Abstract
Context: MicroRNAs (miRNAs) are important and powerful mediators in a variety of diseases including cardiovascular pathology. Thus, they emerged as interesting new drug targets. However, it is important to develop efficient transfer tools to successfully deliver miRNAs or antisense oligonucleotides (antagomirs) to the target tissue.
Objective: The aim of this study was to review the scientific literature on delivery techniques currently used for transfer of miRNAs and antagomirs to animal models of cardiovascular disease and those that are likely to be used for therapeutic miRNA transport in the nearest future.
Methods: The research was carried out by consulting the following medical websites: Medicus Medline Index, PubMed (National Library of Medicine), and a registry database of clinical trials conducted in USA (www.clinicaltrials.gov). The selection gathers articles written in English, published from January 2012.
Results: A current delivery technique includes chemical modification of antagomirs with 2-O-methyl-group or 2-O-methyoxyethyl or using locked nucleic acids to increase drug stability and affinity. Development of miRNA sponges/decoys aims to target all members of a miRNA seed family of interest. A further strategy to augment miRNA levels is to use miRNA delivery through viral-based vectors including adenoviruses, adeno-associated viruses, and lentiviruses. To date, a variety of nanocarriers is available for efficient delivery of miRNAs. Microvesicles, and apoptotic bodies that contain circulating miRNAs could be also used as therapeutic transport systems in the nearest future.
Conclusion: Development of new miRNA carrier systems with advanced properties and large animal data in the cardiovascular field is highly recommended.
Introduction
Arterial atherosclerosis is a chronic disease associated with vascular wall thickening due to the deposition of fat materials such as cholesterol. Atherosclerosis involves progression of a wall lesion from a fatty streak to advanced atherosclerotic plaque accompanied with chronic inflammation, vascular remodeling, and plaque vulnerability. The vulnerable plaque is unstable since it can suddenly rupture and induce thrombus formation in the lumen. Finally, atherosclerosis can result in heart attack or thromboembolism including stroke. The high mortality and morbidity rate from cardiovascular diseases makes atherosclerosis management and treatment to be an issue of emerging significance. Thus, development of new efficient therapeutics with advanced curable properties is a task of great importance in cardiovascular medicine and pharmacy.
MicroRNAs (miRNAs) are endogenous, conserved approximately 22-nucleotide noncoding RNAs that anneal to inexactly complementary sequences in the 3′-untranslated regions (3′UTR) of target mRNAs of protein-coding genes to cause mRNA cleavage or repression of the translational machinery for protein synthesis (Lai, Citation2002). As regulators of gene expression, miRNAs play a pivotal role in all cellular mechanisms, organogenesis, and pathology including cardiovascular diseases. To date, the implication of sets of mRNAs in cardiovascular pathology is well characterized (for review, see Creemers et al., Citation2012; Sacco & Adeli, Citation2012; Salic & De Windt, Citation2012; Zampetaki & Mayr, Citation2012). The miRNA expression patterns were found to change in various cardiovascular diseases such as myocardial infarction, cardiac hypertrophy, and heart failure (Dorn, Citation2010; Mendrick, Citation2011). The examples of miRNAs involved in cardiovascular abnormalities are presented in . Circulating extracellular miRNAs normally presented in body fluids of normal subjects were also detected in cardiovascular diseased patients (Creemers et al., Citation2012). Loading of the miRNAs into proteins, lipids, or lipoprotein complexes such as exosomes or microvesicles provides essential stability to circulating miRNAs against cleavage with RNases presenting in the serum and other body fluids. Indeed, circulating miRNAs may be used as biomarkers but may also function as mediators or protectors against disease (Fichtlscherer et al., Citation2011). Recently, Hergenreider et al. (Citation2012) found atheroprotective communication between endothelial and smooth muscle cells through release of vesicles enriched with antiatherogenic miR-143/145. Since miRNAs target not only single mRNAs, but complete networks of often functionally related transcripts, they could represent interesting candidates for the development of new miRNA-based therapeutics in the treatment of atherosclerosis.
Table 1. Role of miRNAs in cardiovascular pathology.
Chemically modified miRNA analogs
The miRNAs whose expression is markedly increased in atheroslerotic vessels are likely to play an atherogenic role. Therefore, healing of affected vessels should benefit from suppressing these miRNAs. A strategy to inhibit atherogenic miRNAs is using of miRNA interference, i.e., development of antisense oligonucleotides termed antimirs or antagomirs to directly bind to a target miRNA and block it (Krützfeldt et al., Citation2005). An antagomir is a small synthetic RNA that is perfectly complementary to the specific miRNA target with either mispairing at the cleavage site of Ago2 or some sort of base modification to inhibit Argonaute2 cleavage. To increase stability and efficiency, an antisense oligonucleotide could be modified with 2-O-methyl-group (OMe) or 2-O-methyoxyethyl (MOE). Compared to the OMe antagomirs, MOE-analogs have a higher affinity and specificity to RNAs (Broderick & Zamore, Citation2011). A cholesterol construct is linked to an antagomir to improve cellular uptake (Thum et al., Citation2008).
Alternative miRNA chemistries that include oligonucleotide modification with 2′,4′-methylene are termed locked nucleic acids (LNAs). A LNA introduces a 2′,4′ methylene bridge in the ribose to form a bicyclic nucleotide. This modification greatly increases antagomir stability and affinity, and binding of a LNA-based antagomir to a target miRNA results in a thermodynamically very strong duplex formation (Grünweller & Hartmann, Citation2007) ().
Figure 1. Chemical modifications that improve the stability, biodistribution and delivery of antisense oligonucleotides to target miRNAs. 2′-O-methyl RNA contains a methyl group bound to the 2′ oxygen of the ribose; 2′-O-methoxyethyl RNA contains a methoxy group bound to the 2′ oxygen of the ribose; and locked nucleic acid introduces a 2′,4′ methylene bridge in the ribose to form a bicyclic nucleotide.
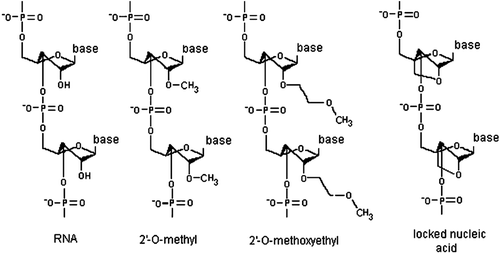
Preclinical and clinical applications of miRNA analogs
Chemically modified antagomirs were widely tested to normalize lipid profiles and improve vascular and heart function in animal models, mostly in mice with artificially induced left ventricular pressure overload or with limb ischemia and myocardial infarction (). Patrick et al. (Citation2010) and Thum et al. (Citation2011) compared efficiency of antagomirs against cardiac miR-21 differed in length and modified with different chemistries. They reported successful repression of cardiac miR-21 by a short (8-mer) anti–miR-21 in mouse model of left ventricular pressure. However, using a long (22-mer) anti–miR-21 resulted in a more profound repression of miR-21 (by 80%). In addition, 8-mer LNA-inhibitors of miR-21 were unable to impove pressure overload-induced cardiac hypertrophy, fibrosis, and cardiac dysfunction (Patrick et al., Citation2010). In contrast, both cholesterol- and MOE-modified 22-mer oligonucleotides were capable to strongly attenuate cardiac disease and prevent decline in cardiac function (Thum et al., Citation2011). Indeed, for long-term inhibition of miR-21 function in vivo, interventions based on longer anti-miRs are likely to prove superior, due to their high potency and treatment duration.
Most miRNAs whose expression was experimentally inhibited by antagomirs in animal models of cardiovascular disease () have atherogenic effect except for miR-29 and miR-133. Suppression of miR-133 in mice by anti-miR-133 resulted in cardiac hypertrophy (Care et al., Citation2007). miR-133 was found to suppress several targets including RhoA (a GDP-GTP exchange protein), Cdc42 (signal transduction kinase), Nelf-A/WHSC2 (nuclear transcription factor), and calcineurin (calcium-dependent serine-threonine phosphatase), all involved in the control of heart hypertrophy and cardiogenesis. Interestingly, calcineurin/NFAT (nuclear factor of activated T cells) signaling was observed to reciprocally regulate expression of miR-133 suggesting for a novel mechanism for progressive cardiac hypertrophy (Dong et al., Citation2010).
Table 2. Examples of using chemically modified antigomirs in in vivo models of heart and vascular injury.
