Abstract
We investigated the association of systemic and local tissue stress responses with heat–tolerant (TOL) levels in mice. Thirty-eight mice were assigned into control and three heat exposure groups—TOL, moderately tolerant, and intolerant (INT), based on their overall thermal responses. Real-time core temperature, blood pressure, and heart rate (HR) were assessed during heat exposure (39.5°C) under conscious condition. Tissue samples were collected 18–22 h following heat exposure. INT mice had significantly higher peak mean arterial pressure and HR than TOL mice during heat exposure. Plasma corticosterone levels were significantly higher in INT than in control mice. No significant changes in plasma cytokines or markers of oxidative status were observed. INT mice showed significant increases in HSP72 and HSP90 protein and mRNA levels in liver, heart, and gastrocnemius muscles compared to TOL and control mice. In contrast, INT mice had significantly lower heat shock factor 1 and glucocorticoid receptor protein and mRNA levels in these tissues than TOL and control mice. These results indicate that acute heat exposure induces stress responses in various tissues of INT mice, but not TOL mice. Upregulation of stress proteins by acute heat exposure involves both transcriptional and translational pathways.
Introduction
Exposure to high temperatures may cause illness and even heat stroke in some people (Bouchama and Knochel Citation2002). Identifying heat-intolerant (INT) individuals will help prevent injuries and possible life-threatening complications especially during various physical activities in warm or hot environments. Unfortunately, physiological characteristics of heat intolerance remain undefined. Several studies have investigated stress-related responses to heat exposure in animals and humans and shown that heat intolerance is indeed associated with an altered stress response. However, the results are inconsistent. For example, Michel et al. (Citation2007) reported that heat-INT rats demonstrated activation of the hypothalamic–pituitary–adrenocortical axis as characterized by increased stress and inflammatory changes. The response included elevations in mRNA levels for cytokines and heat shock protein 70 (HSP70) in pituitary and hypothalamic regions immediately following heat exposure in heat-INT as compared to heat-tolerant (TOL) rats. In contrast, Moran et al. (Citation2006) showed inadequate stress responses in heat-INT compared to heat-TOL individuals; differences included a lower expression of HSP72, an inducible member of the HSP70 family, and heat shock factor 1 (HSF1) in lymphocytes 1 h following heat exposure. Reasons for the discrepancy between the heat stress responses of the brain and blood cells remain unclear. Information on heat-induced changes in additional tissues/organs is needed to determine stress-related alterations associated with heat intolerance, since responses of different tissues or organs to heat may vary markedly (Flanagan et al. Citation1995; Ruell et al. Citation2004).
The objective of this study was to determine the association between heat-induced stress responses and heat tolerance levels in mice. Our hypothesis was that heat exposure induces more extensive stress responses in heat-INT than heat-TOL mice. We examined stress-related responses in mice during and following heat exposure. During heat exposure, real-time thermal and cardiovascular changes were assessed in conscious mice. Specifically, stress-related changes in proteins and protein transcription were evaluated in multiple organs 18–22 h following heat exposure. INT mice showed a significantly higher cardiovascular workload than TOL mice during heat exposure. Moreover, INT, but not TOL, mice had significant upregulation of stress proteins in all tested tissues/organs. Acute heat exposure induced stress responses at both the transcriptional and translational levels in INT mice.
Methods
Animals
The experiments were conducted using 38 adult male C57BL/6J mice (Jackson Laboratories, Bar Harbor, ME, USA). The mice were 12–16 weeks old and weighed 23–29 g when tests were performed. They were maintained in conventional animal facilities (∼21°C) with ad libitum food and water at the Uniformed Services University Laboratory Animal Medicine facility. All procedures performed on animals were approved by the Uniformed Services University Institutional Animal Care and Use Committee.
All experimental mice were surgically implanted with a temperature transponder (Model G2 E-Mitter, Mini Mitter Corp., Bend, OR, USA) and a blood pressure (BP) transmitter (Model PA-C10, Data Science International, DSI, St. Paul, MN, USA). The temperature transponder was placed in the abdominal cavity via a mid-line abdominal incision. The BP transmitter catheter was placed into the left carotid artery, and the transmitter body was tunneled subcutaneously to a small pouch along the right-ventral flank. All instrumentation was performed with the use of aseptic surgical procedures under anesthesia ( ≤ 2% isoflurane in 100% oxygen). Together, the two implants weighed ∼2.5 g. Sham implants were installed in control mice (n = 10). At least 2 weeks were allowed for recovery. At the time of the experimental protocols, all mice were healthy as evidenced by body weight gains ( ≥ presurgical levels), normal activity, and no sign of infection.
Experimental procedure
Heat tests were conducted in an environmental chamber (Model 3950, Thermo Forma, Marietta, OH, USA). Mice were placed in the chamber at ∼21°C (relative humidity: ∼22–30%) a day before experimentation to minimize stress-induced hyperthermia (Leon et al. Citation2005). Heat exposures began the following morning after stable baseline data were obtained. Food and water were removed from cages before exposure. We used a heat protocol modified from the laboratory of Michel et al. (Citation2007) such that heat exposure continued a maximum of 3 h, including ∼1 h of heating the chamber to a predetermined temperature (39.5 ± 0.2°C). Heat exposure was terminated at core temperature (Tc) of ∼42.4°C or 3 h into heat exposure, whichever happened first. Based on our preliminary data (not shown), this protocol allowed sufficient heat exposure to test sensitivity to heat stress without any risk to the animals. Some INT mice reached 42.4°C before 3 h of heat exposure. Thus, all INT mice had a hyperthermic response to the same or shorter duration of heat exposure as compared to other mice. Upon completion, the chamber door was immediately opened and fresh air was supplied through an external fan. The chamber temperature rapidly dropped to below 25°C within ∼15 min. Each animal underwent two heat tests separated by an interval of 7 days. All control mice remained in their housing units and were not exposed to heat.
