O positive muscle fibres in skeletal muscle. Values are means ± SE (n = 5). Different superscripts (a,b) indicate significant differences (P < 0.05) in the means, by ANOVA and Duncan's multiple test.
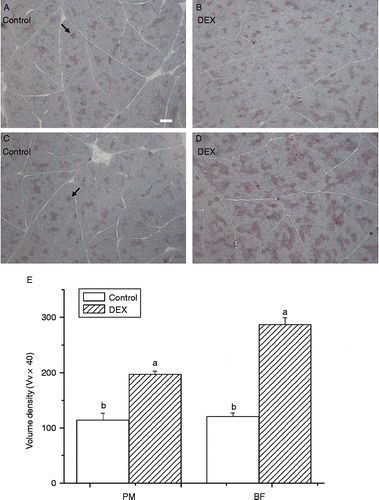
The protein content of β-actin (internal control) was not significantly affected in either PM or BF between groups (P>0.05; ). Compared with the control, phospho-AMPKα (Thr172) was down-regulated by dexamethasone in both PM (P < 0.01, F(1,6) = 32.09) and BF (P < 0.05, F(1,6) = 9.21) ().
Figure 2. Effect of dexamethasone treatment (DEX, daily subcutaneous injection of 2 mg/kg body weight for 3 days) on protein levels of β-actin (A) and phospho-AMPK αThr172 (B) in PM and BF from broiler chickens in Trial 1. Values are means ± SE (n = 4). Different superscripts (a,b) indicate significant differences (P < 0.05) in the means, by ANOVA and Duncan's multiple test.
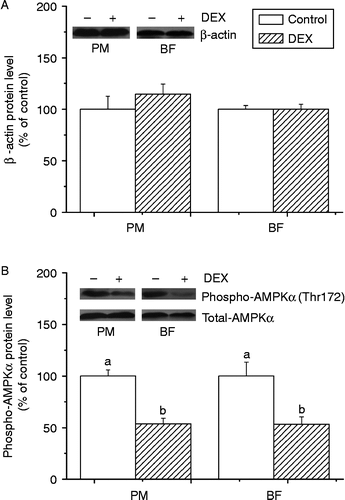
Compared with control, dexamethasone induced a decrease in CPT1 activity in PM (P < 0.05, F(1,13) = 6.22). The same trend was observed in BF, but this was not statistically significant (P>0.05) ().
Figure 3. Effect of dexamethasone treatment (DEX, daily subcutaneous injection of 2 mg/kg body weight for 3 days) on CPT1 activity (nmol/min/mg protein) in PM and BF from broiler chickens in Trial 1. Values are means ± SE (n = 8). Different superscripts (a,b) indicate significant differences (P < 0.05) in the means, by ANOVA and Duncan's multiple test.
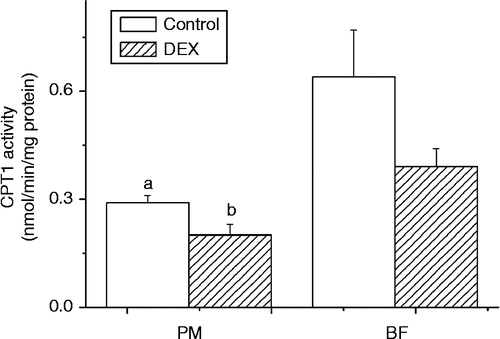
Trial 2: Dexamethasone, pair-feeding and terminal 12-h fasting or feeding
Chicken growth and tissue development
The mean body weight gain of broiler chickens was significantly decreased (P < 0.0001, F(2,6) = 453.81) by dexamethasone treatment, and was lower than that of control and pair-fed counterparts (). Compared with control and pair-fed chickens, dexamethasone-treated chickens had retarded breast (PM) development (P < 0.01, F(2,20) = 6.42) but unaltered thigh (BF) muscle yield (P>0.05) (). The total fat content in both PM and BF from feeding (PM: P < 0.0001, F(2,21) = 42.54; BF: P < 0.0001, F(2,21) = 49.96) and fasted chickens (PM, P = 0.0548, F(2,21) = 3.35; BF, P < 0.05, F(2,21) = 3.85) was increased by dexamethasone treatment compared with pair-fed counterparts. In contrast, the fasting pair-fed chickens had lower fat content in both the PM and BF when compared with control and dexamethasone-treated chickens ().
Table II. Effect of dexamethasone treatment (daily subcutaneous injection, 2 mg/kg body weight for 3 days) on body weight gain, skeletal muscle growth and lipid accumulation in skeletal muscle of broiler chickens.
Enzyme activity
Dexamethasone did not significantly affect the LPL activity in PM of feeding chickens compared with control and pair-fed groups (P>0.05), but decreased the LPL activity in PM of fasting chickens compared with the pair-fed group (P < 0.05, F(2,17) = 4.18) (). In BF, dexamethasone had no influence on LPL activity (P>0.05) during either feeding or fasting compared with control and pair-fed chickens (). AMPK activity was increased by dexamethasone in PM compared with control (P = 0.0757, F(2,15) = 3.08) and in BF compared with control and pair-fed groups (P < 0.05, F(2,15) = 6.16) during feeding but not during fasting (). Generally, the activities of LPL and AMPK were higher in BF than in PM and were lower in fed chickens than in fasted chickens ().
Figure 4. Effect of vehicle (control), dexamethasone (DEX, daily subcutaneous injection of 2 mg/kg body weight for 3 days) or vehicle and pair-feeding (pair-fed) on the activity of LPL (U/mg protein) and AMPK (10 CPM/ng protein) in PM (A) and BF (B) from broiler chickens during feeding or fasting. CPM, count per minute. Values are means ± SE (n = 8). Different superscripts (a,b) indicate significant differences (P < 0.05) in the means, by ANOVA and Duncan's multiple test.