MiR-29 prevents cardiac fibrosis. Expression of this microRNA is down-regulated in the region of the heart adjacent to the infarct in humans and mice affected with myocardial infarction (MI) (van Rooij et al. Citation2008). miR-29 targets multiple genes whose products are implicated in the extracellular matrix production in the heart including collagens (COL1A1, COL1A2, and COL3A1), fibrillin, elastin, and connective tissue growth factor (van Rooij et al., Citation2008; Duisters et al., Citation2009). Due to the central role in the regulation of fibrosis, miR-29 represents an interesting therapeutic target for tissue fibrosis in general.
Overall, preclinical testing of series of chemically modified antagomirs showed promising results. For example, a daily intravenous injection of a 16-nucleotide LNA-modified oligonucleotide complementary to miRNA-122 for 5 successive days at 10 mg/kg resulted in lowered plasma cholesterol levels for more than 20 days in African green monkeys (Elmén et al., Citation2008). This study showed obviously that intravenous administration of LNA-modified anti-miRNA oligonucleotides allows reaching specific, stable, and prolongated therapeutic effects of miRNA analogs. A high efficiency of a 15-nucleotide miR-122-specific LNA inhibitor to inhibit proliferation of hepatitis C virus (HCV) in chimpanzee was then demonstrated (Lanford et al., Citation2010). The LNA inhibitor was injected intravenously each week for 12 weeks at doses of 5 mg/kg to two animals infected with HCV. Free anti-miR-122 LNA was detected in liver for 8 weeks after treatment ended. The treatment with the LNA inhibitors resulted in 200- and 400-fold stable decrease of the virus titer in the liver and serum, respectively, and significant histological hepatic improvements. Promising results achieved in preclinical testing of anti-miR-122 LNA inhibitors in primates provided an essential background to start clinical trials.
There are first examples of clinical testing of LNA suppressors of miRNAs including LNA-based inhibitor of miR-122. This inhibitor termed miravirsen (SPC3649) is currently under investigation in a phase II clinical trial in patients with hepatitis C conducted by Santaris Pharma (San Diego, CA, USA) (http://santarispharma.com/product-pipeline).
Two other US pharmaceutical companies, Miragen Therapeutics (Boulder, CO, USA) and Regulus Therapeutics (San Diego, CA, USA), carried out a large-scale preclinical studying of modified miRNA analogs and antagomirs for targeted therapy of cardiovascular and metabolic diseases. At present, Miragen Therapeutics develops three research applications (programs MGN-6114, MGN-5804, and MGN-2677) to target miR-92 in peripheral arterial disease, miR-378 in cardiometabolic disease, and miR-143/145 in vascular disease, respectively (http://www.miragentherapeutics.com/7/Pipeline/). The MGN-9103 program to target miR208/499 in chronic heart failure and MGN-1374 (targeting miR-15/195 involved in cardiac fibrosis) performed by this company reached the preclinical testing stage. Regulus Therapeutics develops tools to target proatherogenic miR-33 (http://www.regulusrx.com/therapeutic-areas/#undefined). In mouse model of atherosclerosis, treatment with anti-miR-33 developed in Regulus Therapeutics led to reduction in arterial plaque size by 35%, whereas treatment in non-human primates increased circulating levels of high-density lipoprotein-cholesterol (HDL-C) by 50% (Rayner et al., Citation2011a, Citation2011b). Hopefully, clinical testing of chemically modified oligonucleotide inhibitors of atherogenic miRNAs is not far to start soon.
miRNA sponges/decoys
Another innovative approach termed miRNA ‘sponges’ or ‘decoys’ was invented by Ebert et al. (Citation2007). The idea behind it is to produce a single piece of RNAs containing multiple, tandem-binding sites for a miRNA seed family of interest, in order to target all members of that miRNA seed family taking advantage of the fact that the interaction between miRNA and target is nucleated by and largely dependent on base pairing in the seed region (positions 2–8 of the miRNA). The authors constructed sponges by inserting tandemly arrayed miRNA binding sites into the 3′UTR of a reporter gene encoding destabilized green fluorescent protein (GFP) driven by the cytomegalovirus promoter, which can yield abundant expression of the competitive inhibitor transcripts. Binding sites for a particular miRNA seed family were perfectly complementary in the seed region with a bulge at positions 9–12 to prevent RNA-interference-type cleavage and degradation of the sponge RNA.
When vectors encoding miRNA sponges are transiently transfected into cultured cells, they depress miRNA targets as strongly as the conventional antagomirs. The major advancement of this technique over the antagomir technique is that it can better inhibit functional classes of miRNAs than do antagomirs that are designed to block single miRNA sequences. miRNA sponge adenoviral and lentiviral constructs were used to investigate a pathogenic role of miR-133 and miR-21 in cardiac hypertrophy (Carè et al., Citation2007; Sayed et al., Citation2008; Horie et al., Citation2009).
Further generation of miRNA decoy constructs termed ‘miRZips’ and ‘TuD RNAs’ (tough decoy RNAs) are miRNAs decoy targets transcribed by RNA polymerase III (H1 or U6) (Haraguchi et al., Citation2009). Their nuclear export has been optimized to achieve high cytoplasmic expression. miRZips use the RNA polymerase III H1 promoter to express a single miRNA decoy hairpin with one arm that is perfectly complementary to the miRNA. This strategy causes degradation of the miRNA. TuD RNAs use the RNA polymerase III U6 promoter to express an RNA that contains multiple miRNA-binding sites between 18 base pair stem regions that help to prevent nuclease degradation of the RNA decoy targets. For the TuD RNAs, the binding site is perfectly complementary to the miRNA but contains four nucleotides inserted at the site of Ago2 cleavage to prevent the TuD RNA from being inactivated. Such adaptations of the miRNA sponge concept allow longer-term inhibition of miRNAs than can be achieved by transient transfection of RNA polymerase II sponge reporter vectors or modified RNA oligonucleotides. Xie et al. (Citation2012) combined recombinant adeno-associated virus (rAAV) vectors with miRNA TuDs to inhibit specific miRNAs. Injection of a rAAV9 construct expressing anti-miR-122 or anti-let-7 TuDs resulted in long-term depletion of the corresponding miRNA and increased expression of its mRNA targets. rAAV producing anti-miR-122 TuD but not anti-let-7 TuD reduced serum cholesterol by >30% for 25 weeks in wild-type mice. Indeed, Xie et al. (Citation2012) developed a construct of a potential therapeutic interest for treatment of dyslipidemia, a disease that significantly increases risk of atherosclerosis.
Recently, Haraguchi et al. (Citation2012) reported the development of new synthetic miRNA inhibitors designated as S-TuD (Synthetic TuD), which are composed of two fully 2′-O-methylated RNA strands. Each of these strands includes a miRNA-binding site that should increase the inhibitory capacity of an S-TuD miRNA as compared to the TuD miRNA inhibitor containing only a single miRNA-binding site. When tested in different cell lines, S-TuDs shows long-lasting inhibitory effects at very low dosages and had no detectable interferon-inducing activity in culture. These characteristics of S-TuD are advantageous for its potential as a future therapeutic drug.
Viral-based delivery
A further strategy to augment miRNA levels is to use miRNA delivery through adenoviral or lentiviral-based vectors. Certain adenoviral serotypes have the unique feature of cell-type favoring tropism without leading to significant inflammatory responses (Yao et al., Citation2011). Viral vectors used for viral-based therapy should also share several key properties such as safety, low toxicity, stability, and possibility to be identified within the target cell in order to measure the efficiency of delivery. The biosafety of viral-based genetic constructs could be commonly achieved by deleting a part of a virus genome responsible for viral pathogenicity. Such a virus is capable to infect a cell but requires a helper virus to provide the missing proteins for production of new virions (Vetrini & Ng, Citation2010). To increase targeting specificity, a new generation of adenoviral vectors is modified with a bifunctional polyethylene glycol (PEG). Modification of the surface of adenovirus with heterofunctional PEG ablates the normal tropism of the virus and reduces transduction of non-target tissues in vivo. Furthermore, the addition of PEG chains to the surface of the virus shields antigen-binding sites significantly reducing the susceptibility of the virus to antibody neutralization in patients positive for adenoviral antibodies (O’Riordan & Song, Citation2008).