Real-time Tc BP and heart rate (HR) were recorded continuously during experiments. Mice were housed individually and up to two mice were tested each time. The two telemetry systems worked simultaneously in a sandwich layout, with the pressure receiver on the top of the cage and the temperature receiver under the cage. The pressure receiver was placed upside down to facilitate radio signal reception. An open plastic insert was added between the pressure receiver and the mouse cage lid to assure sufficient air ventilation and rapid temperature equilibration in the cage. The pressure receiver was ∼30 cm from the bottom of the cage, a distance to allow high-quality signal transmission over the entire cage range without interference between the two telemetry systems.
Custom applications written in LabView 8.6 (National Instruments, Austin, TX, USA) with a Windows XP desktop computer were used to control and synchronize the recording process of the two telemetry systems. The temperature data acquisition with LabView was established using the communication protocols provided by Mini Mitter Corp. Under dynamic mode analysis, the DSI telemetry system can calculate and export the dual-channel pressure parameters, HR and activities to Microsoft Excel every 10 s. The DSI-exported data (active Excel) were temporarily stored in internal memory and updated every 10 s. Microsoft provides the ActiveX components for Excel to make the spreadsheets fully accessible from external applications. LabView offers support for ActiveX automation as a server as well as support for ActiveX Containers and ActiveX Events. The real-time data transfer from the active Excel worksheet to the LabView was achieved by using a callback for an ActiveX event. The LabView applications provide an interface to process and display the real-time Tc, HR, systolic BP (SBP), diastolic BP (DBP), mean arterial pressure (MAP), activity obtained from the telemetry systems, chamber temperature, and relative humidity (HumidiProbe, Pico Technology, Eaton Socon, UK). These data were obtained and displayed every 10 s and recorded every 60 s.
Collection and processing of blood and tissues
Collection of blood and tissues was performed under anesthesia ∼18–22 h following the last heat test. Blood, taken from a carotid artery, was collected in a heparin-primed tube and immediately centrifuged to obtain plasma. Subsequently, the liver, kidneys, heart (left ventricle), and gastrocnemius and soleus muscles were removed, cleaned in ice-cold phosphate-buffered saline (PBS), frozen immediately in liquid nitrogen, and stored at − 80°C.
Tissue samples were homogenized and further processed before analyses. Briefly, frozen tissues were placed into polypropylene test tubes containing 1 ml of ice-cold PBS and homogenized (5–10 s) with a Tissue-Tearor homogenizer (Bartlesville, OK, USA). The Tissue-Tearor was cleaned in a series of fresh PBS-filled beakers before homogenizing each sample tissue. Homogenates were centrifuged at 14,000 rpm for 3 min. The supernatants were obtained and placed into new 1.5 ml Eppendorf tubes and stored at − 80°C. The remaining pellets were evaporated to dryness and weighed for data correction.
Plasma assays
Cytokine assays were performed in duplicate by using the MasterPlex QT System (Luminex, Austin, TX, USA). The MasterPlex QT System incorporated a fluorescent multi-analyte profiling bead immunoassay specific for murine cytokines. A 20-plex (FGF basic, GM-CSF, IFN-γ, IL-1α, IL-1β, IL-2, IL-4, IL-5, IL-6, IL-10, IL-12p40p70, IL-13, IL-17, IP-10, KC, MCP-1, MIG, MIP-1α, TNF-α, and VEGF) cytokine bead-based kit (Invitrogen Corp., Carlsbad, CA, USA) was used to screen selective samples. A custom 6-plex cytokine panel was designed (Affymetrix, Inc., Santa Clara, CA, USA) to analyze IL-1β/IL-1F2, IL-6, IL10/CSIF, IL-12/IL-23 p40, IL-12 p70, and VEGF-A in each 25 μl plasma sample. Sensitivity of the assay was 2 pg/ml per cytokine.
Plasma samples (50 μl) were analyzed for corticosterone and 8-isoprostane individually in duplicate samples by commercial enzyme-linked immunosorbant assay (ELISA) kits specific for murine samples (Cayman, Ann Arbor, MI, USA) with the Magellan Data Analysis System (Tecan, Grodig, Austria). Sensitivity of the assays was 30 and 2 pg/ml, respectively.
Plasma superoxide dismutase (SOD) capacity was determined based on the inhibition of superoxide anion produced by xanthine oxidase (Hapner et al. Citation2010). Lucigenin (5 μM)-enhanced chemiluminescence was used to assess changes in xanthine oxidase-dependent superoxide. The reaction was initiated by adding xanthine (100 μM) to PBS solutions containing xanthine oxidase and plasma samples in a tube luminometer (Berthold AutoLumat Plus LB 953) at room temperature. The chemiluminescence signal was adjusted to background and continuously measured at 2 Hz. The measurements were performed in duplicate. Inhibition of superoxide was calculated as relative reductions in chemiluminescence, and the plasma SOD activity was calculated from a standard curve of inhibition of superoxide by Cu/Zn-SOD (Sigma-Aldrich, St. Louis, MO, USA). All plasma samples were run in the same assays to avoid inter-assay variability.