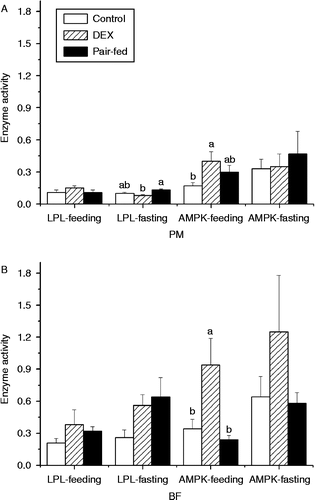
mRNA expression
During feeding, dexamethasone-treated chickens had higher levels of GR (P < 0.0001, F(2,15) = 26.75), VLDLR (P < 0.001, F(2,20) = 13.61), FATP1 (P < 0.0001, F(2,18) = 30.54), H-FABP (P < 0.0001, F(2,20) = 17.06) and LCAD (P < 0.0001, F(2,18) = 37.26) mRNA in PM compared with the control and pair-fed chickens (). During fasting, dexamethasone down-regulated the expression of mRNAs for LPL (P < 0.05, F(2,21) = 4.28) and L-CPT1 (P < 0.05, F(2,21) = 4.18) compared with control, while it up-regulated FATP1 (P < 0.0001, F(2,21) = 28.41), H-FABP (P < 0.05, F(2,21) = 4.61) and LCAD (P < 0.01, F(2,21) = 7.08) mRNA expression compared with the control and pair-fed groups. Dexamethasone increased GR mRNA level compared with the pair-fed group (P < 0.05, F(2,20) = 4.51) (). Dexamethasone treatment and pair-feeding treatment decreased AMPKα2 expression during both feeding (P < 0.01, F(2,20) = 8.27) and fasting (P < 0.01, F(2,20) = 9.48) compared with control ().
Figure 5. Effect of vehicle (control), dexamethasone (DEX, daily subcutaneous injection of 2 mg/kg body weight for 3 days) or vehicle and pair-feeding (pair-fed) on mRNA expression in PM. mRNAs from broiler chickens during feeding (A,B) and fasting (C,D). Values are means ± SE (n = 8). Different superscripts (a,b) indicate significant differences (P < 0.05) in the means, by ANOVA and Duncan's multiple test.
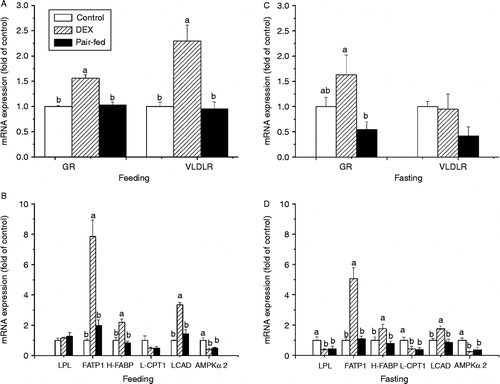
In BF of fed chickens, the levels of mRNAs for GR (P < 0.001, F(2,20) = 11.87), VLDLR (P < 0.001, F(2,20) = 12.33), LPL (P < 0.05, F(2,21) = 5.37), FATP1 (P < 0.0001, F(2,20) = 27.84), H-FABP (P < 0.001, F(2,21) = 11.22), LCAD (P < 0.001, F(2,21) = 12.42) and AMPKα2 (P < 0.001, F(2,21) = 13.52) were all significantly up-regulated by dexamethasone treatment compared with the control and pair-fed groups, and in dexamethasone and pair-fed chickens L-CPT1 expression was increased compared with the control group (P < 0.01, F(2,21) = 9.11) (). During fasting, dexamethasone up-regulated the levels of GR (P < 0.05, F(2,19) = 5.89), FATP1 (P < 0.01, F(2,19) = 10.14) and L-CPT1 (P < 0.01, F(2,18) = 6.76) mRNA in BF when compared with control and pair-fed chickens. However, no significant differences (P>0.05) between treatments were found in the mRNA expression levels of VLDLR, LPL, H-FABP, LCAD and AMPKα2 ().
Figure 6. Effect of vehicle (control), dexamethasone (DEX, daily subcutaneous injection of 2 mg/kg body weight for 3 days) or vehicle and pair-feeding (pair-fed) on mRNA expression in BF. mRNA levels from broiler chickens during feeding (A,B) and fasting (C,D). Values are means ± SE (n = 8). Different superscripts (a,b) indicate significant differences (P < 0.05) in the means, by ANOVA and Duncan's multiple test.
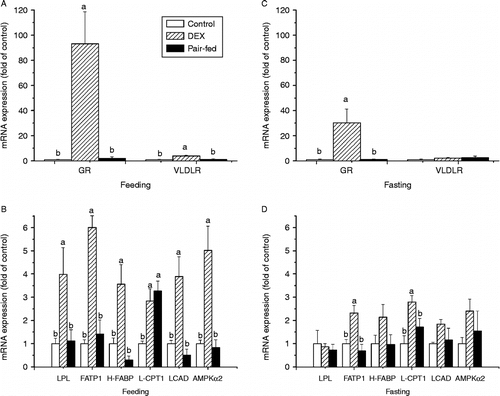
Protein expression
The protein level of ATGL was not significantly affected by dexamethasone treatment compared with control and pair-fed groups, regardless of the muscle type and the feeding state (P>0.05, ).
Figure 7. Effect of vehicle (control), dexamethasone (DEX, daily subcutaneous injection of 2 mg/kg body weight for 3 days) or vehicle and pair-feeding (pair-fed) on protein levels of ATGL in PM and BF from broiler chickens during feeding (A) and fasting (B). Values are means ± SE (n = 4). Different superscripts (a,b) indicate significant differences (P < 0.05) in the means, by ANOVA and Duncan's multiple test.