Adenoviral vectors were successfully used in to deliver miRNA or anti-miR constructs into murine models of cardiovascular disease. Knockdown of miR-98 and other members of the let-7 miRNA family with anti-miR constructs augmented cardiac hypertrophy. In contrast, adenovirus-mediated overexpression of miR-98 in cardiomyocytes reduced cell size both at baseline and in response to angiotensin II suggesting for a protective role of this microRNA against angiotensin II-induced cardiac hypertrophy (Yang et al., 2011b). Caporali et al. (Citation2011) used an adenoviral vector to transfer a miR-503 decoy to the ischemic adductor of diabetic mice. The delivery of a miR-503 decoy corrected diabetes mellitus-induced impairment of post-ischemic angiogenesis and blood flow recovery. As opposed to this, lentiviral-mediated expression of miR-503 in endothelial cells inhibited cell proliferation and migration. Expression of this microRNA was found to be upregulated in vascular endothelial cells growing in culture conditions mimicking diabetes mellitus (high glucose) and ischemia-associated starvation (low growth factors) (Caporali et al., Citation2011). These observations make miR-503 as a possible therapeutic target in diabetic patients with critical limb ischemia.
By adenovirus-mediated delivering of miR-145 into balloon-injured rat carotid arteries in vivo, Cheng et al. (Citation2009) showed a key role of miR-145 against pathogenic neointima formation, which occurs in atherosclerosis, restenosis, hypertension, and other vascular proliferative diseases, through inhibiting proliferation of vascular smooth muscle cells (VSMCs). Forced expression of miR-328 through adenovirus infection in canine atrium and transgenic approach in mice recapitulated the phenotypes of atrial fibrillation such as exemplified by enhanced atrial fibrillation vulnerability, diminished L-type Ca2+ current, and shortened atrial action potential duration. These findings suggest that miR-328 could contribute to the adverse atrial electric remodeling in atrial fibrillation by targeting cardiac L-type Ca2+ channels CACNA1C and CACNB1 (Lu et al., Citation2010).
Compared to adenoviruses whose DNA does not integrate into the genome and is not replicated during cell division, adeno-associated viruses (AAVs) can infect both dividing and nondividing cells and may incorporate its genome into that of the host cell. These features make AAV a very attractive candidate for creating viral vectors for gene therapy (Grieger & Samulski, Citation2012). Since recombinant AAVs are able to target several tissues, it is crucial to improve AAVs tissue specificity to target, for example, a cardiac muscle (Xie et al., Citation2011). This could be achieved by incorporation into a recombinant AAV of target sequences for tissue-specific miRNAs. For example, AAV vectors with capsids of AAV serotype 9 enable an efficient transduction of the heart upon intravenous injection of adult mice but also transduce the liver. To improve a cardiospecificity of AAV9 vectors, Geisler et al. (Citation2011) constructed an AAV9-based vector containing target sites of liver-specific miR-122, miR-192, and miR-148a in the 3′UTR of a reporter luciferase gene. Luciferase expression was then efficiently suppressed in liver cell lines expressing high levels of the corresponding miRs, whereas luciferase expression was unaffected in cardiac myocytes. AAV9 vectors bearing three repeats of the miR-122 target sequence in the 3′UTR of an enhanced GFP (EGFP) expression cassette were then intravenously injected into mice. The injection resulted in the absence of EGFP expression in the liver of adult mice. The liver-specific detargeting in vivo using miR-122 was even more efficient than transcriptional targeting with a cardiac cytomegalovirus-enhanced myosin light chain promoter (Geisler et al., Citation2011). These data indicate that miR-regulated targeting is a powerful new tool to further improve cardiospecificity of AAV9 vectors.
Lentiviruses have recently been adapted as gene delivery vectors thanks to their ability to integrate into the genome of non-dividing cells, which is the unique feature of lentiviruses. Lentiviral-mediated transfer of several miRNAs was performed to animal models with cardiovascular defects. By lentiviral-mediated transduction of rat VSMCs with miR-143 and miR-145, Cordes et al. (Citation2009) reported a crucial role of both miRNAs in preventing neointima formation caused by proliferation and migration of VSMCs. Intravenous injection of a lentiviral anti-miR-33 construct caused increase in plasma HDL and reduction of very light density lipoprotein (VLDL) triglycerides in non-human primates suggesting for the proatherogenic effect of this microRNA (Rayner et al., Citation2011a). MiR-33 suppresses expression of the cholesterol transporter ABC transporter A1 (ABCA1) in plaque macrophages and lowers HDL levels (Rayner et al., Citation2011b). Inhibition of miR-33 results in raising HDL levels and activation of ABCA1 that promotes reverse cholesterol transport and atherosclerosis regression. Thus, miR-33 represents a promising target in the treatment of atherosclerotic vascular disease.
Lentiviral vectors were widely used for delivery of small regulatory RNAs such as short-hairpin RNAs (shRNAs) to silence cardiac-specific expression of various genes upregulated in animal models of heart hypertrophy (Cingolani et al., Citation2007; Gupta et al., Citation2008; Gupta et al., Citation2009). A similar strategy could be used for a cardiac-specific transfer of miRNAs capable to suppress those genes. These studies can be a precursor to the development of novel drugs which aim to block a gene-product to treat a disease. The first clinical trial using a lentiviral vector was conducted in 2005 (Manilla et al., Citation2005). The use of lentiviral vectors has been increasing because the vector system has several attractive features including ability to infect neighboring cells in direct contact with the host cells, without having to form extracellular particles. To date, there are 15 active clinical trials using lentiviral-mediated gene delivery (http://clinicaltrials.gov/ct2/results?term=lentiviral+vector). Most of them focused on the treatment of genetic syndromes. There are no clinical trials evaluating lentiviral vectors for gene therapy of cardiovascular disease. However, due to the great therapeutic potential, we can expect using lentivirus-mediated miRNA delivery for treatment of cardiovascular pathology within the next decade.
Nanocarriers
Nanotechnology can be applied to components of plaque biology to enhance clinical approaches to atherosclerosis. The term nanotechnology, which deals with the study of material properties at the sub–100-nm scale, was originally associated with the miniaturization of semiconductor materials but has now been adapted to provide novel solutions to the clinical management of disease, giving rise to the term nanomedicine (Farokhzad & Langer, Citation2006).
Nanoscale devices, or ‘nanoparticles’, may be used for highly effective delivery of miRNAs in cardiovascular therapy. Prominent examples of nanocarrier matrices include liposomes, micelles, and block co-polymer nanoparticles (Wickline et al., Citation2006). When designing nanocarriers for therapeutics, drugs can be encapsulated within vehicles based on similar building blocks. Polylactide co-glycolide (PLGA) nanoparticles feature controlled degradation rates for tunable drug release profiles (Danhier et al., Citation2012). For gene therapy applications, the cationic polymer polyethyleneimine (PEI) has been extensively utilized, primarily in the branched form (Günther et al., Citation2011). Polyethylenimines (PEIs) are synthetic polymers, which are able to form non-covalent complexes with small RNAs such as shRNAs or miRNAs. These nanoscale complexes (‘nanoplexes’) allow the protection of small RNAs from nucleolytic degradation, their efficient cellular uptake through endocytosis and intracellular release through the ‘proton sponge effect’.
To prolong persistence of the therapeutic agent in vivo, polyethylene glycol-coated phospholipid liposomes have been designed that delay clearance from the circulation (Marik et al., Citation2007). Therapeutic nanocarriers are usually functionalized with biorecognition moieties on the surface such as peptides or antibodies to enable in vivo delivery of the therapy to the intended site such as the macrophage (Günther et al., Citation2011). Compared to PLGA nanoparticles and liposomes, PEI nanocarriers were the nanoparticles the most frequently used for miRNA delivery (Ibrahim et al., Citation2011). However, the disadvantages of PEI- or cationic liposome-based gene carriers are the cytotoxicity induced by excess positive charge as well as the aggregation on the cell surface. To overcome those detriments, biodegradable PLGA nanospheres may be very helpful particularly for efficient delivery of cytosolic therapeutics such as miRNAs (Vasir and Labhasetwar, Citation2007; Liang et al., Citation2011).
Interfering nanoparticles (iNOPs) prepared from lipid-functionalized poly-l-lysine dendrimer represent a new delivery agent for efficient transfer of small RNAs including short interfering RNAs, miRNA mimics, and antimirs. Systemic delivery of a chemically stabilized anti-miR-122 by iNOPs into a mouse liver resulted in a long-term inhibition of miR-122 by 83% and did not induce an immune response (Baigude & Rana, Citation2012).