Enzyme-linked immunosorbant assay
Tissue homogenate supernatant and plasma samples were measured in duplicates using commercial ELISA kits sensitive to murine samples. The following ELISA kits were used for HSP72 (Stressgen, Ann Arbor, MI, USA), HSP90 (TSZ ELISA, Framingham, MA, USA), glucocorticoid receptor (GR) (TSZ ELISA), and HSF1 (Enzo Life Sciences, Plymouth, PA, USA) as per the manufacturers' instructions. The aspirating and washing cycles were completed by using an automatic microplate washer (Tecan Group Ltd., Männedorf, Switzerland). Samples were analyzed using the Magellan Data Analysis System (Tecan) and normalized to dry tissue weight (dw). Sensitivity of the assays were 35, 200, 10, and 60 pg/ml, respectively. Intra- and inter-assay coefficients of variation for ELISA concentrations were less than 5% per assay.
Quantitative Rt-pcr
RNA was isolated from tissue using RNeasy Mini Kit and QIAshredder (Qiagen, Valencia, CA, USA), and cDNA templates were prepared with the Maloney murine leukemia virus reverse transcriptase-directed iScript One-Step RT-PCR system (Hercules, CA, USA). The following primers were synthesized by the Genomics Core at the Biomedical Instrumentation Center (USUHS, MD, USA) and utilized for RT-PCR: HSF1 (5-CCTGGCCCATACTCAGCTC-3 and 5-CTCTTGCTTGACACGGACC-3), HSP72 (5-CTCCCTCTTGCGTTGCCTC-3 and 5-ACCCGCAGTAATAGCCATCTG-3), HSP90 (5-GACGCTCTGGATAAAATCCGTT-3 and 5-TGGGAATGAGATTGATGTGCAG-3), GR (5-AGCTCCCCCTGGTAGAGAC-3 and 5-GGTGAAGACGCAGAAACCTTG-3), and glyceraldehyde-3-phosphate dehydrogenase (GAPDH) (5-TGACAACTTTGGTATCGTGGAAGG-3 and 5-AGGGATGATGTTCTGGAGAGCC-3). RT-PCR was performed for 40 cycles using the Bio-Rad iCycler iQ RT-PCR thermocycler and iScript SYBR green PCR supermix (Hercules, CA, USA). Quantification of the RT-PCR products normalized to GAPDH expression was performed using iCycler iQ data analysis software.
Data processing and statistical analysis
Data are expressed as mean ± SEM. Baseline, peak, and recovery values of Tc, BPs, and HR were calculated as steady-state averages before heat exposure, during the last 5 min of heat exposure and during a lowest Tc period following heat exposure, respectively. A heat stress index (HSI) was calculated as the sum of the product of peak Tc and rate to peak from baseline in two heat tests: HSI = peak Tc (heat 1) × rate (heat 1) + peak Tc (heat 2) × rate (heat 2). The HSI was used to assess the level of heat tolerance. One-way ANOVA with Dunnett's post hoc tests and two-way ANOVA with Bonferroni's post hoc tests were used for comparison. The results were considered significant at P ≤ 0.05.
Results
Thermal responses during heat exposure
Twenty-eight mice exposed to heat were assigned to one of three groups: TOL, moderately tolerant (MT), and INT to reflect their level of heat tolerance based on thermal responses during the two heat tests. presents baseline and peak Tc, rate to peak Tc, and recovery from exposures to Heat 1 and Heat 2 tests. Mice with a HSI of < 1.800 were placed in TOL group (n = 10), those with a HSI of >2.500 in INT group (n = 10), and the remaining mice in MT group (n = 8). INT mice had a higher peak Tc and faster hyperthermic rate than TOL or MT mice during each heat experiment. INT mice also showed a greater hypothermic response than TOL or MT mice following the first heat exposure. No differences in recovery Tc were noted among the three groups following the second heat exposure.
Table I. Thermal responses of TOL, MT, and INT mice in two heat tests.
Cardiovascular responses during heat exposure
shows typical Tc, SBP, DBP, and HR changes in an INT mouse in response to heat exposure. All mice experienced two steady states of Tc, one around the physiological temperature 37.0°C followed by the other around the chamber set temperature 39.5°C (actual peak temperature measured in mouse cage using a E-Mitter transponder: 39.6–39.7°C) during heat exposure. However, Tc in INT mice increased earlier and faster from the second steady state than in TOL and MT mice (not shown). Correspondingly, SBP, DBP, and HR in INT mice increased markedly during the late stage of heat exposure. As shown in , heat exposure resulted in significantly higher peak MAP during heat exposure than their pre-exposure, baseline levels (F (2, 52) = 35.48, P < 0.0001). Moreover, post hoc analysis revealed significantly higher peak MAP (P < 0.01) during exposure for INT mice compared to TOL and MT mice. A significant overall group effect was also noted for HR during heat exposure (F (2, 53) = 5.27, P = 0.008) and post hoc analysis revealed a significantly higher peak HR in INT mice (P < 0.05). No significant differences in MAP or HR were found during recovery compared to baseline.
Changes in plasma variables following heat exposure
Among the tested cytokines, only IL-6, IL-12, and VEGF were consistently detectable in the plasma samples collected 18–22 h following the second heat exposure. No significant differences were noted for these cytokines among control, TOL (n = 10), MT (n = 8), and INT mice (n = 10; ). Similarly, no significant differences in plasma 8-isoprostane or SOD were found across the four groups. In contrast, plasma corticosterone levels following heat exposure were significantly higher (F = 3.02, P < 0.05) in INT mice compared to the control group, but not relative to TOL or MT mice.