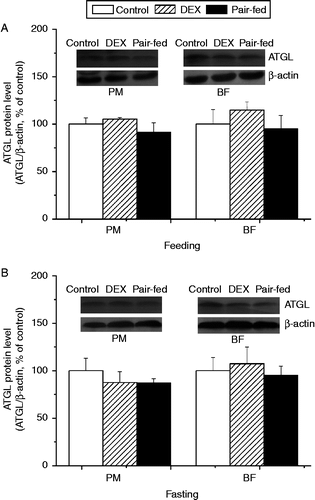
Discussion
In the present study, the effects of glucocorticoid on intramyocellular lipid accumulation and involvement of the AMPK signal pathway were investigated. The results indicate that dexamethasone facilitates intramyocellular lipid accumulation in a tissue-dependent manner during the period of maximum muscle growth. The results suggest that unmatched fatty acid uptake and utilisation may be responsible for glucocorticoid-induced intramyocellular lipid accumulation in broiler chickens, and suppressed AMPK signalling may be involved.
Dexamethasone alters energy redistribution towards lipid deposition
The administration of dexamethasone at a dose of 1 mg/kg body weight has been reported to induce an alteration in fatty acid and glucose metabolism in rats (Qi et al. Citation2004) and chickens (Wang et al. Citation2010). In our previous work, we showed that dexamethasone treatment induced an increase in the plasma concentrations of glucose, insulin and lipid during both feeding and fasting (Cai et al. Citation2011). The increase in circulating insulin level is suggested to be associated with enhanced de novo hepatic lipogenesis in dexamethasone-treated chickens (Cai et al. Citation2009), and the significantly increased levels of triglyceride and very low-density lipoprotein (VLDL) in the plasma during both feeding and fasting indicate increased blood lipid flux (Yuan et al. Citation2008; Cai et al. Citation2011).
Significantly decreased feed intake was observed previously with dexamethasone treatment (Cai et al. Citation2011). In order to exclude a possible effect of feed intake, a pair-fed group was employed in the present study. The markedly retarded body weight gain and muscle growth in dexamethasone-treated chickens, compared with control or pair-fed chickens, is in line with previous studies in rats (Bowes et al. Citation1996) and chickens (Lin et al. Citation2004; Dong et al. Citation2007; Wang et al. Citation2010), indicating that the muscle development is suppressed by the administration of exogenous glucocorticoids. In contrast, fat accumulation in adipose tissue of dexamethasone-treated chickens was increased compared with both control (abdominal fat, +6.43%; cervical fat, +14.1%, thigh fat, +26.5%) and pair-fed (abdominal fat, +9.91%; cervical fat, +17.5%, thigh fat, +35.6%) chickens. The increased fat storage in adipose tissues accompanied by the retardation in skeletal muscle development indicates that energy is redistributed towards lipid deposition during glucocorticoid challenge (Jiang et al. Citation2008; Yuan et al. Citation2008).
In rats, dexamethasone causes an increase in visceral fat accumulation and intramyocellular lipid levels (Korach-André et al. Citation2005). In our previous study, we showed that in 10-day-old chicks, fat accumulation in abdominal and subcutaneous adipose tissues is enhanced by dexamethasone administration and intramyocellular lipid content tends to be increased (Wang et al. Citation2010). In the present study, the experimental chickens were around the age of maximum growth rate (Sakomura et al. Citation2005). Dexamethasone treatment caused a significant increase in the lipid content of skeletal muscle (). Moreover, the fat weight was higher in dexamethasone-fed chickens for both PM (dexamethasone, 14.76 g; control, 9.87 g; pair-fed, 10.53 g) and BF (dexamethasone, 11.76 g; control, 7.19 g; pair-fed, 8.72 g), indicating that the increased lipid accumulation occurred regardless of the decreased muscle weight. However, the fat weight was not altered by dexamethasone in the fasting state. The increased circulating lipid flux together with the enhanced lipid accumulation indicates that increased fatty acid availability and uptake may be involved in the augmented intramyocellular lipid accumulation. This result is in accordance with the increased amount of intramyocellular lipid droplets (), indicating that dexamethasone increases intramyocellular fat accumulation.
Dexamethasone facilitates intramyocellular lipid accumulation in a tissue-dependent manner
We have previously shown that dexamethasone increases hepatic de novo lipogenesis and circulating lipid flux (Cai et al. Citation2009). In the present study, we investigated the direct role of dexamethasone in muscle lipid metabolism in the presence or absence of dietary fatty acid substrate, which was achieved during 12-h feeding or fasting states, respectively (Chen et al. Citation2007).