There are only a few reports for using nanoparticle-mediated delivery of miRNAs in cardiovascular therapy. Cetyltriethylammonium bromide (CTAB)-modified positively-charged poly(ethylene oxide)-poly(epsilon-caprolactone) (PEO-PCL) nanoparticles were used to deliver a plasmid encoding a cardioprotective miR-210 into mesenchymal stem cells. These cells showed a higher survival under lethal anoxia as compared with non-treated cells and then supported a better recovery of ischemic rat heart post-transplantation (Kim et al., in press). However, due to the availability of a variety of nanocarriers capable to effectively transfer miRNAs, it is not surprising if nanoparticle-mediated delivery of miRNAs and antimirs will be extensively explored in animal models of cardiovascular injury in the nearest future.
Exosomes/microvesicles and apoptotic bodies
As mentioned above, miRNAs are able to be transferred in blood by vesicles or exosomes. Circulating miRNAs play a regulatory role, and their serum profiles may be significantly changed in vascular disease (Vickers et al., Citation2011). Cocucci et al. (Citation2008) showed that serum miRNAs were packaged into double-layered microvesicles, which are often shed from almost all cell types under normal and pathologic conditions and interact selectively with target cells through receptors from the original cells. Zhang et al. (Citation2010) observed an active packaging of mainly miR-150 into microvesicles and further secretion of microvesicles in human blood cells and cultured human macrophage cell line THP-1.
In culture, microvesicles secreted by THP-1 were able to transfer miR-150 to human microvessel endothelial cells HMEC-1 and activate their migration. miR-150 is involved in the regulation of innate immunity by targeting mRNA for c-Myb and CXCR4 that control cell lineage commitment, proliferation, differentiation, and migration (Xiao et al., Citation2007a; Tano et al., Citation2011). In the human infarcted heart, cardiac levels of miR-150 were shown to be down-regulated indicating the activation of innate immunity and development of an acute inflammatory reaction as a consequence (Bostjancic et al., Citation2009; Zidar et al., Citation2011). In contrast, plasma levels of miR-150 were elevated in atherosclerosis patients. In cultivated THP-1 cells, secretion of miR-150 could be enhanced by endotoxin, increased lipid levels and oxidative stress (Zhang et al., Citation2010). Indeed, in acute cardiovascular stress, circulating levels of miR-150 are increased causing stimulation of migration of HMECs as a protective response against vascular injury.
Furthermore, Zhang et al. (Citation2010) showed a direct in vivo delivery of miR-150 to the endothelium under physiological conditions by intravenous injection of THP-1-derived microvesicles to laboratory mice. These findings showed that microvesicles could be potentially used for delivery of therapeutics such as miRNAs to the affected endothelium.
Except for miR-150, it is interesting to consider a clinical potential of another circulating mRNA, miR-126. This miRNA plays a proangiogenic role supporting proliferation, migration, tubule formation, and sprouting of endothelial cells (ECs) (Fish et al., Citation2008). miR-126 knockdown in mice with experimentally induced myocardial infarction significantly reduces angiogenesis and increases mortality (Wang et al., Citation2008). Zernecke et al. (Citation2009) isolated apoptotic bodies from ECs, which are typically engulfed by phagocytes, contained mainly miR-126. Incubation of these apoptotic bodies with human umbilical vein ECs (HUVECs) resulted in transfer of miR-126 into recipient cells and production of the anti-inflammatory chemokine CXCL12 by HUVECs. miR-126 targets CXCL12 and RGS16, a negative regulator of expression of CXCL12-binding receptor CXCR4 (Zernecke et al., Citation2009). miR-126-mediated CXCL12 abundance in ECs enhanced CXCR4-mediated recruitment of progenitor cells, which contributes to vascular wall repair after injury (Zernecke et al., Citation2005).
The angioprotective role of miR-126 and the efficiency of apoptotic body miRNA transfer were demonstrated in the apolipoprotein-deficient (Apoe−/−) mouse model of atherosclerosis. Intravenous administration of apoptotic bodies derived from HUVECs and blood of atherosclerosis patients to Apoe−/− mice fed a high-fat diet resulted in increased numbers of endothelial progenitor cells, reduced size and number of macrophages, and enhanced apoptosis in atherosclerotic lesions (Bot et al., Citation2007; Leroyer et al., Citation2007). All these changes were mediated by miR-126 in a CXCR4-dependent manner (Zernecke et al., Citation2008). These experiments clearly indicate antiatherogenic effects of miR-126, its promising clinical significance for treatment of atherosclerosis, and a therapeutic potential of apoptotic bodies for miRNA delivery. A protective role of this miRNA against coronary artery disease and type 2 diabetes could also be supported by reduced levels of miR-126 in blood of affected patients (Fichtlscherer et al., Citation2010; Zampetaki et al., Citation2010). Human progenitor ECs were shown to also release microvesicles containing miR-126, miR-296 and other angiogenic miRNAs (Deregibus et al., Citation2007). Microvesicles derived from these progenitor cells were capable to promote endothelial cell survival, proliferation, and organization in capillary-like structures in immunodeficient mice (Deregibus et al., Citation2007) and to induce neoangiogenesis and enhance recovery in a murine model of hindlimb ischemia (Ranghino et al., Citation2012). These observations suggest for a putative therapeutic potential of progenitor ECs as a source of microvesicles.
To date, the possibility to use microvesicles and apoptotic bodies as therapeutic transport systems is far from reality since it is necessary to establish their production protocols and continue studying their pharmaceutical properties, stability, immunogenicity, toxicity, and biocompatibility/biodegradability. Recently, Akao et al. (Citation2011) showed that chemically modified miR-143 entrapped by microvesicles was significantly secreted from miR-143-transfected THP-1 cells during incubation in serum-free medium. Importantly, nude mice intravenously injected with these miR-143-contained microvesicles revealed a significant increase in the levels of miR-143 in the serum and kidney. Therefore, it seems to be possible to use THP-1 cells for production of microvesicles containing any therapeutically significant miRNA.
New developments in delivery formulations
Specific delivery of therapeutic RNA can met many obstacles such as cell membrane and endosomic vesicles. Systemic delivery of RNA therapeutics through blood flow is challenged by macrophages and monocytes that can phagocytize RNA-loaded nanoparticles. The size of RNA-containing therapeutic vehicles is also important since particles larger than 5 nm diameter cannot penetrate the capillary endothelium and remain to circulate in the blood until removed by the kidney.
To escape being filtered by the kidney and improve intracellular delivery, nucleic acids can be encapsulated in lipid-forming vesicles ranging between 50 and 500 nm in size. The anionic charge of the nucleic acids and their hydrophilicity is counterbalanced by the cationic lipids resulting in a net positive charge and enabling the liposomes to bind to anionic cell surface molecules. The lipid head group, for example, can be pH-sensitive, so that the liposomes interact with anionic phospholipids in the endosome generating non-bilayer structures that disrupt the endosomal membrane liberating the RNA.
By screening a library of ionizable cationic lipids with the best siRNA delivery capacity, Semple et al. (Citation2010) developed a new lipid nanoparticle formulation to decrease an effective dose of oligonucleotides. To test efficiency of this delivery formulation, the investigators used small interference RNA (siRNA) to target mRNA for transthyretin, a hepatic protein with a short half-time secreted to the serum. When administered intravenously as a single dose of 0.01 mg/kg (rodents) and 0.1 mg/kg (nonhuman primates), the siRNA was able to reduce hepatic transthyretin expression by 30% 48 hours after infusion.
Another efficient delivery formulation contains lipid nanoparticles with lipids having an amine-containing polar head group and non-polar alkyl tails. When tested in nonhuman primates, this lipidoid formulation was effective at 0.03 mg/kg of siRNA by decreasing hepatic transthyretin mRNA levels by 70% (Love et al., Citation2010). The advanced
The advanced delivery formulations presented here are 30–100 times more efficient than typical lipid-based delivery carriers, which require doses of at least 1 mg/kg of siRNA to achieve 50% gene silencing.
Toxicity concerns
Before introduction to clinical practice, miRNAs, their synthetic mimetics, and nanocarrier vehicles should be carefully assessed for potential toxic effects, biocompatibility, and biodegradability. The pathogenic pattern-sensing components of innate immunity such as some Toll-like receptors (TLRs) and RIG-1-like receptor helicases that recognize viral and bacterial nucleic acids (Barber, Citation2011; Loo & Gale, Citation2011) may be activated by modified synthetic oligonucleotides and in turn induce the inflammatory response and complement cascade. For example, systemic intratracheal administration or overdoses of synthetic oligodeoxynucleotides with unmethylated CpG dinucleotides were shown to cause acute lung injury and inflammation in laboratory animals (Tasaka et al., Citation2009) through the TLR-9-dependent mechanism (Knuefermann et al., Citation2007). Indeed, it is necessary to carefully titrate the dosage of oligonucleotide in order to prevent adverse tissue reactions due to the activation of immune response and unpleasable overstimulation of target genes.