Table II. Plasma cytokines, 8-isoprostane, corticosterone, and SOD in control, TOL, MT, and INT mice.
Changes in tissue HSP72 following heat exposure
Overall, kidneys showed higher basal levels of HSP72 than liver, heart, or gastrocnemius and soleus muscles under control conditions (F = 59.4, P < 0.0001, ). ANOVA revealed a main group effect for each tissue. Heat exposure induced significant increases in HSP72 in the kidneys in TOL (P < 0.05, n = 10), MT (P < 0.01, n = 8), and INT mice (P < 0.001, n = 10) compared to control mice (n = 10). INT mice (n = 10) also showed significant increases in HSP72 for all other tested organs/tissues including liver (P < 0.005), heart (P < 0.001), and gastrocnemius (P < 0.001) and soleus muscles (P < 0.01). An increase in HSP72 was found only in the soleus muscle of MT mice (P < 0.05, n = 8); no other tested organs/tissues of TOL mice exhibited increases in HSP72. The HSP72 levels in the heart, gastrocnemius muscle, soleus muscle, liver, and kidney of INT mice were ∼7.5, 3.6, 2.1, 0.9, and 0.8 fold higher, respectively, than for TOL mice. Plasma HSP72 levels in the control and heat-exposed mice were below the limit of detection.
Figure 3. Tissue HSP72 levels in control mice and in TOL, MT, and INT mice following heat stress. One-way ANOVA revealed significant (F (3, 37) = 4.9, 16.3, 8.7, 11.6, and 27; P = 0.006, 0.0001, 0.0002, 0.0001, and 0.0001 for liver, kidney, heart, gastrocnemius muscle, and soleus muscle, respectively). P < 0.05: *compared to control; †compared to TOL; #compared to MT.
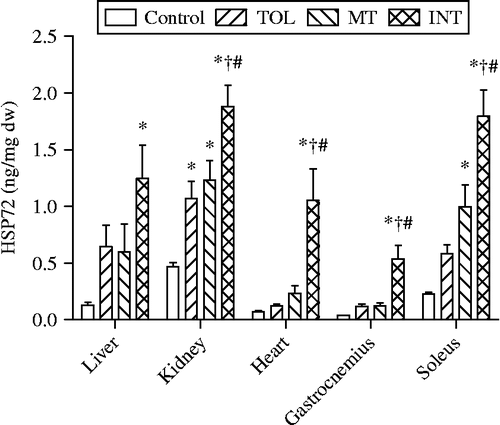
Tissue HSP90, GR, and HSF1 following heat exposure
Protein levels of HSP90, GR, and HSF1 were compared in liver, heart, and gastrocnemius muscle from TOL (n = 10) and INT mice (n = 10) to characterize stress-related changes associated with heat intolerance (), while MT mice were not further investigated. Overall significant group effects were noted for HSP90 in liver (F (2, 17) = 106, P < 0.0001), heart (F (2, 17) = 115, P < 0.0001), and gastrocnemius muscle (F (2, 17) = 181, P < 0.0001); GR in liver (F (2, 17) = 94, P < 0.0001), heart (F (2, 17) = 20, P < 0.0001), and gastrocnemius muscle (F (2, 17) = 22, P < 0.0001); and HSF1 in liver (F (2, 17) = 24, P < 0.0001), heart (F (2, 17) = 20, P < 0.0001), and gastrocnemius muscle (F (2, 17) = 32, P < 0.0001). Post hoc analysis revealed that INT mice had significantly higher HSP90 protein levels in liver, heart, and gastrocnemius muscle, respectively (79%, 66%, and 79%, P < 0.001), compared to TOL mice. In contrast, INT mice had significantly lower HSF1 and GR protein levels (HSF1: 23%, 32%, and 44%, P < 0.01; GR: 33%, 39%, and 40%, P < 0.01) in liver, heart, and gastrocnemius muscle, respectively, compared to TOL mice. No significant differences in HSP90, GR, or HSF1 protein expression were observed between TOL and control mice (n = 10) in these tissues.
Figure 4. HSP90, GR, and HSF1 protein levels in liver, heart, and gastrocnemius muscle tissues of control mice and TOL and INT mice following heat stress. *P < 0.05, compared to control and TOL.
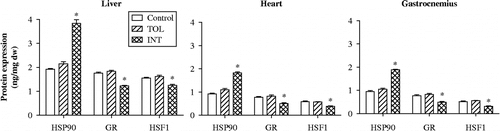
This pattern was also observed for mRNA levels as shown in . Overall there were significant group effects on the mRNA levels of HSP72 in liver (F (2, 17) = 96, P < 0.0001), heart (F (2, 17) = 161, P < 0.0001), and gastrocnemius muscle (F (2, 17) = 224, P < 0.0001); HSP90 in liver (F (2, 17) = 25, P < 0.0001), heart (F (2, 17) = 54, P < 0.0001), and gastrocnemius muscle (F (2, 17) = 130, P < 0.0001); GR in liver (F (2, 17) = 46, P < 0.0001), heart (F (2, 17) = 15, P = 0.0003), and gastrocnemius muscle (F (2, 17) = 23, P < 0.0001); and HSF1 in liver (F (2, 17) = 26, P < 0.0001), heart (F (2, 17) = 23, P < 0.0001), and gastrocnemius muscle (F (2, 17) = 20, P < 0.0001). Post hoc analysis revealed that INT mice had significantly higher HSP72 and HSP90 mRNA levels (HSP72: 145%, 199%, and 286%, P < 0.05; HSP90: 63%, 107%, and 171%, P < 0.05) in liver, heart, and gastrocnemius muscle, respectively, compared to TOL mice. Furthermore, INT mice had significantly lower HSF1 and GR mRNA levels (HSF1: 58%, 47%, and 53%, P < 0.05; GR: 63%, 32%, and 55%, P < 0.05) in liver, heart, and gastrocnemius muscle tissues, respectively, compared to TOL mice. Again no significant changes in HSP72, HSP90, HSF1, or GR mRNA expression were observed between TOL and control mice.