Dexamethasone increases lipid availability in peripheral tissues by increasing the level of circulating VLDL (Yuan et al. Citation2008; Cai et al. Citation2009). In muscle cells, LPL is responsible for the release of fatty acids from triglycerides carried in the circulating lipoproteins, whereas VLDLR facilitates VLDL catabolism both by enhancing LPL activity and by binding to VLDL remnants and internalising them into cells (Argraves et al. Citation1995). In mammals, increased LPL activity is strongly associated with fat deposition, which is regulated by both insulin and glucocorticoids (Fried et al. Citation1993). Both glucocorticoids and starvation cause an increase in skeletal muscle LPL activity (Lithell et al. Citation1981; Nikkilä Citation1987; Ladu et al. Citation1991). In line with the results in mammals, the present study showed increases by fasting in BF rather than in PM for LPL activity (PM: feeding, 0.12 vs. fasting, 0.10 U/mg protein; BF: feeding, 0.30 vs. fasting, 0.49 U/mg protein) and gene expression level (PM, fasting 0.51-fold vs. BF, 2.53-fold), indicating the tissue specificity of fatty acid utilisation. In BF, the higher LPL activity in dexamethasone-treated chickens compared with control in either feeding (+80.9%, P>0.1) or fasting (+115.4%, P = 0.100) states indicated that LPL activity may be increased by dexamethasone in broilers, as it is in mammals. The protein level of ATGL, catalysing the initial step of triglyceride hydrolysis (Zimmermann et al. Citation2004), was further investigated. In contrast to the result for 3T3-L1 pre-adipocytes (Villena et al. Citation2004), the protein expression of ATGL was not significantly altered by dexamethasone treatment regardless of feeding state and muscle types. In contrast, VLDLR mRNA expression was significantly up-regulated by dexamethasone treatment during feeding but not during fasting, regardless of the muscle type. Together, this result indicates that dexamethasone treatment may facilitate the fatty acid uptake from blood by skeletal muscle in a tissue-specific way.
FATP1 and H-FABP have been shown to facilitate long-chain fatty acid uptake and utilisation in both skeletal and cardiac muscles (Corcoran et al. Citation2007). In agreement with previous work in mammals (Veerkamp and Van Moerkerk Citation1993; Maatman et al. Citation1994; Van Nieuwenhoven et al. Citation1995; Kempen et al. Citation1998; Corcoran et al. Citation2007), our study shows that the transcriptional levels of FATP1 and H-FABP are increased following dexamethasone treatment in both PM (FATP1, 7.85-fold; H-FABP, 2.19-fold) and BF (FATP1, 6.01-fold; H-FABP, 3.57-fold), indicating that capacity for fatty acid uptake by muscle cells is likely to be increased (). As this is the case during both feeding and fasting, the effect of dexamethasone on lipid uptake in 35-day-old chickens used in our study seems to be independent of feeding status. By contrast, dexamethasone treatment had no influence on the fatty acid uptake-related gene expression in either PM or BF muscles in young (10-day-old) chicks (Wang et al. Citation2010), indicating that the effects of dexamethasone may be age related.
CPT1 and LCAD are the enzymes responsible for the transport and oxidation of fatty acids in mitochondria. In the present study, we investigated the expression of L-CPT1 because L-CPT1 mRNA expression seems to be more sensitive than muscle-CPT1 to an energy deficit in chickens (Skiba-Cassy et al. Citation2007). During feeding, the expression of LCAD mRNA in PM (3.35-fold increase, ) and both L-CPT1 and LCAD mRNA in BF (L-CPT1, 2.84-fold increase; LCAD, 3.89-fold increase, ) were up-regulated by dexamethasone treatment, showing that fatty acid oxidation seems to be enhanced by dexamethasone. During fasting, mRNA expression was similar to that during feeding, except that LCAD expression in BF was not significantly influenced by dexamethasone treatment. Although the significant effect of dexamethasone on CPT1 activity was only detected in PM, CPT1 activity was decreased in both PM ( − 45.0%, P = 0.027) and BF ( − 64.1%, P = 0.107) during fasting (), indicating that fatty acid oxidation may be depressed in both glycolytic and oxidative muscles. These results indicate that post-transcriptional control is responsible for changes in CPT1 activity. Together, these results suggest that the unmatched fatty acid uptake and utilisation are likely to be responsible for augmented intramyocellular lipid accumulation. This hypothesis is supported by the observation that the dexamethasone-treated and pair-fed chickens had a higher lipid content in skeletal muscle during feeding than during fasting in both breast muscle (dexamethasone, +64.0%, pair-fed, +25.7%) and thigh muscle (dexamethasone, +50.9%, pair-fed, +32.5%).
Perilipin, a major lipid droplet-associating protein, protects against lipolysis (Greenberg et al. Citation1993; Blanchette-Mackie et al. Citation1995). In contrast, hormone-sensitive lipase (HSL) has been shown to catalyse lipolysis in skeletal muscle with a higher specificity for diglyceride than triglyceride (Langfort et al. Citation1999). The effects of HSL-induced lipolysis and perilipin-related lipogenesis in the augmented lipid accumulation cannot be excluded.
In rats, acute dexamethasone treatment promotes acetyl-CoA carboxylase phosphorylation and increases cardiac palmitate oxidation, likely by increasing AMPK phosphorylation and total AMPK protein and gene expression (Qi et al. Citation2006). AMPKα2 is preferentially expressed in the skeletal muscle of chickens (Proszkowiec-Weglarz et al. Citation2006), and it has been proposed that α2 plays a greater role than α1 in mediating AMPK activities. Phosphorylation of the α-isoform at Thr172 by an upstream kinase is known to be required for AMPK activity (Hardie Citation2007). In line with our previous study (Wang et al. Citation2010), the AMPK activity was increased by dexamethasone treatment during feeding but not during fasting, indicating that the effect of dexamethasone on AMPK activity is related to feeding state. Furthermore, the dexamethasone-induced reduction in AMPK phosphorylation during fasting suggests that lipid oxidation may be retarded (). However, AMPKα2 expression was down-regulated by dexamethasone in PM but up-regulated in BF regardless of feeding state (), indicating that the effects of dexamethasone treatment are tissue specific, which is in line with previous work (Christ-Crain et al. Citation2008; Wang et al. Citation2010). These results indicate that dexamethasone may alter fatty acid oxidation by means of alterations in AMPK phosphorylation and activity rather than by affecting the transcription of AMPK. In rats, treatment with glucocorticoids influences LKB1/AMPK signalling in skeletal muscle, which contributes in part to the decrease in insulin sensitivity and adiposity (Nakken et al. Citation2010). Glucocorticoid-induced hyperglycaemia and hyperinsulinaemia have been demonstrated in our previous studies (Yuan et al. Citation2008; Zhao et al. Citation2009a,Citationb; Cai et al. Citation2011). In the present study, we showed that disruptions in fatty acid oxidation may interfere with the utilisation of glucose by muscle cells, which in turn may contribute to insulin resistance.