Special biosafety concerns should be addressed to nanoparticles as therapeutics. For the calculation of the biological or chemical reactivity of the material, knowledge about the physico-chemical properties, the number of molecules on the surface of the nanosized material is needed, as well as the number of particles per cell. The number per cell is important to determine the effective dose, since nanoparticles have larger surface area than the corresponding bulk material including a higher number of molecules on the surface, which can interact with the biological material, and their larger number per mass allows their dispersion into more cells. Information about the physico-chemical properties including size and shape are also important in order to estimate specific effective dose of engineered nanaparticle as well (Teeguarden et al., Citation2007).
Another important dose measure might be the relative biological effectiveness (RBE). If the physico-chemical properties and the number of molecules on the surface of the nanosized material are known, as well as the number of particles per cell, weighting factors might be introduced to calculate RBE (CitationSCENIHR, 2007). For dose calculations relevant for both chronic and for acute exposure, the dose rate has to be known, which includes the time factor. To determine the retention time (how long a nanoparticle is present in a cell or a body) of a certain nanoparticle, knowledge about the physico-chemical properties, but also about the biological deposition time in each site (deposition and retention time are depending on deposition site) is needed (Gwinn & Vallyathan, Citation2006). In other words, knowledge about site-dependent retention time and bioavailability is needed to calculate the time factor for dose rate. Other factors that will affect the dose rate are that certain nanoparticles are biodegradable with a relatively short half-life whereas others will not be metabolized within the body. In addition, some engineered nanoparticles may be excreted, whereas others may accumulate over time.
Special attention should be paid to the assessment of biocompatibility and safety of materials from which nanoparticles are engineered. For example, poly-l-lactic acid, a popular polymer in bionanoengineering, is known to induce inflammatory response (Kim et al., Citation2011). Polydopamine coating of poly-l-lactic acid surfaces greatly reduces adverse biological responses and functionalizes surface forming a biocompatible layer to which proteins, peptides, oligonucleotides, metal ions or synthetic polymers are able to be attached (Hong et al., Citation2011). Another strategy to reduce toxicity of poly-l-lactic acid nanoparticles and increase their biocompatibility is using poly (d,l-lactide-co-glycolide acid) copolymers or chitozan as biodegradable nanoparticle drug carriers (Singha et al., Citation2011). Thus, multiple approaches are developed in modern bionanotechnology to make therapeutic nanoparticles more efficient and safe.
Conclusion
Many new roles for individual miRNAs in cardiovascular disease are likely to emerge over the next 5 years. As a particular disease condition may be associated with multiple miRNAs, and a given gene may be regulated by multiple miRNAs. Thus, interactions between miRNAs may have impact on the protein expression of the target genes and may complicate the potential role of miRNA as therapeutic targets in cardiovascular disease. We should further validate the specificity of the established miRNA targets before application.
Once the role of a specific miRNA in disease pathogenesis is established, selecting specific anti-miRNA inhibitor chemistries and delivery strategies promises to be straightforward. Thus, treating vascular diseases with anti-miRNA oligonucleotides will require the development of novel modification, conjugation or formulation strategies. It is our hope that the antimiRNA therapeutics field will soon converge on a small number of ‘platform’ technologies that allow a rapid and safe development path from academic discovery to effective drug. Soon, we will see first detailed phase I and II data using miRNA therapeutics in noncardiovascular but also cardiovascular medicine.
Development of new viral and non-viral technologies to transfer miRNAs and antimirs and their testing in animal models of cardiovascular injury should be further continued. Among non-viral delivery tools, generating new nanoparticles with advanced properties and their implementation in cardiovascular medicine is of great promise.
Large animal data also in the cardiovascular field will probably appear as this is worldwide currently under investigation in several laboratories. This will not only result in more insight into their therapeutic potential in larger species but also lead to important information about pharmacokinetics of such drugs and safety data.
New promising fields of miRNA applications should appear soon in the cardiovascular research. One of these fields may concern using miRNAs to improve growth, differentiation, and regenerative properties of progenitor and stem cells before transplantation to the infarcted heart. This new approach has been recently launched, and mesenchymal stem cells or cardiac progenitor cells genetically modified with miRNAs such as miR-1, miR-21, miR-24, miR-126, miR-155, and miR-221 showed advanced prosurvival properties and regenerative capacity in animal models of cardiovascular disease (Chen & Zhou, Citation2011; Glass & Singla, Citation2011; Hu et al., Citation2011; Liu et al., Citation2011).
Acknowledgement
This study is supported by the Russian Ministry of Education and Science.
Declaration of interest
The authors report no declarations of interest.
References
- Akao Y, Iio A, Itoh T, Noguchi S, Itoh Y, Ohtsuki Y et al. Microvesicle-mediated RNA molecule delivery system using monocytes/macrophages. Mol Ther 2011;19:395–399.
- Baigude H, Rana TM. Interfering nanoparticles for silencing microRNAs. Meth Enzymol 2012;509:339–353.
- Barber GN. Cytoplasmic DNA innate immune pathways. Immunol Rev 2011;243:99–108.
- Bonauer A, Carmona G, Iwasaki M, Mione M, Koyanagi M, Fischer A et al. MicroRNA-92a controls angiogenesis and functional recovery of ischemic tissues in mice. Science 2009;324:1710–1713.
- Boon RA, Seeger T, Heydt S, Fischer A, Hergenreider E, Horrevoets AJ et al. MicroRNA-29 in aortic dilation: implications for aneurysm formation. Circ Res 2011;109:1115–1119.
- Bostjancic E, Zidar N, Glavac D. MicroRNA microarray expression profiling in human myocardial infarction. Dis Markers 2009;27:255–268.
- Bot I, de Jager SC, Zernecke A, Lindstedt KA, van Berkel TJ, Weber C et al. Perivascular mast cells promote atherogenesis and induce plaque destabilization in apolipoprotein E-deficient mice. Circulation 2007;115:2516–2525.
- Broderick JA, Zamore PD. MicroRNA therapeutics. Gene Ther 2011;18:1104–1110.
- Callis TE, Pandya K, Seok HY, Tang RH, Tatsuguchi M, Huang ZP et al. MicroRNA-208a is a regulator of cardiac hypertrophy and conduction in mice. J Clin Invest 2009;119:2772–2786.
- Carè A, Catalucci D, Felicetti F, Bonci D, Addario A, Gallo P et al. MicroRNA-133 controls cardiac hypertrophy. Nat Med 2007;13:613–618.
- Caporali A, Meloni M, Völlenkle C, Bonci D, Sala-Newby GB, Addis R et al. Deregulation of microRNA-503 contributes to diabetes mellitus-induced impairment of endothelial function and reparative angiogenesis after limb ischemia. Circulation 2011;123:282–291.
- Chan LS, Yue PY, Mak NK, Wong RN. Role of microRNA-214 in ginsenoside-Rg1-induced angiogenesis. Eur J Pharm Sci 2009;38:370–377.
- Chen T, Huang Z, Wang L, Wang Y, Wu F, Meng S et al. MicroRNA-125a-5p partly regulates the inflammatory response, lipid uptake, and ORP9 expression in oxLDL-stimulated monocyte/macrophages. Cardiovasc Res 2009;83:131–139.
- Chen JJ, Zhou SH. Mesenchymal stem cells overexpressing MiR-126 enhance ischemic angiogenesis via the AKT/ERK-related pathway. Cardiol J 2011;18:675–681.
- Chen J, Yin H, Jiang Y, Radhakrishnan SK, Huang ZP, Li J et al. Induction of microRNA-1 by myocardin in smooth muscle cells inhibits cell proliferation. Arterioscler Thromb Vasc Biol 2011a;31:368–375.
- Chen T, Li Z, Tu J, Zhu W, Ge J, Zheng X et al. MicroRNA-29a regulates pro-inflammatory cytokine secretion and scavenger receptor expression by targeting LPL in oxLDL-stimulated dendritic cells. FEBS Lett 2011b;585:657–663.
- Chen T, Li Z, Jing T, Zhu W, Ge J, Zheng X et al. MicroRNA-146a regulates the maturation process and pro-inflammatory cytokine secretion by targeting CD40L in oxLDL-stimulated dendritic cells. FEBS Lett 2011c;585:567–573.