Discussion
We have characterized immediate and prolonged stress responses of mice to heat exposure as a function of heat intolerance. Individual differences in heat sensitivity have been reported previously in rodents (Ohara et al. Citation1975; Wright Citation1976; Wright et al. Citation1977; Michel et al. Citation2007), and in this study we further demonstrated that this wide range of individual heat sensitivities is relatively stable within mice, as shown in two repeated heat tests. The reduced heat tolerance in INT mice could not be explained by their health and physical properties such as body mass (Epstein Citation1990; Havenith Citation2001), as all mice were matched for age, gender, body weight, and genetic background. The vulnerability of INT mice to heat was revealed in acute as well as delayed heat-induced changes. Specifically, INT mice featured severe hyperthermia and heavy cardiovascular workload that were not observed in TOL mice during heat exposure. The effect of heat stress was extensive and sustained in INT mice as evidenced by elevated corticosterone in plasma and HSP72 and HSP90 in multi-organs/tissues 18–22 h following heat exposure. It is likely these heat-induced changes involve pathways at both transcriptional and translational levels.
The cardiovascular system serves an important role in thermoregulation during heat stress, and changes in cardiovascular activity typically reflect the degree of stress (Bouchama and Knochel Citation2002). Previous studies have shown that both BP and HR increase significantly in anesthetized rats during induction of heat stroke, but then drop rapidly upon development of heat stroke (Niu et al. Citation2003; Wang et al. Citation2005). Heat-vulnerable individuals are likely to demonstrate marked cardiovascular loads and impaired cardiovascular compensatory responses during heat stress (Bouchama and Knochel Citation2002; Wilson and Crandall Citation2011). Cardiovascular activities have not been assessed previously in conscious mice during heat exposure. Our findings suggest that INT mice experience greater cardiovascular stress during heat exposure than TOL mice as evidenced by significantly higher BP and HR at peak Tc. Interestingly, Tc, BP, and HR seem to exhibit two steady states in conscious mice, one around the physiological temperature 37.0°C and the other around chamber temperature 39.5°C (). In contrast, these steady states appear to be missing in anesthetized animals during heat exposure (Chang et al. Citation2003; Niu et al. Citation2003).
Heat-induced hyperthermia is associated with inflammation (Katschinski et al. Citation1999) and cellular oxidative stress (Kim et al. Citation2010). However, no changes in blood cytokines or oxidative status were found in our study 18–22 h following heat exposure. The patterns of change in plasma cytokines after animals are exposed to heat are unclear (Leon Citation2002). Leon et al. reported that most cytokines tested, except IL-6, returned to baseline levels 24 h after heat stress (Leon et al. Citation2006). Patterns of changes in plasma VEGF with heat-induced hyperthermia remain undefined, although it is widely tested as a marker for infection-induced fever (Tseng et al. Citation2005; Alves et al. Citation2011). In contrast, tissue VEGF has been shown to increase gradually and reach its peak around 24 h following brief heat-induced whole body hyperthermia (15 min at Tc of 42°C; Gong et al. Citation2006). Our inability to detect differences in plasma cytokines could reflect the timing of our blood collection. Also we collected blood samples via an intravascular catheter instead of cardiac puncture (Leon et al. Citation2006; Michel et al. Citation2007). It is possible that our blood collection technique might also have contributed to the low levels of plasma cytokines. Additional work would be required to confirm these issues. Cytokines have been implicated in heat-induced hyperthermia (Katschinski et al. Citation1999; Leon Citation2002), but the dynamics and patterns of change in cellular cytokines associated with hyperthermic intensity remain unclear. Our results suggest that plasma cytokines should not be used as sole markers to assess heat stress.
Heat-induced hyperthermia elicits a stress response, as indicated by increases in plasma corticosterone in rats (Michel et al. Citation2007) and mice (Leon et al. Citation2006) immediately following heat stress. In this study, INT mice had significantly elevated plasma corticosterone levels as compared to the other groups 18–22 h following heat exposure, which seems not consistent with Leon et al. (Citation2006) who noted that corticosterone levels returned to baseline 24 h after heat test. However, in their study, corticosterone values were not examined based on the heat tolerance classification of the mice. In our study, neither TOL nor MT mice had increased plasma corticosterone levels following heat exposure.
Dehydration or reduced plasma volume is unlikely to explain the elevated corticosterone in INT mice as all heat-treated mice had recovered lost body weight (∼4–7% immediately after heat) at time of sacrifice. Interestingly, Michel et al. reported a greater increase in plasma corticosterone in heat-TOL rats than in heat-exhausted rats immediately following heat exposure, but no follow-up results were reported (Michel et al. Citation2007). Thus, timings in the measurement of corticosterone may be important. There has been considerable interest in using plasma parameters to identify biologic markers of heat stress in humans. Our results support previous findings that plasma corticosterone may become one potential biomarker to detect heat intolerance. However, heat intolerance should not be based solely on a single biologic marker due to the complexity of heat stress and thermoregulation.