The effect of glucocorticoids is mediated by ligand-dependent activation of GR, which is expressed in almost every cell type. The activated GR acts as a transcription factor, controlling the level of target gene expression and modulating intracellular signalling pathways (Beato et al. Citation1995; Tronche et al. Citation1998; Herrlich Citation2001; Karin and Chang Citation2001; Hafezi-Moghadam et al. Citation2002). A dexamethasone-induced increase in GR expression occurred in PM and BF muscles during both feeding and fasting (), suggesting that the regulatory role of glucocorticoids is enhanced in muscle tissues. In humans, up-regulation of GR in skeletal muscle is associated with insulin resistance, high body mass index and fat accumulation, indicating that increased GR expression may play a fundamental role in mechanisms contributing to the pathogenesis of the metabolic syndrome (Whorwood et al. Citation2002). In chickens, Taouis et al. (Citation1993) and Dupont et al. (Citation1999) reported that chronic injection of corticosterone induced insulin resistance. We have also shown that glucocorticoids induce hyperglycaemia and hyperinsulinaemia in previous studies (Yuan et al. Citation2008; Zhao et al., Citation2009a,Citationb; Cai et al. Citation2011). The greater increase in GR expression level in BF (feeding, 93-fold; fasting, 30-fold) than in PM (feeding, 1.6-fold; fasting, 1.6-fold) may indicate that thigh muscle is more sensitive to the regulatory effects of glucocorticoids. The result may be associated with the greater efficiency of slow-twitch muscles in fuel production when compared with fast-twitch muscles.
In conclusion, the results indicate that dexamethasone facilitates intramyocellular lipid accumulation in a tissue-dependent manner during the period of maximum muscle growth. Dexamethasone may facilitate fatty acid uptake from blood by skeletal muscle in a tissue-specific way. In the fasting state, dexamethasone-induced-retarded fatty acid utilisation may be involved in the augmented intramyocellular lipid accumulation in both glycolytic and oxidative muscles. In the feeding state, dexamethasone promotes the transcriptional activity of genes related to lipid uptake and oxidation in muscles. These results suggest that dexamethasone-induced unmatched fatty acid uptake and utilisation are likely to be responsible for the increase in intramyocellular lipid accumulation, and the suppressed AMPK signal may be involved.
Acknowledgements
This work was supported by grants from the Shandong Science Fund for Distinguished Youth Scholars and the National Natural Science Foundation of China (No. 30771573).
Declaration of interest: The authors report no conflicts of interest. The authors alone are responsible for the content and writing of the paper.
References
- Andrews RC, Walker BR. 1999. Glucocorticoids and insulin resistance: Old hormones, new targets. Clin Sci. 96:513–523.
- Argraves KM, Battey FD, MacCalman CD, McCrae KR, Gåfvels M, Kozarsky KF, Chappell DA, Strauss JFIII, Strickland DK. 1995. The very low density lipoprotein receptor mediates the cellular catabolism of lipoprotein lipase and urokinase-plasminogen activator inhibitor type I complexes. J Biol Chem. 270:26550–26557.
- Beato M, Herrlich P, Schutz G. 1995. Steroid hormone receptors: Many actors in search of a plot. Cell. 83:851–857.
- de Beaudrap P, Witten G, Biltz G, Perrier E. 2006. Mechanistic model of fuel selection in the muscle. J Theor Biol. 242:151–163.
- Bieber LL, Abraham T, Helmrath T. 1972. A rapid spectrophotometric assay for carnitine palmitoltransferase. Anal Biochem. 50:509–518.
- Blanchette-Mackie EJ, Dwyer NK, Barber T, Coxey RA, Takeda T, Rondinone CM, Theodorakis JL, Greenberg AS, Londos C. 1995. Perilipin is located on the surface layer of intracellular lipid droplets in adipocytes. J Lipid Res. 36:1211–1226.
- Bowes SB, Jackson NC, Papachristodoulou D, Umpleby AM, Sönksen PH. 1996. Effect of corticosterone on protein degradation in isolated rat soleus and extensor digitorum longus muscles. J Endocrinol. 148:501–507.
- Braun EJ, Sweazea KL. 2008. Glucose regulation in birds. Comp Biochem Physiol. 151:B1–B5.
- Cai YL, Song ZG, Zhang XH, Wang XJ, Jiao HC, Lin H. 2009. Increased de novo lipogenesis in liver contributes to the augmented fat deposition in dexamethasone exposed broiler chickens (Gallus gallus domesticus). Comp Biochem Physiol. 150:C164–C169.
- Cai YL, Song ZG, Wang XJ, Jiao HC, Lin H. 2011. Dexamethasone-induced hepatic lipogenesis is insulin dependent in chickens (Gallus gallus domesticus). Stress. 14:273–281.
- Chen LL, Jiang QY, Zhu XT, Shu G, Bin YF, Wang XQ, Gao P, Zhang YL. 2007. Ghrelin ligand-receptor mRNA expression in hypothalamus, proventriculus and liver of chicken (Gallus gallus domesticus): Studies on ontogeny and feeding condition. Comp Biochem Physiol. 147:A893–A902.
- Christ-Crain M, Kola B, Lolli F, Fekete C, Seboek D, Wittmann G, Feltrin D, Igreja SC, Ajodha S, Harvey-White J, Kunos G, Müller B, Pralong F, Aubert G, Arnaldi G, Giacchetti G, Boscaro M, Grossman AB, Korbonits M. 2008. AMP-activated protein kinase mediates glucocorticoid-induced metabolic changes: A novel mechanism in Cushing's syndrome. FASEB J. 22:1672–1683.