- Chen H, Untiveros GM, McKee LA, Perez J, Li J, Antin PB et al. Micro-RNA-195 and -451 regulate the LKB1/AMPK signaling axis by targeting MO25. PLoS ONE 2012;7:e41574.
- Cheng Y, Liu X, Yang J, Lin Y, Xu DZ, Lu Q et al. MicroRNA-145, a novel smooth muscle cell phenotypic marker and modulator, controls vascular neointimal lesion formation. Circ Res 2009;105:158–166.
- Cingolani E, Ramirez Correa GA, Kizana E, Murata M, Cho HC, Marbán E. Gene therapy to inhibit the calcium channel β subunit: physiological consequences and pathophysiological effects in models of cardiac hypertrophy. Circ Res 2007;101:166–175.
- Cocucci E, Racchetti G, Rupnik M, Meldolesi J. The regulated exocytosis of enlargeosomes is mediated by a SNARE machinery that includes VAMP4. J Cell Sci 2008;121:2983–2991.
- Cordes KR, Sheehy NT, White MP, Berry EC, Morton SU, Muth AN et al. miR-145 and miR-143 regulate smooth muscle cell fate and plasticity. Nature 2009;460:705–710.
- Creemers EE, Tijsen AJ, Pinto YM. Circulating microRNAs: novel biomarkers and extracellular communicators in cardiovascular disease? Circ Res 2012;110:483–495.
- da Costa Martins PA, Salic K, Gladka MM, Armand AS, Leptidis S, el Azzouzi H et al. MicroRNA-199b targets the nuclear kinase Dyrk1a in an auto-amplification loop promoting calcineurin/NFAT signalling. Nat Cell Biol 2010;12:1220–1227.
- Danhier F, Ansorena E, Silva JM, Coco R, Le Breton A, Préat V. PLGA-based nanoparticles: an overview of biomedical applications. J Control Release 2012;161:505–522.
- Dentelli P, Rosso A, Orso F, Olgasi C, Taverna D, Brizzi MF. microRNA-222 controls neovascularization by regulating signal transducer and activator of transcription 5A expression. Arterioscler Thromb Vasc Biol 2010;30:1562–1568.
- Deregibus MC, Cantaluppi V, Calogero R, Lo Iacono M, Tetta C, Biancone L et al. Endothelial progenitor cell derived microvesicles activate an angiogenic program in endothelial cells by a horizontal transfer of mRNA. Blood 2007;110:2440–2448.
- Dong DL, Chen C, Huo R, Wang N, Li Z, Tu YJ et al. Reciprocal repression between microRNA-133 and calcineurin regulates cardiac hypertrophy: a novel mechanism for progressive cardiac hypertrophy. Hypertension 2010;55:946–952.
- Dorn GW 2nd. MicroRNAs: redefining mechanisms in cardiac disease. J Cardiovasc Pharmacol 2010;56:589–595.
- Duisters RF, Tijsen AJ, Schroen B, Leenders JJ, Lentink V, van der Made I et al. miR-133 and miR-30 regulate connective tissue growth factor: implications for a role of microRNAs in myocardial matrix remodeling. Circ Res 2009;104:170–178, 6p following 178.
- Ebert MS, Neilson JR, Sharp PA. MicroRNA sponges: competitive inhibitors of small RNAs in mammalian cells. Nat Methods 2007;4:721–726.
- Elmén J, Lindow M, Schütz S, Lawrence M, Petri A, Obad S et al. LNA-mediated microRNA silencing in non-human primates. Nature 2008;452:896–899.
- Esau C, Davis S, Murray SF, Yu XX, Pandey SK, Pear M et al. miR-122 regulation of lipid metabolism revealed by in vivo antisense targeting. Cell Metab 2006;3:87–98.
- Fang Y, Shi C, Manduchi E, Civelek M, Davies PF. MicroRNA-10a regulation of proinflammatory phenotype in athero-susceptible endothelium in vivo and in vitro. Proc Natl Acad Sci USA 2010;107:13450–13455.
- Fang J, Song XW, Tian J, Chen HY, Li DF, Wang JF et al. Overexpression of microRNA-378 attenuates ischemia-induced apoptosis by inhibiting caspase-3 expression in cardiac myocytes. Apoptosis 2012;17:410–423.
- Farokhzad OC, Langer R. Nanomedicine: developing smarter therapeutic and diagnostic modalities. Adv Drug Deliv Rev 2006;58:1456–1459.
- Fichtlscherer S, De Rosa S, Fox H, Schwietz T, Fischer A, Liebetrau C et al. Circulating microRNAs in patients with coronary artery disease. Circ Res 2010;107:677–684.
- Fichtlscherer S, Zeiher AM, Dimmeler S. Circulating microRNAs: biomarkers or mediators of cardiovascular diseases? Arterioscler Thromb Vasc Biol 2011;31:2383–2390.
- Fiedler J, Jazbutyte V, Kirchmaier BC, Gupta SK, Lorenzen J, Hartmann D et al. MicroRNA-24 regulates vascularity after myocardial infarction. Circulation 2011;124:720–730.
- Fish JE, Santoro MM, Morton SU, Yu S, Yeh RF, Wythe JD et al. miR-126 regulates angiogenic signaling and vascular integrity. Dev Cell 2008;15:272–284.
- Geisler A, Jungmann A, Kurreck J, Poller W, Katus HA, Vetter R et al. microRNA122-regulated transgene expression increases specificity of cardiac gene transfer upon intravenous delivery of AAV9 vectors. Gene Ther 2011;18:199–209.
- Glass C, Singla DK. MicroRNA-1 transfected embryonic stem cells enhance cardiac myocyte differentiation and inhibit apoptosis by modulating the PTEN/Akt pathway in the infarcted heart. Am J Physiol Heart Circ Physiol 2011;301:H2038–H2049.
- Grieger JC, Samulski RJ. Adeno-associated virus vectorology, manufacturing, and clinical applications. Meth Enzymol 2012;507:229–254.
- Grünweller A, Hartmann RK. Locked nucleic acid oligonucleotides: the next generation of antisense agents? BioDrugs 2007;21:235–243.
- Günther M, Lipka J, Malek A, Gutsch D, Kreyling W, Aigner A. Polyethylenimines for RNAi-mediated gene targeting in vivo and siRNA delivery to the lung. Eur J Pharm Biopharm 2011;77:438–449.
- Gupta S, Young D, Maitra RK, Gupta A, Popovic ZB, Yong SL et al. Prevention of cardiac hypertrophy and heart failure by silencing of NF-κB. J Mol Biol 2008;375:637–649.
- Gupta S, Maitra R, Young D, Gupta A, Sen S. Silencing the myotrophin gene by RNA interference leads to the regression of cardiac hypertrophy. Am J Physiol Heart Circ Physiol 2009;297:H627–H636.
- Gwinn MR, Vallyathan V. Nanoparticles: health effects–pros and cons. Environ Health Perspect 2006;114:1818–1825.
- Ibrahim AF, Weirauch U, Thomas M, Grünweller A, Hartmann RK, Aigner A. MicroRNA replacement therapy for miR-145 and miR-33a is efficacious in a model of colon carcinoma. Cancer Res 2011;71:5214–5224.
- Ikeda S, He A, Kong SW, Lu J, Bejar R, Bodyak N et al. MicroRNA-1 negatively regulates expression of the hypertrophy-associated calmodulin and Mef2a genes. Mol Cell Biol 2009;29:2193–2204.
- Haraguchi T, Ozaki Y, Iba H. Vectors expressing efficient RNA decoys achieve the long-term suppression of specific microRNA activity in mammalian cells. Nucleic Acids Res 2009;37:e43.
- Haraguchi T, Nakano H, Tagawa T, Ohki T, Ueno Y, Yoshida T et al. A potent 2′-O-methylated RNA-based microRNA inhibitor with unique secondary structures. Nucleic Acids Res 2012;40:e58.
- Hergenreider E, Heydt S, Tréguer K, Boettger T, Horrevoets AJ, Zeiher AM et al. Atheroprotective communication between endothelial cells and smooth muscle cells through miRNAs. Nat Cell Biol 2012;14:249–256.
- Hong S, Kim KY, Wook HJ, Park SY, Lee KD, Lee, DY, Lee H. Attenuation of the in vivo toxicity of biomaterials by polydopamine surface modification. Biomaterials 2011;32:2241–2247.
- Horie T, Ono K, Nishi H, Iwanaga Y, Nagao K, Kinoshita M et al. MicroRNA-133 regulates the expression of GLUT4 by targeting KLF15 and is involved in metabolic control in cardiac myocytes. Biochem Biophys Res Commun 2009;389:315–320.