Upregulation of HSPs, in particular HSP72, the inducible isoform of the HSP70 family, is one of the primary cellular defense mechanisms against heat stress (Mizzen and Welch Citation1988). Induction of HSP72 synthesis seems to occur only in organs undergoing stress (Flanagan et al. Citation1995), and internal organs/tissues also have different vulnerability to heat injury (Ritchie et al. Citation1994). These may partially explain why HSP72 levels were increased selectively and differentially in various organs/tissues among TOL, MT, and INT mice. Differential HSP72 induction in organs or tissues by hyperthermia remains inconsistent in the literature (Hotchkiss et al. Citation1993; Flanagan et al. Citation1995; Ruell et al. Citation2004). Hotchkiss et al. (Citation1993) reported that gut of mice had the highest expression of HSP72, followed by lung, liver, kidney heart, brain, and abdominal muscle 12 h after brief heat-induced hyperthermia. Similarly, Flanagan et al. (Citation1995) found that HSP72 content increased in liver, gut, and kidney, but not in brain or quadriceps muscles of anesthetized rats. Ruell et al. reported that HSP72 content was significantly elevated in the heart but not in liver and gut of rats exposed to 40°C for 1 h; however, significant induction was noted in all the three tissues of rats exposed to 42°C (Ruell et al. Citation2004). These discrepancies among these studies (including this study) are, in part, due to experimental conditions, such as different target Tc and duration of hyperthermia (Hotchkiss et al. Citation1993; Flanagan et al. Citation1995; Ruell et al. Citation2004). However, such conditions do not explain why differential expression would occur in the same tissue among mice when stratified by heat tolerance. This remains an issue for further investigation.
Interestingly, the kidney had the highest basal HSP72 concentrations among the tested organs/tissues and was also the only organ showing increases in HSP72 in all heat-exposed mice. The precise mechanism underlying high renal HSP72 content is unclear, but may reflect the inherently unfavorable environment within the kidney (Neuhofer and Beck Citation2005), such as constant exposure to hypoxic (Epstein Citation1985; Welch et al. Citation2001), osmotic, and pH challenges (Garcia-Perez and Burg Citation1991; Neuhofer and Beck Citation2005). Elevations in Tc are also associated with increased renal sympathetic nerve activity and reduced renal blood flow (Badoer Citation2010). This inherent sensitivity may explain why HSP72 increased in the kidneys of all heat-exposed mice.
We also demonstrated that heat intolerance in mice is associated with specific and late increases in the expression of both mRNA and protein levels of HSP72 and HSP90 in major organs/tissues. However, INT mice also had a specific decrease in the mRNA expression and protein levels of HSF1 and GR in liver, heart, and gastrocnemius muscle, compared to TOL mice. This was unexpected given HSF1 is constitutively expressed in most tissues and cell types and appears to be regulated mainly via post-translational mechanisms (Wu Citation1995). Following activation by heat stress, HSF1 typically binds to specific heat shock elements to induce HSP gene expression (Trinklein et al. Citation2004; Anckar and Sistonen Citation2007). If so, then one would expect heat-sensitive tissues to express higher levels of HSF1 rather than lower levels. As HSF1 is the transcription factor for both HSP72 and HSP90, this could infer a novel gene-specific regulation of HSF1 in INT mice as opposed to TOL mice. Although distinct SNPs in the HSF1 gene were reported to be associated with thermal tolerance in cattle (Li et al. Citation2011), this possibility has not been examined in mice. INT mice showed significant elevations in plasma corticosterone and reductions in tissue GR protein and mRNA levels 18–22 h following heat exposure, and downregulation of GR protein and mRNA has been shown to occur after exposure to high concentrations of glucocorticoid within 24 h (Cidlowski and Cidlowski Citation1981; Silva et al. Citation1994). The physiological implication of this altered hormone–receptor activity in heat intolerance remains unclear and will require further investigation. Complex relationships between GR and HSF1 have been reported in previous studies, and it is understood that GR exerts inhibitory control over HSF1 activation specifically mediated by heat stress (Jones et al. Citation2004; Wadekar et al. Citation2004). Dysfunction in these pathways could potentially account for the parallel decrease in GR and HSF1 expression in INT mice. Further detailed investigations into these complex pathways regulating the HSF1 gene are needed to fully characterize heat intolerance in mice.