- Close B, Banister K, Baumans V, Bernoth EM, Bromage N, Bunyan J, Erhardt W, Flecknell P, Gregory N, Hackbarth H, Morton D, Warwick C. 1997. Recommendations for euthanasia of experimental animals: Part 2. DGXT of the European Commission. Lab Anim. 31:1–32.
- Coppack SW, Jensen MD, Miles JM. 1994. In vivo regulation of lipolysis in humans. J Lipid Res. 35:177–193.
- Corcoran MP, Lamon-Fava S, Fielding RA. 2007. Skeletal muscle lipid deposition and insulin resistance: Effect of dietary fatty acids and exercise. Am J Clin Nutr. 85:662–677.
- Davies SP, Carling D, Hardie DG. 1989. Tissue distribution of the AMP-activated protein kinase, and lack of activation by cyclic-AMP-dependent protein kinase, studied using a specific and sensitive peptide assay. Eur J Biochem. 186:123–128.
- Dong H, Lin H, Jiao HC, Song ZG, Zhao JP, Jiang KJ. 2007. Altered development and protein metabolism in skeletal muscle of broiler chickens (Gallus gallus domesticus) by corticosterone. Comp Biochem Physiol. 147:A189–A195.
- Dupont J, Derouet M, Simon J, Taouis M. 1999. Corticosterone alters insulin signaling in chicken muscle and liver at different steps. J Endocrinol. 162:67–76.
- Dupont J, Dagou C, Derouet M, Simon J, Taouis M. 2004. Early steps of insulin receptor signaling in chicken and rat: Apparent refractoriness in chicken muscle. Domest Anim Endocrinol. 26:127–142.
- Dupont J, Tesseraud S, Derouet M, Collin A, Rideau N, Crochet S, Godet E, Cailleau-Audouin E, Métayer-Coustard S, Duclos MJ, Gespach C, Porter TE, Cogburn LA, Simon J. 2008. Insulin immuno-neutralization in chicken: Effects on insulin signaling and gene expression in liver and muscle. J Endocrinol. 197:531–542.
- Eaton S. 2002. Control of mitochondrial β-oxidation flux. Prog Lipid Res. 41:197–239.
- Folch J, Lees M, Sloane Stanley GH. 1957. A simple method for the isolation and purification of total lipids from animal tissues. J Biol Chem. 226:497–509.
- Foucaud L, Niot I, Kanda T, Besnard P. 1998. Indirect dexamethasone down-regulation of the liver fatty acid-binding protein expression in rat liver. Biochim Biophys Acta. 1391:204–212.
- Freedman MR, Horwitz BA, Stern JS. 1986. Effect of adrenalectomy and glucocorticoid replacement on development of obesity. Am J Physiol. 250:R595–R607.
- Fried SK, Russell CD, Grauso NL, Brolin RE. 1993. Lipoprotein lipase regulation by insulin and glucocorticoid in subcutaneous and omental adipose tissues of obese women and men. J Clin Invest. 92:2191–2198.
- Greenberg AS, Egan JJ, Wek SA, Moos MCJr., Londos C, Kimmel AR. 1993. Isolation of cDNAs for perilipins A and B: Sequence and expression of lipid droplet-associated proteins of adipocytes. Proc Natl Acad Sci USA. 90:12035–12039.
- Haemmerle G, Lass A, Zimmermann R, Gorkiewicz G, Meyer C, Rozman J, Heldmaier G, Maier R, Theussl C, Eder S, Kratky D, Wagner EF, Klingenspor M, Hoefler G, Zechner R. 2006. Defective lipolysis and altered energy metabolism in mice lacking adipose triglyceride lipase. Science. 312:734–737.
- Hafezi-Moghadam A, Simoncini T, Yang E, Limbourg FP, Plumier JC, Rebsamen MC, Hsieh CM, Chui DS, Thomas KL, Prorock AJ, Laubach VE, Moskowitz MA, French BA, Ley K, Liao JK. 2002. Acute cardiovascular protective effects of corticosteroids are mediated by non-transcriptional activation of endothelial nitric oxide synthase. Nat Med. 8:473–479.
- Halevy O, Geyra A, Barak M, Uni Z, Sklan D. 2000. Early posthatch starvation decreases satellite cell proliferation and skeletal muscle growth in chicks. J Nutr. 130:858–864.
- Hancock CR, Han DH, Chen M, Terada S, Yasuda U, Wright DC, Holloszy JO. 2008. High-fat diets cause insulin resistance despite an increase in muscle mitochondria. Proc Natl Acad Sci USA. 105:7815–7820.
- Hardie DG. 2007. AMP-activated protein kinase as a drug target. Annu Rev Pharmacol Toxicol. 47:185–210.
- Herrlich P. 2001. Cross-talk between glucocorticoid receptor and AP-1. Oncogene. 20:2465–2475.
- Izai K, Uchida Y, Orii T, Yamamoto S, Hashimoto T. 1992. Novel fatty acid beta-oxidation enzymes in rat liver mitochondria. I. Purification and properties of very-long-chain acyl-coenzyme A dehydrogenase. J Biol Chem. 267:1027–1033.
- Jiang KJ, Jiao HC, Song ZG, Yuan L, Zhao JP, Lin H. 2008. Corticosterone administration and dietary glucose supplementation enhance fat accumulation in broiler chickens. Br Poult Sci. 49:625–631.
- Karin M, Chang L. 2001. AP-1-glucocorticoid receptor crosstalk taken to a higher level. J Endocrinol. 169:447–451.