- Hu S, Huang M, Nguyen PK, Gong Y, Li Z, Jia F et al. Novel microRNA prosurvival cocktail for improving engraftment and function of cardiac progenitor cell transplantation. Circulation 2011;124:S27–S34.
- Huang RS, Hu GQ, Lin B, Lin ZY, Sun CC. MicroRNA-155 silencing enhances inflammatory response and lipid uptake in oxidized low-density lipoprotein-stimulated human THP-1 macrophages. J Investig Med 2010;58:961–967.
- Jentzsch C, Leierseder S, Loyer X, Flohrschütz I, Sassi Y, Hartmann D et al. A phenotypic screen to identify hypertrophy-modulating microRNAs in primary cardiomyocytes. J Mol Cell Cardiol 2012;52:13–20.
- Ji R, Cheng Y, Yue J, Yang J, Liu X, Chen H et al. MicroRNA expression signature and antisense-mediated depletion reveal an essential role of MicroRNA in vascular neointimal lesion formation. Circ Res 2007;100:1579–1588.
- Kim HI, Ishihara K, Lee S, Seo JH, Kim HY, Suh D et al. Tissue response to poly(L-lactic acid)-based blend with phospholipid polymer for biodegradable cardiovascular stents. Biomaterials 2011;32:2241–2247.
- Kim HW, Choi YH, Kang SM, Ku JY, Ahn JH, Kim JM et al. Malignant inflammatory myofibroblastic tumor of the bladder with rapid progression. Korean J Urol 2012;53:657–661.
- Knuefermann P, Baumgarten G, Koch A, Schwederski M, Velten M, Ehrentraut H et al. CpG oligonucleotide activates Toll-like receptor 9 and causes lung inflammation in vivo. Respir Res 2007;8:72.
- Krützfeldt J, Rajewsky N, Braich R, Rajeev KG, Tuschl T, Manoharan M et al. Silencing of microRNAs in vivo with ‘antagomirs’. Nature 2005;438:685–689.
- Lai EC. Micro RNAs are complementary to 3′ UTR sequence motifs that mediate negative post-transcriptional regulation. Nat Genet 2002;30:363–364.
- Lanford RE, Hildebrandt-Eriksen ES, Petri A, Persson R, Lindow M, Munk ME et al. Therapeutic silencing of microRNA-122 in primates with chronic hepatitis C virus infection. Science 2010;327:198–201.
- Leroyer AS, Isobe H, Lesèche G, Castier Y, Wassef M, Mallat Z et al. Cellular origins and thrombogenic activity of microparticles isolated from human atherosclerotic plaques. J Am Coll Cardiol 2007;49:772–777.
- Liang GF, Zhu YL, Sun B, Hu FH, Tian T, Li SC et al. PLGA-based gene delivering nanoparticle enhance suppression effect of miRNA in HePG2 cells. Nanoscale Res Lett 2011;6:447.
- Love KT, Mahon KP, Levins CG, Whitehead KA, Querbes W, Dorkin JR et al. Lipid-like materials for low-dose, in vivo gene silencing. Proc Natl Acad Sci USA 2010;107:1864–1869.
- Liu X, Cheng Y, Zhang S, Lin Y, Yang J, Zhang C. A necessary role of miR-221 and miR-222 in vascular smooth muscle cell proliferation and neointimal hyperplasia. Circ Res 2009;104:476–487.
- Liu J, van Mil A, Vrijsen K, Zhao J, Gao L, Metz CH et al. MicroRNA-155 prevents necrotic cell death in human cardiomyocyte progenitor cells via targeting RIP1. J Cell Mol Med 2011;15:1474–1482.
- Loo YM, Gale M Jr. Immune signaling by RIG-I-like receptors. Immunity 2011;34:680–692.
- Lu Y, Zhang Y, Wang N, Pan Z, Gao X, Zhang F et al. MicroRNA-328 contributes to adverse electrical remodeling in atrial fibrillation. Circulation 2010;122:2378–2387.
- Manilla P, Rebello T, Afable C, Lu X, Slepushkin V, Humeau LM et al. Regulatory considerations for novel gene therapy products: a review of the process leading to the first clinical lentiviral vector. Hum Gene Ther 2005;16:17–25.
- Marik J, Tartis MS, Zhang H, Fung JY, Kheirolomoom A, Sutcliffe JL et al. Long-circulating liposomes radiolabeled with [18F]fluorodipalmitin ([18F]FDP). Nucl Med Biol 2007;34:165–171.
- Mendrick DL. Transcriptional profiling to identify biomarkers of disease and drug response. Pharmacogenomics 2011;12:235–249.
- Najafi-Shoushtari SH, Kristo F, Li Y, Shioda T, Cohen DE, Gerszten RE et al. MicroRNA-33 and the SREBP host genes cooperate to control cholesterol homeostasis. Science 2010;328:1566–1569.
- Patrick DM, Montgomery RL, Qi X, Obad S, Kauppinen S, Hill JA et al. Stress-dependent cardiac remodeling occurs in the absence of microRNA-21 in mice. J Clin Invest 2010;120:3912–3916.
- O’Riordan CR, Song A. PEGylated adenovirus for targeted gene therapy. Methods Mol Biol 2008;434:133–160.
- Porrello ER, Johnson BA, Aurora AB, Simpson E, Nam YJ, Matkovich SJ et al. MiR-15 family regulates postnatal mitotic arrest of cardiomyocytes. Circ Res 2011;109:670–679.
- Rayner KJ, Suárez Y, Dávalos A, Parathath S, Fitzgerald ML, Tamehiro N et al. MiR-33 contributes to the regulation of cholesterol homeostasis. Science 2010;328:1570–1573.
- Rayner KJ, Esau CC, Hussain FN, McDaniel AL, Marshall SM, van Gils JM et al. Inhibition of miR-33a/b in non-human primates raises plasma HDL and lowers VLDL triglycerides. Nature 2011a;478:404–407.
- Rayner KJ, Sheedy FJ, Esau CC, Hussain FN, Temel RE, Parathath S et al. Antagonism of miR-33 in mice promotes reverse cholesterol transport and regression of atherosclerosis. J Clin Invest 2011b;121:2921–2931.
- Ramirez CM, Dávalos A, Goedeke L, Salerno AG, Warrier N, Cirera-Salinas D et al. MicroRNA-758 regulates cholesterol efflux through posttranscriptional repression of ATP-binding cassette transporter A1. Arterioscler Thromb Vasc Biol 2011;31:2707–2714.
- Ranghino A, Cantaluppi V, Grange C, Vitillo L, Fop F, Biancone L et al. Endothelial progenitor cell-derived microvesicles improve neovascularization in a murine model of hindlimb ischemia. Int J Immunopathol Pharmacol 2012;25:75–85.
- Ren XP, Wu J, Wang X, Sartor MA, Qian J, Jones K et al. MicroRNA-320 is involved in the regulation of cardiac ischemia/reperfusion injury by targeting heat-shock protein 20. Circulation 2009;119:2357–2366.
- Roy S, Khanna S, Hussain SR, Biswas S, Azad A, Rink C et al. MicroRNA expression in response to murine myocardial infarction: miR-21 regulates fibroblast metalloprotease-2 via phosphatase and tensin homologue. Cardiovasc Res 2009;82:21–29.
- Sacco J, Adeli K. MicroRNAs: emerging roles in lipid and lipoprotein metabolism. Curr Opin Lipidol 2012;23:220–225.
- Salic K, De Windt LJ. MicroRNAs as biomarkers for myocardial infarction. Curr Atheroscler Rep 2012;14:193–200.
- Sayed D, Rane S, Lypowy J, He M, Chen IY, Vashistha H et al. MicroRNA-21 targets Sprouty2 and promotes cellular outgrowths. Mol Biol Cell 2008;19:3272–3282.
- SCENIHR. (2007). The scientific aspects of the existing and proposed definitions relating to products of nanoscience and nanotechnologies. 2007. Available at http://ec.europa.eu/health/ph_risk/committees/04_scenihr/docs/scenihr_o_012.pdf
- Semple SC, Akinc A, Chen J, Sandhu AP, Mui BL, Cho CK et al. Rational design of cationic lipids for siRNA delivery. Nat Biotechnol 2010;28:172–176.
- Singha K, Namgung R, Kim WJ. Polymers in small-interfering RNA delivery. Nucleic Acid Ther 2011;21:133–147.