Several limitations regarding this study need to be acknowledged. First, we determined heat tolerance based on peak Tc and rate of rise in Tc during heat exposure. Two types of heat protocols are commonly used to assess heat tolerance in animals, targeted peak Tc or fixed exposure duration. In the heat protocols with targeted peak Tc, exposure duration or heat load (area under curve) is used to determine heat tolerance (Furuyama et al. Citation2003). That is, the shorter duration or the smaller heat load, the more severe the heat intolerance. In heat protocols with fixed duration, peak Tc is primarily used to determine heat tolerance levels (Lin and Chai Citation1975; Szelenyi et al. Citation2004; Michel et al. Citation2007). That is, the higher peak Tc, the more severe the heat intolerance. In these fixed duration protocols, a maximal peak Tc is usually chosen as an early endpoint to prevent heat injury or stroke (Szelenyi et al. Citation2004). We used a fixed duration heat protocol in this study. All INT mice had a significantly higher peak Tc before or at end of heat exposure (). Second, we used a HSI, which included the peak Tc and rate of rise in Tc, to classify mice into TOL, MT, and INT groups. The cut-off points were selected to obtain similar numbers of mice among the three groups. The average peak Tc in INT mice was similar to that reported in heat-INT rats exposed at 40°C for 90 min (Michel et al. Citation2007) though studies on heat-INT mice remain unavailable. Both the thermal and cardiovascular responses support that INT mice were different from others in heat tolerance and stress levels. Third, baseline Tc values were not consistent in this study as shown in . The reason for these variations remains unknown, but does not seem to reflect differences in acclimation or the experimental setting among TOL, MT, and INT mice. All mice were placed in the chamber the night before each experiment, and all heat tests started in the morning around 9:00 am. Also a trend of a lower baseline Tc in Heat 2 than in Heat 1 was noted, which may reflect improved acclimation in all mice. Importantly, peak Tc between the two heat tests was very similar within each group, suggesting that heat tolerance may not be affected by basal Tc. Finally, tissue and blood samples were only assessed at a single time point (18–22 h after the second heat exposure). Thus, we cannot rule out potential alterations in many of the undetected or unchanged biochemical parameters at any other time following heat exposure. Within the confines of these limitations, the data clearly indicate that INT mice experienced a more robust response to heat stress than TOL and MT mice.
In conclusion, we have identified a subset of age- and gender-matched mice from the same strain that is INT. INT mice were characterized by severe hyperthermia, intensive cardiovascular activity, and extensive tissue stress in response to acute heat exposure; these responses were not observed in TOL mice. Tissue stress was associated with release of glucocorticoids and induction of HSPs, which resulted in downregulation of GR and upregulation of HSPs at both protein and mRNA levels. INT mice also showed another novel feature of the reduced HSF1 protein and mRNA levels. Genetic comparisons between INT and TOL mice for HSF1 regulation of HSPs would merit further investigation as it may provide insight into thermoregulatory compromise or help identify individuals with thermoregulatory limitations.
Acknowledgements
The authors would like to thank Dr. Ling-Ling Tsai of National Chung-Cheng University for her assistance programing the Mini Mitter communication and Adrianna Levesque for her assistance in data analysis. This work was supported by Uniformed Services University of the Health Sciences Intramural Grant R091EH and the Office of Naval Research Grant N0001411MP20025.
Declaration of interest: The authors report no conflicts of interest. The authors alone are responsible for the content and writing of the paper.
References
- Alves BE, Montalvao SA, Aranha FJ, Lorand-Metze I, De Souza CA, Annichino-Bizzacchi JM, De Paula EV. 2011. Time-course of sFlt-1 and VEGF-A release in neutropenic patients with sepsis and septic shock: A prospective study. J Transl Med. 9:23.
- Anckar J, Sistonen L. 2007. Heat shock factor 1 as a coordinator of stress and developmental pathways. Adv Exp Med Biol. 594:78–88.
- Badoer E. 2010. Role of the hypothalamic PVN in the regulation of renal sympathetic nerve activity and blood flow during hyperthermia and in heart failure. Am J Physiol Renal Physiol. 298:F839–F846.
- Bouchama A, Knochel JP. 2002. Heat stroke. N Engl J Med. 346:1978–1988.
- Chang CP, Hsu YC, Lin MT. 2003. Magnolol protects against cerebral ischaemic injury of rat heatstroke. Clin Exp Pharmacol Physiol. 30:387–392.
- Cidlowski JA, Cidlowski NB. 1981. Regulation of glucocorticoid receptors by glucocorticoids in cultured HeLa S3 cells. Endocrinology. 109:1975–1982.
- Epstein FH. 1985. Hypoxia of the renal medulla. Q J Med. 57:807–810.
- Epstein Y. 1990. Heat intolerance: Predisposing factor or residual injury?. Med Sci Sports Exerc. 22:29–35.
- Flanagan SW, Ryan AJ, Gisolfi CV, Moseley PL. 1995. Tissue-specific HSP70 response in animals undergoing heat stress. Am J Physiol. 268:R28–R32.
- Furuyama F, Murakami M, Tanaka E, Hida H, Miyazawa D, Oiwa T, Isobe Y, Nishino H. 2003. Regulation mode of evaporative cooling underlying a strategy of the heat-tolerant FOK rat for enduring ambient heat. Am J Physiol Regul Integr Comp Physiol. 285:R1439–R1445.
- Garcia-Perez A, Burg MB. 1991. Renal medullary organic osmolytes. Physiol Rev. 71:1081–1115.
- Gong B, Asimakis GK, Chen Z, Albrecht TB, Boor PJ, Pappas TC, Bell B, Motamedi M. 2006. Whole-body hyperthermia induces up-regulation of vascular endothelial growth factor accompanied by neovascularization in cardiac tissue. Life Sci. 79:1781–1788.
- Hapner CD, Deuster P, Chen Y. 2010. Inhibition of oxidative hemolysis by quercetin, but not other antioxidants. Chem Biol Interact. 186:275–279.
- Havenith G. 2001. Human surface to mass ratio and body core temperature in exercise heat stress—a concept revisited. J Thermal Biol. 26:387–393.
- Hotchkiss R, Nunnally I, Lindquist S, Taulien J, Perdrizet G, Karl I. 1993. Hyperthermia protects mice against the lethal effects of endotoxin. Am J Physiol. 265:R1447–R1457.
- Jones TJ, Li D, Wolf IM, Wadekar SA, Periyasamy S, Sanchez ER. 2004. Enhancement of glucocorticoid receptor-mediated gene expression by constitutively active heat shock factor 1. Mol Endocrinol. 18:509–520.