- Kelley DE, Goodpaster BH. 2001. Skeletal muscle triglyceride: An aspect of regional adiposity and insulin resistance. Diabetes Care. 24:933–941.
- Kelley DE, Mandarino LJ. 2000. Fuel selection in human skeletal muscle in insulin resistance: A reexamination. Diabetes. 49:677–683.
- Kempen KPG, Saris WHM, Kuipers H, Glatz JFC, Van Der Vusse GJ. 1998. Skeletal muscle metabolic characteristics before and after energy restriction in human obesity: Fibre type, enzymatic beta-oxidative capacity and fatty acid-binding protein content. Eur J Clin Invest. 28:1030–1037.
- Komamura K, Shirotani-Ikejima H, Tatsumi R, Tsujita-Kuroda Y, Kitakaze M, Miyatake K, Sunagawa K, Miyata T. 2003. Differential gene expression in the rat skeletal and heart muscle in glucocorticoid-induced myopathy: Analysis by microarray. Cardiovasc Drugs Ther. 17:303–310.
- Korach-André M, Gao J, Gounarides JS, Deacon R, Islam A, Laurent D. 2005. Relationship between visceral adiposity and intramyocellular lipid content in two rat models of insulin resistance. Am J Physiol. 288:E106–E116.
- Koves TR, Ussher JR, Noland RC, Slentz D, Mosedale M, Ilkayeva O, Bain J, Stevens R, Dyck JRB, Newgard CB, Lopaschuk GD, Muoio DM. 2008. Mitochondrial overload and incomplete fatty acid oxidation contribute to skeletal muscle insulin resistance. Cell Metab. 7:45–56.
- Ladu MJ, Kapsas H, Palmer WK. 1991. Regulation of lipoprotein lipase in adipose and muscle tissues during fasting. Am J Physiol. 260:R953–R959.
- Laemmli UK. 1970. Cleavage of structural proteins during the assembly of the head of bacterio phage T4. Nature. 227:680–685.
- Langfort J, Ploug T, Ihlemann J, Saldo M, Holm C, Galbo H. 1999. Expression of hormone-sensitive lipase and its regulation by adrenaline in skeletal muscle. Biochem J. 340:459–465.
- Lee K, Shin J, Latshaw JD, Suh Y, Serr J. 2009. Cloning of adipose triglyceride lipase complementary deoxyribonucleic acid in poultry and expression of adipose triglyceride lipase during development of adipose in chickens. Poult Sci. 88:620–630.
- Lillie RD, Fullmer HM. 1976. Histopathologic technic and practical histochemistry. New York: McGraw-Hill.
- Lin H, Decuypere E, Buyse J. 2004. Oxidative stress induced by corticosterone administration in broiler chickens (Gallus gallus domesticus): 1. Chronic exposure. Comp Biochem Physiol. 139:B737–B744.
- Lithell H, Cedermark M, Fröberg J, Tesch P, Karlsson J. 1981. Increase of lipoprotein-lipase activity in skeletal muscle during heavy exercise. Relation to epinephrine excretion. Metabolism. 30:1130–1134.
- Livak KJ, Schmittgen TD. 2001. Analysis of relative gene expression data using real-time quantitative PCR and the 2 ( − Delta Delta C (T)) method. Methods. 25:402–408.
- Maatman RGHJ, Van Moerkerk HTB, Nooren IMA, Van Zoelen EJJ, Veerkamp JH. 1994. Expression of human liver fatty acid binding protein in Escherichia coli and comparative analysis of its binding characteristics with muscle fatty acid binding protein. Biochim Biophys Acta. 1214:l–10.
- Matteri RL, Carroll JA, Dyer CJ. 2000. Neuroendocrine responses to stress. In: Moberg GP, Mench JA. editors. The biology of animal stress. Wallingford, Oxon, UK: CAB International43–56.
- McGarry JD, Brown NF. 1997. The mitochondrial carnitine palmitoyltransferase system. From concept to molecular analysis. Eur J Biochem. 244:1–14.
- McGarry JD, Woeltje KF, Kuwajima M, Foster DW. 1989. Regulation of ketogenesis and the renaissance of carnitine palmitoyltransferase. Diabetes Metab Res Rev. 5:271–284.
- Nakken GN, Jacobs DL, Thomson DM, Fillmore N, Winder WW. 2010. Effects of excess corticosterone on LKB1 and AMPK signaling in rat skeletal muscle. J Appl Physiol. 108:298–305.
- Nikkilä EA. 1987. Role of lipoprotein lipase in metabolic adaptation to exercise and training. In: Borensztajn J. editors. Lipoprotein lipase. Chicago, IL: Evener187–199.
- Post J, Rebel JM, ter Huurne AA. 2003. Physiological effects of elevated plasma corticosterone concentrations in broiler chickens, an alternative means by which to assess the physiological effects of stress. Poult Sci. 82:1313–1318.
- Proszkowiec-Weglarz M, Richards MP. 2009. Expression and activity of the 5′-adenosine monophosphate-activated protein kinase pathway in selected tissues during chicken embryonic development. Poult Sci. 88:159–178.
- Proszkowiec-Weglarz M, Richards MP, Ramachandran R, McMurtry JP. 2006. Characterization of the AMP-activated protein kinase pathway in chickens. Comp Biochem Physiol. 143:B92–B106.
- Qi D, Pulinilkunnil T, An D, Ghosh S, Abrahani A, Pospisilik JA, Brownsey R, Wambolt R, Allard M, Rodrigues B. 2004. Single-dose dexamethasone induces whole-body insulin resistance and alters both cardiac fatty acid and carbohydrate metabolism. Diabetes. 53:1790–1797.