- Shan H, Zhang Y, Lu Y, Zhang Y, Pan Z, Cai B et al. Downregulation of miR-133 and miR-590 contributes to nicotine-induced atrial remodelling in canines. Cardiovasc Res 2009;83:465–472.
- Shan ZX, Lin QX, Deng CY, Zhu JN, Mai LP, Liu JL et al. miR-1/miR-206 regulate Hsp60 expression contributing to glucose-mediated apoptosis in cardiomyocytes. FEBS Lett 2010;584:3592–3600.
- Tang Y, Zheng J, Sun Y, Wu Z, Liu Z, Huang G. MicroRNA-1 regulates cardiomyocyte apoptosis by targeting Bcl-2. Int Heart J 2009;50:377–387.
- Tano N, Kim HW, Ashraf M. microRNA-150 regulates mobilization and migration of bone marrow-derived mononuclear cells by targeting Cxcr4. PLoS ONE 2011;6:e23114.
- Tasaka S, Kamata H, Miyamoto K, Nakano Y, Shinoda H, Kimizuka Y et al. Intratracheal synthetic CpG oligodeoxynucleotide causes acute lung injury with systemic inflammatory response. Respir Res 2009;10:84.
- Tatsuguchi M, Seok HY, Callis TE, Thomson JM, Chen JF, Newman M et al. Expression of microRNAs is dynamically regulated during cardiomyocyte hypertrophy. J Mol Cell Cardiol 2007;42:1137–1141.
- Teeguarden JG, Hinderliter PM, Orr G, Thrall BD, Pounds JG. Particokinetics in vitro: dosimetry considerations for in vitro nanoparticle toxicity assessments. Toxicol Sci 2007;95:300–312.
- Terentyev D, Belevych AE, Terentyeva R, Martin MM, Malana GE, Kuhn DE et al. miR-1 overexpression enhances Ca(2+) release and promotes cardiac arrhythmogenesis by targeting PP2A regulatory subunit B56α and causing CaMKII-dependent hyperphosphorylation of RyR2. Circ Res 2009;104:514–521.
- Thum T, Gross C, Fiedler J, Fischer T, Kissler S, Bussen M et al. MicroRNA-21 contributes to myocardial disease by stimulating MAP kinase signalling in fibroblasts. Nature 2008;456:980–984.
- Thum T, Chau N, Bhat B, Gupta SK, Linsley PS, Bauersachs J et al. Comparison of different miR-21 inhibitor chemistries in a cardiac disease model. J Clin Invest 2011;121:461–462; author reply 462.
- van Rooij E, Sutherland LB, Qi X, Richardson JA, Hill J, Olson EN. Control of stress-dependent cardiac growth and gene expression by a microRNA. Science 2007;316:575–579.
- van Rooij E, Sutherland LB, Thatcher JE, DiMaio JM, Naseem RH, Marshall WS et al. Dysregulation of microRNAs after myocardial infarction reveals a role of miR-29 in cardiac fibrosis. Proc Natl Acad Sci USA 2008;105:13027–13032.
- van Solingen C, Seghers L, Bijkerk R, Duijs JM, Roeten MK, van Oeveren-Rietdijk AM et al. Antagomir-mediated silencing of endothelial cell specific microRNA-126 impairs ischemia-induced angiogenesis. J Cell Mol Med 2009;13:1577–1585.
- Vasir JK, Labhasetwar V. Biodegradable nanoparticles for cytosolic delivery of therapeutics. Adv Drug Deliv Rev 2007;59:718–728.
- Vetrini F, Ng P. Gene therapy with helper-dependent adenoviral vectors: current advances and future perspectives. Viruses 2010;2:1886–1917.
- Vickers KC, Palmisano BT, Shoucri BM, Shamburek RD, Remaley AT. MicroRNAs are transported in plasma and delivered to recipient cells by high-density lipoproteins. Nat Cell Biol 2011;13:423–433.
- Wang S, Aurora AB, Johnson BA, Qi X, McAnally J, Hill JA et al. The endothelial-specific microRNA miR-126 governs vascular integrity and angiogenesis. Dev Cell 2008;15:261–271.
- Weber M, Baker MB, Moore JP, Searles CD. MiR-21 is induced in endothelial cells by shear stress and modulates apoptosis and eNOS activity. Biochem Biophys Res Commun 2010;393:643–648.
- Wickline SA, Neubauer AM, Winter P, Caruthers S, Lanza G. Applications of nanotechnology to atherosclerosis, thrombosis, and vascular biology. Arterioscler Thromb Vasc Biol 2006;26:435–441.
- Xiao C, Calado DP, Galler G, Thai TH, Patterson HC, Wang J et al. MiR-150 controls B cell differentiation by targeting the transcription factor c-Myb. Cell 2007a;131:146–159.
- Xiao J, Luo X, Lin H, Zhang Y, Lu Y, Wang N, Zhang Y, Yang B, Wang Z. MicroRNA miR-133 represses HERG K+ channel expression contributing to QT prolongation in diabetic hearts. J Biol Chem 2007b;282:12363−12367.
- Xie J, Xie Q, Zhang H, Ameres SL, Hung JH, Su Q et al. MicroRNA-regulated, systemically delivered rAAV9: a step closer to CNS-restricted transgene expression. Mol Ther 2011;19:526–535.
- Xie J, Ameres SL, Friedline R, Hung JH, Zhang Y, Xie Q et al. Long-term, efficient inhibition of microRNA function in mice using rAAV vectors. Nat Methods 2012;9:403–409.
- Xu C, Lu Y, Pan Z, Chu W, Luo X, Lin H et al. The muscle-specific microRNAs miR-1 and miR-133 produce opposing effects on apoptosis by targeting HSP60, HSP70 and caspase-9 in cardiomyocytes. J Cell Sci 2007;120:3045–3052.
- Yang B, Lin H, Xiao J, Lu Y, Luo X, Li B et al. The muscle-specific microRNA miR-1 regulates cardiac arrhythmogenic potential by targeting GJA1 and KCNJ2. Nat Med 2007;13:486–491.
- Yang K, He YS, Wang XQ, Lu L, Chen QJ, Liu J et al. MiR-146a inhibits oxidized low-density lipoprotein-induced lipid accumulation and inflammatory response via targeting toll-like receptor 4. FEBS Lett 2011;585:854–860.
- Yang Y, Ago T, Zhai P, Abdellatif M, Sadoshima J. Thioredoxin 1 negatively regulates angiotensin II-induced cardiac hypertrophy through upregulation of miR-98/let-7. Circ Res 2011;108:305–313.
- Yao XL, Nakagawa S, Gao JQ. Current targeting strategies for adenovirus vectors in cancer gene therapy. Curr Cancer Drug Targets 2011;11:810–825.
- Zampetaki A, Kiechl S, Drozdov I, Willeit P, Mayr U, Prokopi M et al. Plasma microRNA profiling reveals loss of endothelial miR-126 and other microRNAs in type 2 diabetes. Circ Res 2010;107:810–817.
- Zampetaki A, Mayr M. MicroRNAs in vascular and metabolic disease. Circ Res 2012;110:508–522.
- Zernecke A, Schober A, Bot I, von Hundelshausen P, Liehn EA, Möpps B et al. SDF-1α/CXCR4 axis is instrumental in neointimal hyperplasia and recruitment of smooth muscle progenitor cells. Circ Res 2005;96:784–791.
- Zernecke A, Bidzhekov K, Noels H, Shagdarsuren E, Gan L, Denecke B et al. Delivery of microRNA-126 by apoptotic bodies induces CXCL12-dependent vascular protection. Sci Signal 2009;2:ra81.
- Zernecke A, Bot I, Djalali-Talab Y, Shagdarsuren E, Bidzhekov K, Meiler S et al. Protective role of CXC receptor 4/CXC ligand 12 unveils the importance of neutrophils in atherosclerosis. Circ Res 2008;102:209–217.
- Zhang Y, Liu D, Chen X, Li J, Li L, Bian Z et al. Secreted monocytic miR-150 enhances targeted endothelial cell migration. Mol Cell 2010;39:133–144.
- Zhao Y, Ransom JF, Li A, Vedantham V, von Drehle M, Muth AN et al. Dysregulation of cardiogenesis, cardiac conduction, and cell cycle in mice lacking miRNA-1-2. Cell 2007;129:303–317.
- Zidar N, Boštjancic E, Glavac D, Stajer D. MicroRNAs, innate immunity and ventricular rupture in human myocardial infarction. Dis Markers 2011;31:259–265.