- Katschinski DM, Wiedemann GJ, Longo W, d'Oleire FR, Spriggs D, Robins HI. 1999. Whole body hyperthermia cytokine induction: A review, and unifying hypothesis for myeloprotection in the setting of cytotoxic therapy. Cytokine Growth Factor Rev. 10:93–97.
- Kim JI, Jung SW, Yang E, Park KM, Eto M, Kim IK. 2010. Heat shock augments angiotensin II-induced vascular contraction through increased production of reactive oxygen species. Biochem Biophys Res Commun. 399:452–457.
- Leon LR. 2002. Invited review: Cytokine regulation of fever: Studies using gene knockout mice. J Appl Physiol. 92:2648–2655.
- Leon LR, DuBose DA, Mason CW. 2005. Heat stress induces a biphasic thermoregulatory response in mice. Am J Physiol Regul Integr Comp Physiol. 288:R197–R204.
- Leon LR, Blaha MD, DuBose DA. 2006. Time course of cytokine, corticosterone, and tissue injury responses in mice during heat strain recovery. J Appl Physiol. 100:1400–1409.
- Li QL, Ju ZH, Huang JM, Li JB, Li RL, Hou MH, Wang CF, Zhong JF. 2011. Two novel SNPs in HSF1 gene are associated with thermal tolerance traits in Chinese Holstein cattle. DNA Cell Biol. 30:247–254.
- Lin MT, Chai CY. 1975. Effects of sodium acetylsalicylate on body temperature of monkeys under heat exposure. J Pharmacol Exp Ther. 194:165–170.
- Michel V, Peinnequin A, Alonso A, Buguet A, Cespuglio R, Canini F. 2007. Decreased heat tolerance is associated with hypothalamo–pituitary–adrenocortical axis impairment. Neuroscience. 147:522–531.
- Mizzen LA, Welch WJ. 1988. Characterization of the thermotolerant cell. I. Effects on protein synthesis activity and the regulation of heat-shock protein 70 expression. J Cell Biol. 106:1105–1116.
- Moran DS, Eli-Berchoer L, Heled Y, Mendel L, Schocina M, Horowitz M. 2006. Heat intolerance: Does gene transcription contribute?. J Appl Physiol. 100:1370–1376.
- Neuhofer W, Beck FX. 2005. Cell survival in the hostile environment of the renal medulla. Annu Rev Physiol. 67:531–555.
- Niu KC, Lin KC, Yang CY, Lin MT. 2003. Protective effects of alpha-tocopherol and mannitol in both circulatory shock and cerebral ischaemia injury in rat heatstroke. Clin Exp Pharmacol Physiol. 30:745–751.
- Ohara K, Furuyama F, Isobe Y. 1975. Prediction of survival time of rats in severe heat. J Appl Physiol. 38:724–729.
- Ritchie KP, Keller BM, Syed KM, Lepock JR. 1994. Hyperthermia (heat shock)-induced protein denaturation in liver, muscle and lens tissue as determined by differential scanning calorimetry. Int J Hyperthermia. 10:605–618.
- Ruell PA, Hoffman KM, Chow CM, Thompson MW. 2004. Effect of temperature and duration of hyperthermia on HSP72 induction in rat tissues. Mol Cell Biochem. 267:187–194.
- Silva CM, Powell-Oliver FE, Jewell CM, Sar M, Allgood VE, Cidlowski JA. 1994. Regulation of the human glucocorticoid receptor by long-term and chronic treatment with glucocorticoid. Steroids. 59:436–442.
- Szelenyi Z, Hummel Z, Szolcsanyi J, Davis JB. 2004. Daily body temperature rhythm and heat tolerance in TRPV1 knockout and capsaicin pretreated mice. Eur J Neurosci. 19:1421–1424.
- Trinklein ND, Murray JI, Hartman SJ, Botstein D, Myers RM. 2004. The role of heat shock transcription factor 1 in the genome-wide regulation of the mammalian heat shock response. Mol Biol Cell. 15:1254–1261.
- Tseng CS, Lo HW, Teng HC, Lo WC, Ker CG. 2005. Elevated levels of plasma VEGF in patients with dengue hemorrhagic fever. FEMS Immunol Med Microbiol. 43:99–102.
- Wadekar SA, Li D, Sanchez ER. 2004. Agonist-activated glucocorticoid receptor inhibits binding of heat shock factor 1 to the heat shock protein 70 promoter in vivo. Mol Endocrinol. 18:500–508.
- Wang NL, Chang CK, Liou YL, Lin CL, Lin MT. 2005. Shengmai San, a Chinese herbal medicine protects against rat heat stroke by reducing inflammatory cytokines and nitric oxide formation. J Pharmacol Sci. 98:1–7.
- Welch WJ, Baumgartl H, Lubbers D, Wilcox CS. 2001. Nephron pO2 and renal oxygen usage in the hypertensive rat kidney. Kidney Int. 59:230–237.
- Wilson TE, Crandall CG. 2011. Effect of thermal stress on cardiac function. Exerc Sport Sci Rev. 39:12–17.
- Wright GL. 1976. Critical thermal maximum in mice. J Appl Physiol. 40:683–687.
- Wright G, Knecht E, Wasserman D. 1977. Colonic heating patterns and the variation of thermal resistance among rats. J Appl Physiol. 43:59–64.
- Wu C. 1995. Heat shock transcription factors: Structure and regulation. Annu Rev Cell Dev Biol. 11:441–469.