- Qi D, An D, Kewalramani G, Qi Y, Pulinilkunnil T, Abrahani A, Al-Atar U, Ghosh S, Wambolt RB, Allard MF, Innis SM, Rodrigues B. 2006. Altered cardiac fatty acid composition and utilization following dexamethasone-induced insulin resistance. Am J Physiol. 291:E420–E427.
- Ruderman NB, Saha AK. 2006. Metabolic syndrome: Adenosine monophosphate-activated protein kinase and malonyl coenzyme A. Obesity. 14:25S–33S.
- Sakomura NK, Longo FA, Oviedo-Rondon EO, Boa-Viagem C, Ferraudo A. 2005. Modeling energy utilization and growth parameter description for broiler chickens. Poult Sci. 84:1363–1369.
- Scheuermann GN, Bilgili SF, Hess JB, Mulvaney DR. 2003. Breast muscle development in commercial broiler chickens. Poult Sci. 82:1648–1658.
- Skiba-Cassy S, Collin A, Chartrin P, Médale F, Simon J, Duclos MJ, Tesseraud S. 2007. Chicken liver and muscle carnitine palmitoyltransferase 1: Nutritional regulation of messengers. Comp Biochem Physiol. 147:B278–B287.
- Taouis M, Derouet M, Chevalier B, Simon J. 1993. Corticosterone effect on insulin receptor number and kinase activity in chicken muscle and liver. Gen Comp Endocrinol. 89:167–175.
- Tronche F, Kellendonk C, Reichardt HM, Schütz G. 1998. Genetic dissection of glucocorticoid receptor function in mice. Curr Opin Genet Dev. 8:532–538.
- Urdaneta-Rincon M, Leeson S. 2002. Quantitative and qualitative feed restriction on growth characteristics of male broiler chickens. Poult Sci. 81:679–688.
- Van Nieuwenhoven FA, Verstijnen CPHJ, Abumrad NA, Willemsen PHM, Vaneys GJJM, Vandervusse GJ, Glatz JFC. 1995. Putative membrane fatty acid translocase and cytoplasmic fatty-acid-binding protein are co-expressed in rat heart and skeletal muscle. Biochim Biophys Res Commun. 207:747–752.
- Veerkamp JH, Van Moerkerk HTB. 1993. Fatty acid-binding protein and its relation to fatty acid oxidation. Mol Cell Biochem. 123:101–106.
- Venkatesan N, Lim J, Bouch C, Marciano D, Davidson MB. 1996. Dexamethasone-induced impairment in skeletal muscle glucose transport is not reversed by inhibition of free fatty acid oxidation. Metabolism. 45:92–100.
- Villena JA, Roy S, Sarkadi-Nagy E, Kim KH, Sul HS. 2004. Desnutrin, an adipocyte gene encoding a novel patatin domain-containing protein, is induced by fasting and glucocorticoids: Ectopic expression of desnutrin increases triglyceride hydrolysis. J Biol Chem. 279:47066–47075.
- Wang XJ, Lin H, Song ZG, Jiao HC. 2010. Dexamethasone facilitates lipid accumulation and mild feed restriction improves fatty acids oxidation in skeletal muscle of broiler chicks (Gallus gallus domesticus). Comp Biochem Physiol. 151:C447–C454.
- Watt MJ, van Denderen BJ, Castelli LA, Bruce CR, Hoy AJ, Kraegen EW, Macaulay L, Kemp BE. 2008. Adipose triglyceride lipase regulation of skeletal muscle lipid metabolism and insulin responsiveness. Mol Endocrinol. 22:1200–1212.
- Weibel ER. 1973. Stereological techniques for electron microscopic morphometry. In: Hayat MA. editors. Principles and techniques of electronmicroscopy biological application. New York: Van Nostrand Rheinhold237–296.
- Whorwood CB, Donovan SJ, Flanagan D, Phillips DIW, Byrne CD. 2002. Increased glucocorticoid receptor expression in human skeletal muscle cells may contribute to the pathogenesis of the metabolic syndrome. Diabetes. 51:1066–1075.
- Winder WW, Hardie DG. 1996. Inactivation of acetyl-CoA carboxylase and activation of AMP-activated protein kinase in muscle during exercise. Am J Physiol. 270:E299–E304.
- Ye JM, Ruderman NB, Kraegen EW. 2005. AMP-activated protein kinase and malonyl-CoA: Targets for treating insulin resistance?. Drug Discov Today Ther Strateg. 2:157–163.
- Yuan L, Lin H, Jiang KJ, Jiao HC, Song ZG. 2008. Corticosterone administration and high-energy feed results in enhanced fat accumulation and insulin resistance in broiler chickens. Br Poult Sci. 49:487–495.
- Zerehdaran S, Vereijken ALJ, van Arendonk JAM, van der Waaijt EH. 2004. Estimation of genetic parameters for fat deposition and carcass traits in broilers. Poult Sci. 83:521–525.
- Zhao JP, Lin H, Jiao HC, Song ZG. Corticosterone suppresses insulin- and NO-stimulated muscle glucose uptake in broiler chickens (Gallus gallus domesticus). Comp Biochem Physiol. 2009a; 149:C448–C454.
- Zhao JP, Jiao HC, Song ZG, Lin H. Effects of l-arginine supplementation on glucose and nitric oxide (NO) levels and activity of NO synthase in corticosterone-challenged broiler chickens (Gallus gallus). Comp Biochem Physiol. 2009b; 150:474–480.
- Zimmermann R, Strauss JG, Haemmerle G, Schoiswohl G, Birner-Gruenberger R, Riederer M, Lass A, Neuberger G, Eisenhaber F, Hermetter A, Zechner R. 2004. Fat mobilization in adipose tissue is promoted by adipose triglyceride lipase. Science. 306:1383–1386.